MOSAICISM OF THE RETINAL PIGMENT EPITHELIUM: seeing the small picture
Abstract
The retinal pigment epithelium (RPE) is a monolayer of cells that appear phenotypically regular, but which exhibit striking cell-cell variability in content of melanin and lipofuscin granules, and in expression of many proteins. This naturally occurring cell heterogeneity likely arises by normal mechanisms regulating gene expression during development and postnatal aging. The consequence is a tissue in which individual cells may differ in their ability to support adjacent photoreceptors, and which may respond differentially to oxidative stress and other environmental influences that contribute to cell dysfunction during aging. The inherent variability of RPE cells is probably one factor contributing to the characteristically patchy pattern of retinal diseases like age-related macular degeneration.
Introduction
The retinal pigment epithelium (RPE) is a monolayer of cuboidal cells located subjacent to the neural retina (1). As expected for an epithelium, RPE cells have apical microvilli and parallel lateral membranes joined near the cell apices by junctional complexes of occluding and adherens junctions. RPE cells receive their name from the melanin pigment granules (melanosomes) located in the apical cytoplasm. RPE cells also contain prominent granules of lipofuscin, autofluorescent materials that increase in amount throughout life in this largely non-mitotic tissue. Basal membranes of the RPE are in contact with Bruch’s membrane, a multilayered matrix that separates the RPE from the underlying vascular choroid. Unlike most monolayer epithelial cells, which have an apical domain that faces a lumen, RPE cell apices are associated with the outer segments of retinal photoreceptor cells (rods and cones).
The close structural association of RPE cells with the outer retina provides a clue to the major functions of this tissue, which are to support the survival and normal functioning of photoreceptors. The RPE layer acts as the outer blood–retinal barrier and operates as a transporting epithelium that moves nutrients, waste products, ions, and gases between the underlying choroidal blood vessels and overlying photoreceptors. The RPE is essential for photoreceptor renewal, an ongoing process in which outer segment membrane discs are shed, phagocytized by the RPE, and digested. Membrane materials are then shuttled by the RPE back to photoreceptors. The RPE participates in the visual cycle in which retinoids, used by photoreceptors to synthesize visual pigments, are moved back and forth between the RPE and the neural retina. RPE cells also produce growth and trophic factors necessary for photoreceptor survival. The interactions between the tissues are so critical that damage to the RPE, or separation of the RPE from photoreceptors as occurs with retinal detachment, can lead to photoreceptor degeneration.
Each RPE cell interacts with approximately forty-five photoreceptors, and individual photoreceptor cells are highly dependent for support on the individual RPE cell that serves them. One might expect that photoreceptors would be equivalently supported by the adjacent RPE since a superficial examination of the RPE layer suggests that the cells are homogeneous, indeed strikingly so. When the retina is stripped to prepare an RPE flat mount so that cells can be seen in surface view, the RPE looks like a layer of regular, hexagonal, phenotypically similar cells (Figure 1⇓). This gross phenotypic similarity, however, belies marked cell–cell variability. In this review we focus on the intercellular heterogeneity of RPE cells, emphasizing differences among cells in protein expression. We describe how this tissue mosaicism may arise and speculate on the consequences of RPE cell variability for maintenance of the photoreceptors.
Whole mount of the human RPE monolayer stained with fluorescein phalloidin for actin to show similarity in cell shape but heterogeneity in granule content. Actin is organized in a circumferential microfilament bundle (green), which illustrates the regular hexagonal shape of the cells. Individual cells vary in granule content, with some cells having abundant brown pigment granules (large arrows) and others abundant yellow lipofuscin granules (small arrows). The specimen was prepared by lifting off the neural retina, then processing the RPE with underlying choroid for epifluorescence microscopy. Scale bar: 20 μm.
