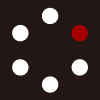
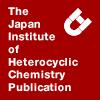
HETEROCYCLES
An International Journal for Reviews and Communications in Heterocyclic ChemistryWeb Edition ISSN: 1881-0942
Published online by The Japan Institute of Heterocyclic Chemistry
e-Journal
Full Text HTML
Received, 16th October, 2008, Accepted, 18th December, 2008, Published online, 24th December, 2008.
DOI: 10.3987/REV-08-SR(D)10
■ Unconventional Activators in the Synthesis of Oligonucleotides and Their Structural Analogues
Wojciech Dabkowski and Jan Michalski*
Centre of Molecular and Macromolecular Studies, Polish Academy of Sciences, Sienkiewicza 112, 90-363 Lodz, Poland
Abstract
Activation of phosphoramidites as phosphitylating reagents in the synthesis of biophosphates and their structural analogues is of great importance. Tetrazole a customary activator can be conveniently replaced by trimethylchlorosilane (TMCS) or 2,4-dinitrophenol. Advantages of use of these reagents in the synthesis of biophosphates and their mechanism of action are discussed. Phosphites containing 4-nitrophenoxy leaving group are activated by DBU which role is revealed. Readily accessible P(III)-F structures, can be “activated” by their facile transformation into the corresponding bromides using trimethylbromosilane (TMBS). Application of phosphoramidites in synthesis of phosphates of biological interest and their activation by azolides and acids salts, including and stereochemical aspects was described in 'Topics in Current Chemistry'.R. L. Letsinger’s original nucleosidyl phosphite synthesis was based on chlorophosphites and was too difficult for general use as it required special precautions.1 The method was modified by M. H. Caruthers who used phosphoroamidites which are relatively stable compounds.2 There was a need to activate the amide leaving group, and tetrazole became the most widely used activator. In spite of impressive progress in this field there are still important problems to be solved. When the necessity arises to produce phosphates of alcohols of biological importance in large quantities, better procedures are required for activation of phosphoroamidites. It also seems to be desirable to find leaving groups other than amide groups at the tricoordinate phosphorus center which are suitable for coupling procedures under alkaline conditions. In this area P(III)-compounds containing an aryloxy group or a fluorine ligand are likely to act as valuable phosphitylating reagents. As expected, these systems show chemical and stereochemical stability. Such P(III)-compounds are of great interest for application in the synthesis of modified oligonucleotides which a contain chiral center at phosphorus atom.
ACTIVATION OF PHOSPHORAMIDITES BY TRIMETHYLCHLOROSILANE
Phosphoramidites are the phosphitylating reagents most often used in the synthesis of various biomolecules such as oligonucleotides, sugar phosphates, phosphoroinositols, and phopsholipides.3,4 An indispensable part of the phosphoramidite strategy is the employment of a proper activator and a suitable protecting group at the phosphorus centre. Coupling with alcohols is controlled by steric and electronic effects. Bulky substituents in the amido group can help to control selectivity of a coupling, preventing formation of a symmetrical structure. This can be achieved by employing N,N-diisopropylamino derivatives which are most frequently used because of their comparatively high stability. Relatively low reactivity of N,N-diisopropyl amidites is caused by electronic and steric factors can be compensated by appropriate activation. Tetrazole is by far the most commonly used activator in coupling reactions of phosphoroamidites with alcohols and other nucleophiles. However this activator suffers from some disadvantages. Tetrazole is expensive, explosive, hygroscopic and sparingly soluble in acetonitrile, the solvent most frequently used in the coupling reaction. It may induce transesterification of trialkylphosphites.5 Tetrazole is not effective, or must be used in large excess, when strongly electronegative substituents are attached to the PIII center.6 These problems have become even more pronounced in the synthesis of oligonucleotide phosphorothioates at production scale.
Our earlier work on interaction of P(III) amides with halogenosilanes suggested, that phosphoramidites would react with alcohols in the presence of trimethylchlorosilane (TMCS) as catalyst.7 Indeed the reaction of P(III) amidites with an equivalent amount of nucleoside proceeds in the presence of TMCS in very high yield and at rates comparable or higher than those when tetrazole is used. Phosphitylations activated by TMCS proceed at room temperature in solvents such as THF, CH2Cl2 or MeCN. On average, the amount of activator required for an efficient coupling is ca. 30-60% of the stoichiometric ratio.8
The mechanism of activation by TMCS is presumed to involve its reaction with P(III) amide (Scheme 1). The first hypothetical step produces the salt-like species R2P+(SiMe3)NR’2Cl- or R2PN+R’2(SiMe3)Cl- which react either directly with alcohol to give ester R2POR or via intermediate formation of R2PCl. Attempts to evidence formation of salt-like species by 31P NMR spectroscopy failed. Formation of R2PCl was confirmed by 31P NMR spectroscopy. In both cases TMCS is regenerated. The following cycle involved in the activation is proposed.
