GIRK Channel Trafficking: Different Paths for Different Family Members
Ion channel proteins act as gateways to the excitation of cells; therefore, their activation is a highly regulated process. Research has focused mainly on the signaling mechanisms (e.g., phosphorylation, protein–protein interactions, and ligand binding) that regulate the function of these integral membrane proteins. These regulatory mechanisms control the activity of proteins that have already been targeted to the membrane. An area of research only now becoming appreciated is the process by which the ion-channel proteins are translocated to the cell surface (1).
Several groups have reported specific trafficking signals embedded within ion channels, that is, amino acid sequence motifs that are crucial for channel membrane–targeting and coassembly (2). Studies on inwardly rectifying K+ channels (Kir), a family of potassium channels with intracellular N and C termini and two transmembrane domains flanking a P region (which controls K+ selectivity), have provided some clues to the complexity with which ion channels are processed and targeted to the membrane (3–6). Members of this family (Kir1–7) form tetramers. Some of these channels can function as homomers (e.g., Kir2.1) and some as heteromers (e.g., Kir3.1–Kir3.4) (7). One group of these channels, Kir6, can multimerize with the sulfonylurea receptor (SUR), a member of the ATP-binding-cassette family, to form ATP-sensitive K+ channels.
One class of inward rectifiers, Kir3, also termed G protein–regulated inwardly rectifying K+ channels (GIRK), forms channels that are directly activated by the βγ subunits of G proteins (8). These channels are abundant in atrial cells as well as neurons, and four different channel subunits have been cloned so far (Kir3.1–3.4). Kir3.1 does not form functional channels when expressed alone, due to defects in its P region and in membrane targeting (4, 9, 10). Overexpression of Kir3.1 and other Kir3s results in highly active channels that are indistinguishable from native channels (11). Although homomeric Kir3.2 and Kir3.4 channels have been found in native tissues (12), exogenously expressed homomers of these channels give very brief and poorly resolved open-time kinetics that are different from native channels (9).
Kir3.1 can be coprecipitated with all of the other Kir3 subunits (11, 13). Interestingly, Jelacic and colleagues showed that Kir3.2–Kir3.3 heteromers have some activity; however, these channels show reduced Gβγ sensitivity compared to other heteromers containing Kir3.1 subunits (14). These researchers also coimmunoprecipitated Kir3.2 and Kir3.3 from brain, indicating they interact with each other in native tissues.
Ma and colleagues used immunofluorescence, surface labeling, western-blot analysis, and whole-cell current measurements to characterize complex trafficking patterns of Kir3 channels (15). They show that Kir3.1 and Kir3.3 reside in the endoplasmic reticulum (ER). Unlike Kir6.2 (5), no specific ER retention signal has been identified for either Kir3.1 or Kir3.3. However, just as Kir6.2 can be brought to the cell surface by coexpression of SUR, Kir3.1, which can associate with microtubules (16), localizes to the surface upon association with Kir3.2 or Kir3.4. Although attachment of an ER transport signal facilitates Kir3.1 exit from the ER, Kir3.1 still fails to reach the cell surface in the absence of Kir3.2 or Kir3.4, suggesting additional control mechanisms are necessary.
On the other hand, Kir3.2 and Kir3.4, both of which can function as homomers, contain ER export signals and reach the surface efficiently (16). ER export signals have also been identified in Kir2.1 and Kir1.1 (6, 17). Ma and colleagues now show that a region in the N terminus of Kir3.4 is involved in the ER export of channels because a deletion in this region leads to channel accumulation in the ER (15). Once Kir3.4 channels exit the ER, the majority of them localize to the plasma membrane, although some channel proteins can also be found in vesicles and juxtanuclear regions. Deletions in the Kir3.4 C terminus that remove a cluster of acidic residues lead to the accumulation of a large number of channels in vesicles, resulting in a reduction in surface expression and total number of Kir3.4-containing channels. It may be important to note that the C-terminal region in this Kir3.4 diverges from the C-terminal sequence in another human Kir3.4 clone.
In Kir3.2, a similar cluster of acidic residues in the C terminus contains a consensus Ser-Thr phosphorylation site. Mutation of Thr to Ala at this site leads to reduced surface expression of Kir3.2; however, mutation of Thr to Asp, mimicking the phosphorylated state of the channel, leads to enhanced surface expression (15). These results suggest that the surface expression of Kir3.2 channel can be dynamically regulated by the phosphorylation state of the channel, implying that this channel can be recruited to the surface in response to a stimulus that leads to its phosphorylation. Also, a “VL motif”—similar to the well-characterized LL motif that controls both endocytic and secretory pathways (18) in the N terminus of Kir3.2—is critical for targeting the channel to the endosomes. There are four different splice variants of Kir3.2 that differ in their sequences mainly at the extreme N- and C-termini of the channel. Kir3.2B, which is truncated at the C terminus and lacks the acidic cluster, shows reduced surface expression. Kir3.2C, which has a Postsynaptic Density-95 (PSD-95)/Discs Large/Zona Occludens-1- (PDZ)-binding domain shows enhanced surface expression. Kir3.2D lacks several residues in the N terminus including the essential VL motif, leading to increased levels of this splice variant on the surface. Both Kir3.2 and Kir3.4 can recruit Kir3.1, greatly increasing its surface expression (4, 16, 19, 20) and modestly increasing its total expression (16).