The idea is not new that the RPE, or any tissue for that matter, is a mosaic of differing cells. As mentioned below, all tissues in females are mosaics as a consequence of random X-chromosome inactivation during development. However, the type of mosaicism we discuss here is not so well recognized (or perhaps it is more easily forgotten). It is the mosaicism that may arise as a normal end stage of development from what might be called refinements in gene expression beyond those that determine tissue-level differentiation. Such intercellular variability might also originate from ongoing gene silencing within mature tissues in the adult.
Regardless of how RPE cell heterogeneity arises, it is reasonable to ask whether inherently different RPE cells might respond differently to the effects of aging. This question becomes particularly relevant because the incidence of aging eye diseases, especially age-related macular degeneration (AMD), is growing as life expectancies increase and more of the population attains the age when AMD becomes prevalent. AMD is a complex neurodegenerative disease involving the retina, RPE and choroid in the macular region, the spot in the posterior retina that is used for high acuity vision. The most common form of the disease is characterized by disturbances at the level of the RPE, and there is growing evidence to suggest that age-related RPE dysfunction is an underlying cause of AMD (2–4).
The age-related changes in the RPE and surrounding tissues that predispose the retina to degenerate are unknown, but oxidative stress is believed to play a role (2–5). The RPE is at high risk for oxidative damage due to its location in a highly oxygenated environment and its exposure to high levels of visible light, which can increase oxidative damage by reacting with endogenous photosensitizers, notably lipofuscin (6, 7). Additional oxidative stress to the RPE results from phagocytosis of photoreceptor outer segment membranes, which are rich in polyunsaturated fatty acids that may undergo peroxidation (8). RPE cells are normally well protected against oxidative damage by their complement of antioxidants and antioxidant enzymes (9) such as catalase, superoxide dismutase, glutathione peroxidase, vitamins E and C, and even melanin (10). But if the RPE layer is a mosaic of cells with differing gene/protein expression patterns, is it reasonable to consider that all cells, even all cells within the macula, will be equally affected by a lifetime of oxidative stress?
Topographic and Cellular Variability of the RPE
Topographic Heterogeneity
There is considerable interest in understanding why the macular region of the retina is predisposed to aging disease. Consequently, there have been many studies comparing features of the RPE from peripheral and posterior regions; the posterior region is where the macula is located in primate eyes or the analogous area centralis is found in eyes of many other animals. Morphometric analyses demonstrate topographical differences in RPE cell size, shape, packing density, and granule content (11–14). Many regional functional differences in the RPE have also been demonstrated. Using several species, posterior RPE cells have been shown to have lower in vitro growth potential (15), lower Na+/K+–ATPase pump site density (16), and lower activities of cytochrome oxidase (17) and γ-glutamyltranspeptidase (18). The posterior RPE also has lower activities of some lysosomal enzymes such as acid phosphatase, β-glucuronidase, and N-acetyl-β-glucuronidase (19), but higher activity of cathepsin D (19–21). It is likely that there are many other as yet unidentified differences between posterior and peripheral RPE cells. Such topographic variations are expected because the adjacent retina with which the RPE associates also has well recognized regional specializations. Cone-type photoreceptor frequency, for example, is much higher in the macula, which accounts for the macula’s role in high acuity and color vision, visual functions mediated by cones but not rods.
Cell–Cell Heterogeneity
In addition to differences in the RPE from different topographical regions of the eye, RPE cells within regions also vary, showing differences among individual cells or patches of cells. The field of RPE cells illustrated in Figure 1⇑ was selected to demonstrate the morphologic regularity of RPE cells in the same monolayer, but close scrutiny reveals that all cells in this field are not alike; there is, in fact, substantial cell-cell heterogeneity in a basic property of RPE cells, namely, granule content. Some cells have large numbers of melanosomes and few lipofuscin granules, whereas others have the reverse, and the variability among cells in granule number can be remarkable. When human RPE cells are explanted into culture and first begin to spread so that individual granules can be discriminated, autofluorescent lipofuscin granules range in number from 15 or so to hundreds per cell (22). Because the biogenesis of RPE granules is complex, there are many points of regulation that could determine granule frequency; the specific differences among RPE cells that contribute to differing granule abundance are not known. Lipofuscin arises from ongoing processes of outer segment phagocytosis and autophagy (23), so one could argue that lipofuscin granule number in any given cell could vary over short time intervals. However, lipofuscin content slowly increases in the RPE with age (11), so the large differences in granule number among individual cells likely represents a persistent difference among cells leading to differential accumulation. Similarly, RPE melanosomes are synthesized largely during development and show little turnover (24), so high or low melanosome number is likely to be an inherent property of each RPE cell throughout its lifetime. The variation in light-absorbing melanin among RPE cells is great enough to determine whether individual cells are killed or spared when the retina is treated with subclinical laser energies (25).