An alternative mechanistic path may be considered in which TMCS reacts with alcohol to form hydrogen chloride which then activates an P(III)-amide in situ. But it is well known that TMCS reacts very slowly with alcohols unless a catalyst is present.9
An example of activation in the presence of a catalytic amount of TMCS (0.6 equiv.) is the reaction of thymidine (1) with tris(dimethylamino)phosphine to give thymidine 3’,5’-cyclic dimethylphosphoramidite (2) in 95% yield (Scheme 2).8 Activation by tetrazole is visibly less effective.10
Commercially available bis(diisopropylamino)-2-cyanoethoxyphosphine reacts selectively in the presence of TMCS (0.6 equiv.) with 5’-O-DMTr-nucleoside (3a, b) to form 5’-O-DMTr-thymidine 3’-O-(2-cyano-N,N-diisopropyl)phosphoramidite or N6-benzoyl-5’-O-DMTr-deoxyadenosine 3’-O-(2-cyanoethyl-N,N-diisopropyl)phosphoramidite (4b) in over 97% yield (Scheme 3). The yield and purity of amidites are identical to those obtained by activation with tetrazole.11 This type of procedure has been applied to a wide variety of alcohols of biological interest. The activation works well with sterically hindered alcohols. Formation of hydrogen chloride would effect the removal of the acid labile dimethoxytrityl DMTr protection group used in the synthesis of oligonucleotides. This is actually observed when commercial TMCS is contaminated with HCl. When TMCS is free of hydrogen chloride the DMTr group is retained.12
O-Methyl-O-4-nitrophenyl-N,N-diisopropylphosphoroamidite, which can be qualified as a bidentate phosphitylating reagent having two different leaving groups, is readily available and shows high stability. Michalski et al. have applied this reagent in the synthesis of natural and modified dinucleotides.13 An example of its use in a synthetic procedure is given in Scheme 4.
The intermediate mononucleoside phosphites (6a, b) are formed via activation either of the 4-nitrophenoxy group of (5) by DBU (step a) or of the diisopropylamido group by TMCS (step b). Dinucleoside methylphosphite (7) was prepared from the corresponding monophosphites (6a, b) in a similar manner (step c and d).
Fast oxidation by dry air or elemental sulfur (selenium) was observed and attributed to the presence of 4-nitrophenoxide anion (step e). Even more remarkable is high capability of the 4-nitrophenolate anion to demethylate the intermediate phosphate (8) (step f). This step is so rapid that we were unable to observe the intermediate methylphosphate or corresponding sulfur or selenium analogues (8). This series of reactions can be performed as a one-flask procedure with a total yield of (9) in over 90%. The involvement of DBU 4-nitrophenolate in the oxidation of methylphosphonites R2P-OMe and dealkylation of R2P(X)OMe structures has been confirmed on several model compounds.14
Phosphoramidites containing a strongly electronegative group are more efficiently activated by TMCS than by tetrazole (Scheme 5). For example, bis(O-4-nitrophenyl)-N,N-diisopropylphosphoramidite (10) is an efficient phosphitylating reagent via exchange of the aryloxy group. Exchange of the amido group is not practicable with tetrazole activation but can be readily performed in the presence of TMCS to give 11.15
It has been also demonstrated that activation by tetrazole may give rise to some side reactions which can be avoided when TMCS is used.16
Trimethylbromosilane (TMBS) is a more potent activator of phosphoramidites than TMCS (Scheme 6). However its application raises additional problems. TMBS may cause the rearrangement of an P(III) amidoester (12) into a >P(O) structure (13) with formation of a P-C bond.17 TMCS is inert to this type of reaction.
ACTIVATION OF PHOSPHORAMIDITES BY 2,4-DINITROPHENOL.