A most intriguing finding by Ma and colleagues is that Kir3.3 not only lacks an ER export signal, despite its high similarity to Kir3.2 and Kir3.4, but in addition, Kir3.3 contains a lysosomal targeting signal (15). Coexpression of Kir3.3 with Kir3.1 and Kir3.2 leads to reduced expression of Kir3.1 at the cell surface. This suggests that one role for Kir3.3 may be to inhibit the number of Kir3.1-containing channels at the surface. Figure 1⇓ summarizes some of the trafficking patterns of Kir3 channels.
Overall, the complex trafficking of Kir3 channels in the cell assures that only properly assembled channels reach the surface. However, several questions remain. Although homomeric Kir3.2 and Kir3.4 exhibit reduced activity, they are expressed efficiently on the cell surface. Do they form functional homomers on the surface? Is Kir3.3 part of the “functional” channel complex in the native tissue or does it rather act as a chaperone ensuring proper assembly and numbers of Kir3 channels on the surface? One untested, possible scenario for regulating the density of functional Kir3.1–Kir3.2 (or Kir3.1–Kir3.4) channels on cell surface is to control the amount of Kir3.3 expressed, and therefore, the number of Kir3.1–3.2 channels on the plasma membrane.
Recent data show that Kir3.3 knockout mice show reduced numbers of Kir3.1 subunits, especially those that are fully glycosylated (21). If Kir3.3 expression leads to the reduced surface expression of Kir3.1, as suggested by Ma and colleagues (15), one would have expected enhanced surface expression of fully glycosylated Kir3.1 channels in Kir3.3 knockout mice. Of course this discrepancy may simply reflect that in a knockout animal, the protein expression levels might be controlled by other compensatory mechanisms. Also, coassembly of Kir3.2 and Kir3.3 has been observed in both native and heterologous expression systems (14). Does the presence of Kir3.3 lead to a reduction of Kir3.2 surface expression? Neurons from the locus ceruleus of Kir3.3−/− mice show depolarized resting potentials, similar to the phenotype observed in the same neurons from Kir3.2−/− mice. This suggests that both these subunits play important functional roles in maintaining the membrane potential in these neurons. What remains unclear is whether this is due to the direct functional consequences of knocking out Kir3.3 or a different mechanism.
Comparison of the sequences of Kir3.3 and other Kir3 family members suggests that other differences might have functional consequences as well. For example, Glu35 in Kir3.3 is replaced by His64 (the equivalent position) in Kir3.4, and His64 interacts with Gβγ (22). Whereas Kir3.3 has Asp165, other inward rectifiers contain a lysine residue that is involved in interacting with phosphoinositide-4,5-bisphosphate (PIP2) (23). These functional differences, along with the unique trafficking characteristics of Kir3.3, suggest a special regulatory role for this channel subunit when it is part of the G protein–sensitive K+ channel complex.
Overall, the recent findings point to a complex regulation of GIRK channel expression on the cell surface. However, the functional implications of such complex regulation remain to be elucidated.
Kir3/GIRK channel trafficking. A. Diagram showing the topology of Kir channels and the location of the domains identified by Ma et al. (15) that play important roles in Kir3 channel trafficking. B. Schematic representation of the localization of different channel subunits (Kir3.1—Kir3.4). Channels and cell compartments are color coded. Combinations of only two subunits (rather than four) are shown for purposes of illustration. Kir, inwardly rectifying K+ channels; GIRK, G protein-regulated inwardly rectifying channels; TM, transmembrane; P, P-region; TGN, trans Golgi network.
- © American Society for Pharmacology and Experimental Theraputics 2002
References
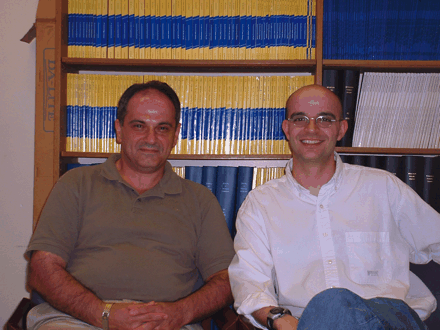
Diomedes E. Logothetis, PhD, (left) is Professor of Physiology and Biophysics at Mount Sinai School of Medicine of NYU. Please address correspondence to DEL. E-mail diomedes{at}inka.mssm.edu Tooraj Mirshahi, PhD, (right) is a postdoctoral fellow in the Logothetis lab at the Department of Physiology and Biophysics at Mount Sinai School of Medicine of NYU.