Aside from gross differences in granule content, the expression of many specific proteins can differ from cell to cell in the RPE. Bovine RPE whole mounts have been particularly useful for demonstrating heterogeneous protein expression among cells because bovine eyes are abundant and large, and cells in the tapetal region have few melanin (or lipofuscin) granules to obscure protein localization. In bovine RPE whole mounts, the intermediate filament protein vimentin has been localized to cells arranged in small clusters or rows (Figure 2⇓) (26). The adhesion protein E-cadherin distributes to cell–cell junctions in scattered patches of about twenty to thirty contiguous RPE cells (27). The membrane cytoskeletal linker protein ankyrin is abundant in contiguous clusters or rows of about ten to twenty RPE cells, which, interestingly, comprise a subset of cells in the patches with high levels of junctional E-cadherin (27). The Na+/K+–ATPase is found at very high levels in patterns of individual, pairs, or short rows of RPE cells (27) and the frequency of RPE cells with high Na+/K+–ATPase expression levels is lower in the posterior retina as compared to the periphery, thereby exemplifying a higher order of mosaicism. Several other enzymes also show pronounced cell–cell heterogeneity in bovine RPE, including dipeptidylpeptidase II and IV, aminopeptidase M, alkaline phosphatase, and γ-glutamyltranspeptidase (18); the cells that highly express these enzymes occur in patterns of irregular interconnected islands. In addition to cell–cell variability in steady-state protein levels, treatment of the bovine RPE layer in isolated eyes with the tyrosine phosphatase inhibitor orthovanadate produces heterogeneous patterns of phosphotyrosine staining (26). The staining, for example, shows discrete junctional enrichment only in some individual cells or small groups of cells.
Whole mount of the bovine RPE monolayer immunostained for the intermediate filament protein vimentin to illustrate a mosaic pattern of protein expression. Vimentin has a circumferential distribution in the peripheral cytoplasm (green) within a row-like subset of RPE cells. The tissue shown here is from the tapetal region of the cow eye which has relatively few melanosomes (brown granules), lipofuscin (yellow granules), and combined melanolipofuscin granules. Specimen was prepared as described in Figure 1. Scale bar: 20 μm.
Mosaicism in protein expression is not limited to bovine RPE, but has been demonstrated in other species as well. E-cadherin has a discrete patch-like distribution in human RPE (28), and vimentin varies among cells in the RPE layer of several species (29). Human RPE cells are heterogeneous in protein (and gene) expression for the antioxidant enzymes heme oxygenase-1 (HO-1) and catalase (30). Although the two-dimensional mosaic pattern of these enzymes was not investigated, gene and protein expression were analyzed, and both levels of expression demonstrated mosaicism.
It is difficult to know with certainty if protein expression differences seen among RPE cells in situ are transient, reflecting the moment in time when the tissue was processed, or are a persistent and inherent property of the cells. Frohlich and Klessen (18) argue for the latter because the cell–cell heterogeneity in enzyme levels they observed in adult bovine RPE was also found in the fetal tissue, and it persisted when RPE cells were propagated in culture.