Dabkowski et al. have found that 2,4-dinitrophenol (DNP), whose pKa value 4.1 is close to that of tetrazole (4.9), acts as an efficient activator of phosphate synthesis via the phosphoroamidite procedure.16
The reaction of P(III) amidites with an equivalent amount of nucleoside in the presence of DNP proceeds in very high yield and at rates comparable or higher than those when tetrazole is used. Phosphitylations activated by DNP take place at room temperature in aprotic solvents like THF, CH2Cl2 or MeCN. On average the optimal amount of activator required for efficient coupling is ca 1.5 equivalents of the stoichiometrical ratio. As in the case of TMCS, phosphoramidites (14) containing a strongly electronegative group like fluorine at the P(III) center are more efficiently activated by DNP than by tetrazole and react with 3’-O-DMTr-thymidine (15) yielding the nucleoside phosphorofluoridite (16) (Scheme 7).
Similar situations involving the electronegative leaving group OCH(CF3)2 have been observed in the phosphitylating procedure introduced by Tataku et al. (Scheme 8).19 Both steps a and b are highly selective and final dinucleotides (18) are formed in over 95% yield from amidite (17) at room temperature in THF solution.
Mechanistic features of the DNP activation are shown in Scheme 9.
Studies on activation by DNP have paved the way for a novel type of phosphitylating reagent. Spontaneous displacement of the 2,4-dinitrophenoxy group in the reaction of the reagent with alcohols liberates DNP. The latter activates the amino group allowing further ligand exchange. However if phosphitylation of 3a by the bis(2,4-dinitrophenyl)phosphoroamidite (19) is performed in the presence of one equivalent of triethylamine, high chemoselectivity is observed, and the nucleosidyl (2,4-dinitrophenyl)phosphoramidite is formed in over 92% yield (Scheme 10).
ROLE OF DBU IN THE REACTION OF P(III)-OAR SYSTEMS WITH ALCOHOLS
DBU is often referred to as non-nucleophilic strong base. On the other hand the efficiency of DBU in some transformations is related to its nucleophilicity. The procedure involving displacement of a 4-nitrophenoxy ligand by a nucleosidyl-OH group may qualify for the latter category. The involvement of a (=P-N≡)+ cationic species has been demonstrated in phosphitylation of nucleosides in the presence of DBU by 31P NMR studies and its reaction with benzoyl fluorides (Scheme 11).20
PHOSPHITYLATING REAGENTS CONTAINING A PHOSPHORUS-FLUORINE BOND
Easy access to P(III)-OAr compounds allowed us to synthesize a number of P(III)-F structures derived from nucleosides and other alcohols of biological interest.
The synthetic pathway leading to the dinucleosidyl phosphorofluorides (24) is shown in Scheme 12.21
The mono nucleosidyl phosphoroamidofluoridites (22) are formed from 21 with some degree of stereoselectivity and can be separated into pure diastereoisomers. However, their coupling reaction with 3’O-protected nucleosides in the presence of TMCS affords dinucleosidyl phosphorofluoridite (22) as 1:1 mixtures of diastereomers in almost quantitative yield. It is noteworthy that this coupling does not affect the phosphorus-fluorine bond. Chromatography of phosphorofluoridites on silica gel gives pure ‘’fast’’ isomers of high configurational stability. This is the first case in nucleotide chemistry of obtaining relative stable P(III)-F diastereoizomers with a chirality center at the phosphorus atom. Relatively little is known about the chemistry and stereochemistry of organophosphorus compounds containing the P(III)-F functional centre. trans-2-Fluoro-4-methyl-1,3,2-dioxaphosphorinane has been prepared by Mikolajczyk et al.22 The first resolution of a free fluorophosphane MePhPF has been achieved only recently.23 Interestingly, the optical stability of this phosphane was much lower than of those of dinucleosidyl phosphorofluorides.
The configurational stability of phosphorofluoridites can be explained by the presence of the strong P-F bond and steric hindrance exerted by the nucleosidyl groups. Diastereoisomeric P(III)-F nucleotide compounds racemize in the presence of F- donors via achiral intermediates containing two fluorine ligands in apical positions.24 Pure diastereoisomers separated by chromatography were transformed in a stereospecific way into the corresponding P(X)-F (X=S, Se) compounds (24). It is most likely that the transformations presented above proceed with retention of configuration at the stereogenic center connected to the phosphorus atom.
As expected, compounds containing a thiophosphoryl centre are distinctly more resistant towards hydrolysis and other nucleophilic displacements than their oxo analogues. The hydrolytic susceptibility of phosphorofluoridates and phosphorofluoridothionates is strongly influenced by the presence of fluoride ions.25 The relatively high stability of phosphorus(III) phosphorofluoridites towards hydrolysis is somewhat surprising.
Two new readily available amidite reagents containing a fluorine group have been synthesized in our laboratory. Both phosphitylating reagents (25a, b) containing tert-butyl or 2-cyanoethyl protecting group react with alcohols in the presence of TMCS at ambient temperature (Scheme 13, steps a and d).