Indeed, the issue of inherent differences among RPE cells comes down to whether a trait can be transmitted with fidelity to daughter cells in a propagating population. This issue can be addressed using cultured RPE, which readily grow in vitro from many species even though the tissue in the adult eye is post-mitotic. Unfortunately, although RPE cells can be propagated, they have not been successfully cloned. Clonal subpopulations produced from the same donor could be used to demonstrate heritable differences among cellular descendants arising from different individual progenitors. Because RPE clones are unavailable for analysis of cell-cell differences, other strategies have been used. Human RPE cells, for example, have been plated sparsely so that individual antecedent cells grow into localized colonies. These display several discrete cell phenotypes that vary in frequency in RPE cultures from different donors (26) (Figure 3⇓). The phenotypically distinct colonies appear to represent RPE cell subpopulations that differ inherently in determinants of cell shape. The shape variants remain unchanged as long as cultures remain undisturbed at confluency, and when cultures are re-plated sparsely they recur in a frequency typical for the donor. Partial separation of epithelioid and fusiform RPE subpopulations has been accomplished, and these “breed true” on repropagation, retaining their different morphologies (31). Cell shape is determined by a large number of structural and regulatory proteins, and the specific differences among RPE cells that produce the phenotypic variants are unknown. Although environmental factors may also affect shape, these are controlled for in cell culture. Similar phenotypic variants have been found in RPE cultures from other species where the morphotypes were shown to exhibit different functional properties as well (32, 33).
Postconfluent culture of human RPE cells immunostained with antibodies to cytokeratins to illustrate phenotypic variants in the same culture. Adjacent colonies of highly epithelioid (ep) and highly fusiform (fus) RPE cells are shown. Epifluorescence microscopy; scale bar: 100 μm.
Aside from heterogeneity in the complex property of cell phenotype, RPE cells in culture also display heterogeneity in expression of specific proteins. In addition to the enzymes analyzed by others (18), we have found prominent intercellular differences in Na+/K+–ATPase (34) and E-cadherin (28) in postconfluent cultures of human RPE, similar to the differences that were demonstrated in situ within bovine or human eyes. E-cadherin positive patches in culture differ in frequency among donors, remain stable over time within undisturbed confluent cultures, and recur on propagation in about the same frequency from passage to passage. Collectively these observations indicate that the RPE monolayer is a mosaic of structurally and functionally heterogeneous cells that intermingle in various patterns both in situ and in vitro.
Mechanisms for the Development of Topographic Heterogeneity and Cellular Mosaicism
Topographic Heterogeneity and RPE Development
Heterogeneity of epithelial tissues is an expected outcome of normal development and organogenesis (35). For the RPE, the differences between posterior pole and peripheral regions arise from how this tissue develops in tandem with the adjacent sensory retina. Detailed investigations of RPE development come largely from studies of the mouse (36, 37), the rhesus macaque monkey (38, 39) and the zebrafish (40). The final topography of the RPE varies for organisms without posterior retinal specializations (i.e., rats and mice) or with posterior cone-rich regions (i.e., the macula of primates or the area centralis of dogs, pigs, and cows). Regardless of topography, the outlines of RPE development remain the same. The tissue arises from the neuroectoderm-derived optic vesicle, which collapses to form the bilayered optic cup. The inner layer of cells gives rise to the sensory retina, and the outer layer gives rise to the RPE. The developing RPE undergoes a period cell proliferation, followed by growth quiescence and then differentiation. These stages of development proceed in a topographical pattern; cells at the posterior pole stop dividing first, followed by a radial entry of RPE cells into quiescence. Because posterior and peripheral RPE undergo differentiation at different times as well as in different locations, it is not surprising that there are differences within the tissue related to topography.
Cellular Mosaicism
Of greater interest to us here than topographical variation that arises through conventional organogenesis, however, is the cell–cell heterogeneity that is seen within the RPE. This heterogeneity appears to be an example of somatic cell mosaicism, a phenomenon in which tissues exhibit a patchwork of cells varying in phenotype or genotype (or both). Within each patch, neighboring cells are identical, but adjacent patches are not. The existence of cellular mosaics has been known for some time, and mosaicism is best identified with normal development and differentiation, as well as with X-linked genetic syndromes (41).
There are a remarkable number of ways in which tissue mosaics can arise, which include both genetic and epigenetic mechanisms. During development, a single cell becomes a multicellular organism through highly ordered events of division, migration, and differentiation. Any genetic event that occurs in one cell in a population, which is otherwise identical, will give rise to mosaicism in the adult tissues arising from this lineage. The number of tissues affected and the distribution and size of clonal populations within each tissue are results of the timing of the genetic alteration relative to the stage of tissue development.
Genetic Models of Cellular Mosaicism
Mosaicism in the pigment epithelium was first studied using pigmentary chimeras that were experimentally produced by mixing blastocysts from two lines of mice, one albino and one pigmented (36, 37, 42, 43, 44). Because the mixing occurred early in the development of the chimeric animals, most tissues (including the RPE) were mosaics due to the presence of daughter cells from each genotype. Because pigmentation is a cell autonomous marker, chimeras formed from pigmented and nonpigmented mouse strains have adult tissues in which clone size and position can be observed, providing insight into developmental processes like timing of clonal cell expansion and cell mixing. In mouse chimeras, singletons and doublets are the most frequently observed clone sizes, but patches of up to fifty cells do occur at lower frequency. The peripheral RPE has larger patches, which frequently (but not always) have a radial orientation.
The more common genetic causes for the formation of tissue mosaics occur after the blastula stage of development. These include mutations, reversions, and deletions, which may occur in a single cell and then be propagated into all its descendants (41). Mosaicism in the RPE of the pink-eyed mouse mutant is a good example of this phenomenon (42). In this animal, RPE cells are typically amelanotic, but during RPE development when cells are dividing, several independent reversions of the mutation occur, giving rise to clones of wild-type, pigmented cells. The result is an albino fundus with small, pigmented islands of RPE cells.
Epigenetic Models of Mosaicism
Epigenetics is broadly defined as the study of covalent modifications of the genome that are heritable but do not involve direct changes to the DNA sequence (45). The major forms of epigenetic modification are covalent modification of histones and methylation of cytosine in CpG islands of gene promoters, both of which can produce transcriptional silencing. These modifications can generate tissue-specific gene expression patterns during normal development. Further, they may theoretically produce differential gene expression patterns within a tissue if they occur during later stages of developmental tissue maturation, and the result then would be a tissue mosaic. It is reasonable to consider that the type of cell–cell heterogeneity that has been observed in the RPE and is described above arises at least partially from this mechanism.
X-inactivation is a form of epigenesis in which one of the two X chromosomes in females is inactivated during normal development by chromatin condensation, generating the Barr Body. This process is random with respect to whether the maternal or the paternal X chromosome is inactivated (45). The result is an embryo in which all tissues (including the RPE) become X chromosome mosaics, and X-inactivation can thus be exploited as a method to produce pigmentary mosaics in the RPE (42). X-inactivation and chimeric mosaics in the mouse RPE are essentially identical in terms of cell patch size and distribution. Which X-chromosome is inactivated has significance for X-linked diseases; the patchy appearance of fundus changes for X-linked retinal degenerations has been directly attributed to X-linked mosaic patterns (46, 47).
Epigenetic gene silencing may continue later in life, which would generate mosaic patterns of cells with different gene expression profiles within tissues as they age (48, 49). Examples of mosaicism resulting from age-related epigenetic gene silencing occur in early-onset Alzheimer’s disease (50) as well as in cancer susceptibility resulting from the silencing of tumor suppressor genes (51). In the RPE, a mosaic pattern of age-related loss of heme oxygenase-1 gene expression has been observed (30). The mechanism of this loss is unknown, but hypermethylation of gene promoters is an attractive possibility. Age-related silencing might occur for other genes in the RPE as well, contributing to cell–cell variability in phenotype and function.
Implications of RPE Mosaicism
As the descriptions above imply, mosaicism is a normal feature of the RPE. Mosaicism has been observed in many species and is broadly manifest as cell–cell variability in properties both gross (e.g., granule content) and subtle (e.g., expression levels of specific proteins). The normal and widespread nature of RPE mosaicism suggests that it arises through normal and widespread processes, such as the epigenetic mechanisms of gene silencing that occur during normal development and postnatal aging. There is little direct evidence at this time to show that structurally different subsets of RPE cells in the normal monolayer function differently, but it is not unreasonable to speculate that RPE mosaicism may have functional consequences. One might ask, for example, whether naturally occurring subpopulations of differing RPE cells differentially support their adjacent photoreceptors. One might also ask whether environmental factors throughout life that diminish cell function might differentially affect RPE cells in the mosaic, resulting in a differential impact over time on the photoreceptors they serve.
Evidence to support the idea that clonal islands of functionally different RPE cells in the mosaic differentially support their adjacent photoreceptors comes from studies of the Royal College of Surgeons (RCS) rat. These animals have a mutation in the gene encoding the receptor tyrosine kinase Mer (52, 53), which produces a defect in photoreceptor outer segment ingestion by the RPE and a consequent retinal degeneration. Chimeric animals generated between RCS and albino rat strains produce RPE cells exhibiting a pigmentary mosaic, as expected (54). The animals also undergo a retinal degeneration with a mosaic pattern, limited to photoreceptors adjacent to RCS patches of RPE cells. Foci of defective RPE cells, therefore, clearly have local effects on photoreceptor survival.
It is more difficult to demonstrate that variability in complex supportive functions of the RPE produces local differences in photoreceptor longevity. Studies using pigmentary mosaics of the RPE have been undertaken to determine whether RPE melanin content has a direct effect on the degeneration of photoreceptors induced by bright or prolonged light exposure (55, 56). In these studies, no direct spatial correlation could be made between pigmented RPE cells and healthy photoreceptors, which might be expected because melanin is only one of many potential antioxidants that protect the RPE against light damage. Cells low in melanin, for example, could be high in other cytoprotective defenses.
There is indirect evidence, however, that oxidative damage induced by widespread stressors (e.g., light and chemical toxins) has locally differential effects on the RPE. As indicated above, oxidative stress is believed to be one contributor to AMD, yet hallmarks of the disease are focal. One AMD hallmark is drusen, which are local deposits in Bruch’s membrane composed of debris originating from the RPE (57). Another is lipofuscin accumulation within RPE cells (3, 6, 7). Accumulation detected by measures of autofluorescence has an inhomogeneous pattern (58–60), with a spatial relationship to drusen (60). These and other changes in the RPE and underlying Bruch’s membrane may be markers of declining RPE health in the aging eye, which appears to be spotty.
Oxidative damage to the RPE produced by specific toxins also appears to be focal. Formate, the toxic metabolite in methanol poisoning, inhibits mitochondrial function and thereby generates cytotoxic reactive oxygen species. Despite the ubiquitous delivery of formate to the retina, morphologic damage to the RPE (and neural retina), in both humans and animals, is patchy (61, 62). Retinal degenerative diseases resulting from genetic causes are also notoriously heterogeneous, producing local lesions despite a global defect. For example, mutations in retinoid cycle genes, which affect all photoreceptors, produce focal, “white dot” fundus lesions. This multifocal disease phenotype has been attributed to a mosaic of functional subtypes of photoreceptors or of their supporting RPE cells (63).
Given that damaging stimuli can be widespread and yet evoke localized responses in the RPE, we must look to the cells themselves to understand differences in susceptibility. Cellular effects of agents that induce oxidative stress are clearly not universal. Even RPE cells in vitro, which are in a common and controlled environment, do not respond identically when treated with agents that induce oxidative stress. We tend to analyze cells as populations in culture models of oxidative damage, yet scrutiny of individual cell morphology reveals a heterogeneous response. Figure 4⇓ shows a field of cultured RPE cells exposed to the oxidant hydrogen peroxide. Many cells are damaged by the treatment and rounded up, whereas other cells in the same field appear unaffected. Indeed, these resistant cells will go on to re-populate the culture, indicating that they are insensitive to the same concentration of agent that killed their neighbors.
Phase contrast micrograph of a human RPE cell culture showing cell heterogeneity in effects of exposure to a chemical oxidant. The cultures were treated for 24 hr with 400 μM H2O2. Rounded, sensitive cells are adjacent to clusters of flattened, unaffected cells (arrows). Scale bar: 50 μm.
The RPE mosaic of structurally and functionally differing cells in situ presents a backdrop in which complex changes play out over a lifetime, notably those induced by oxidative stress. Even immediately adjacent cells in the young eye may differentially support their associated photoreceptors, and age-related changes can differentially reduce that support function in some cells. Figure 5⇓ illustrates two adjacent RPE cells differing in a few of many cytoprotectant properties known to differ among cells in the mosaic. In the younger eye both RPE cells may adequately support their adjacent complement of photoreceptors. But with aging, HO-1 may decline (30). Melanin’s light protective/antioxidant function may also decline as melanin becomes photooxidized (64), melanosomes fuse with lipofuscin, and lipofuscin levels increase (3, 6, 7, 11). The consequence for susceptible RPE cells may be a more rapid age-related decline in function and a commensurate decline in support for adjacent photoreceptors. One could imagine that photoreceptors associated with progressively dysfunctional RPE cells would be at greater risk for functional impairment and degeneration than those associated with more robust cells (see Figure 5⇓).
Adjacent RPE cells with differing properties, and their overlying photoreceptors. The left RPE cell might be at greater risk for oxidative damage over time because of its lower abundance of photoprotective melanosomes (brown granules), higher abundance of photoreactive lipofuscin (yellow granules), and lower levels of antioxidant enzymes catalase, heme-oxygenase-1 (HO-1), and γ-glutamyltranspeptidase (γGT). The left cell also has an apical domain of short microvilli interacting with overlying photoreceptors, a property that correlates with the patchy expression of E-cadherin in the RPE (27). The cell on right is distinct in each of these properties. Also illustrated is Bruch’s membrane (BrM) on the basal side of the RPE, and photoreceptor outer segments (OS) embedded in RPE apical microvilli. The microvilli vary in length between the two cells.
Whether the patchy photoreceptor atrophy seen in many retinal degenerations results from mosaicism in gene or protein expression in the RPE is currently an unanswered question. Even if it proves to be the case, recognizing that the RPE is a mosaic of variable cells rather than a homogeneous monolayer does not change the impact of aging on the tissue. Indeed, mosaicism is a powerful reminder that finding ways to prevent age-related RPE decline will be daunting because no preventive measure can be universally effective for all cells.
Acknowledgments
JMB receives research support from National Eye Institute grants EY013722, EY015284, Core Grant P30 EY01931, and local Milwaukee donors or foundations (Gene and Ruth Posner Foundation, David and Ruth Coleman Charitable Foundation) supporting macular degeneration research. LMH is also supported by the NEI (EY006473) and both JMB and LMH receive support from unrestricted grants from Research to Prevent Blindness, Inc., to their respective institutions.
- © American Society for Pharmacology and Experimental Theraputics 2005
References

Leonard M. Hjelmeland, PhD, was trained in biophysical chemistry at Stanford University. He is currently Professor of Ophthalmology at UC Davis. Dr. Hjelmeland’s research interests include the genetics of susceptibility to retinal degeneration in the mouse and age-related gene silencing in the RPE.
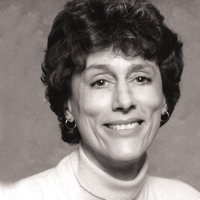
Janice M. Burke, PhD, is the Heil Professor of Ophthalmology and Professor of Cell Biology, Neurobiology and Anatomy at the Medical College of Wisconsin. Cell–cell heterogeneity continues to complicate her research on the role of cadherins in determining RPE cell phenotype, and on the oxidant/antioxidant function of melanin in the aging RPE. Address correspondence to JMB. E-mail jburke{at}mcw.edu; fax 414-456-6304.