The latter reagent proved to be the superior activating reagent. The corresponding fluorophosphates (26a, b) were formed in very high yield. Oxidation by hydrogen tert-butyl peroxide or addition of elemental sulfur (selenium) (steps c and d) proceed in very high yield to give the intermediate phosphorofluoridates (27a, b) or their sulfur and selenium analogues. The final steps e and f involve thermal elimination of 2-methyl-1-propene or acetonitrile respectively to give the desired fluorophosphorus, fluorothio and fluoroseleno acids in very high yield. Reactions described in Scheme 13 are best performed as one flask
procedures with overall yield above 95%. It is noteworthy that in the above coupling procedure activated by TMCS, the tert-butyl protective group is unaffected. The method described in Scheme 13 is more convenient than the one described by us earlier26 and also by other authors.27
THE ACTIVATION OF P(III)-F GROUP
Compounds containing the P(III)-F moiety are visibly inert towards alcohols. They react selectively with organometallic reagents to form P(III)-C bonds without affecting the nucleosidyloxy residue.14
Our recent studies show that P(III)-F structures containing a fluorine ligand can be very readily converted into P(III)-Br bromides by reaction with TMBS or trimethyliodosilane (Scheme 15).14
The driving force of these reactions seems be a high affinity of fluorine toward the silicon center. Me3SiF is inert to alcohols. This type of activation of P(III)-F structures opens a new perspective for a variety of phopsphitylation procedures.14
ACKNOWLEDGEMENTS
The authors’ work was supported in part by the Ministry of Science and Higher Education, Poland (1 T09A 139 30).
References
1. R. L. Letsinger and W. B. Lunsford, J. Am. Chem. Soc., 1976, 98, 3655. CrossRef
2. S. L. Beaucage and M. M. Caruthers, Tetrahedron Lett., 1981, 22, 1859. CrossRef
3. The comprehensive source of information concerning oligonucleotides and other biophosphates up to 1990 are reviewed. a) E. Uhlman and A. Peyman, Chem. Rev., 1990, 90, 453; CrossRef b) S. L. Beaucage and R. P. Iyer, Tetrahedron, 1992, 48, 2223; CrossRef c) S. L. Beaucage and R. P. Iyer, Tetrahedron, 1993, 49, 1925; CrossRef d) S. L. Beaucage and R. P. Iyer, Tetrahedron, 1993, 49, 6123; CrossRef e) S. L. Beaucage and R. P. Iyer, Tetrahedron, 1993, 49, 10441; CrossRef f) E. E. Nifantiev, M. K. Grachev, and S. Yu. Burmistrov, Chem. Rev, 2000, 100, 3755; CrossRef g) R. Strömberg and J. Stawinski, ”Current Protocols in Nucleic Acid Chemistry”, ed. by S. L. Beaucage, D. E. Bergstrom, G. D. Glick, and R. A. Jones, John Wiley & Sons, New York, 2000, , pp. 3.4.1-3.4.11; h) Y. Hayakawa, Bull. Chem. Soc. Jpn., 2001, 74, 1547. CrossRef
4. J. Michalski and W. Dabkowski, ‘Topics in Current Chemistry’, ed. by J.-P. Majoral, 2004, 232, 93.
5. Y. Watanabe, S-i. Maehara, and S. Ozaki, J. Chem. Soc., Perkin Trans. 1, 1992, 1879. CrossRef
6. a) R. M. Karl, W. Richter, R. Klösel, M. Mayer, and I. Ugi, Nucleotides & Nucleotides, 1996, 15, 379; CrossRef b) W. Dabkowski, I. Tworowska, J. Michalski, and F. Cramer, J. Chem. Soc., Chem. Commun., 1995, 1435; CrossRef c) Y. S. Sanghvi, Z. Guo, H. M. Pfundheller, and A Converso, Org. Proc. Res. Dev., 2000, 4, 175. CrossRef
7. a) J. Chojnowski, M. Cypryk, and J. Michalski, J. Organomet. Chem., 1981, 215, 355; CrossRef b) M. Cypryk, J. Chojnowski, and J. Michalski, Tetrahedron, 1985, 41, 2471. CrossRef
8. W. Dąbkowski, I. Tworowska, J. Michalski, and F. Cramer, J. Chem. Soc., Chem. Commun., 1997, 877. CrossRef
9. a) J. G. Lee and K. K. Kang, J. Org. Chem., 1988, 53, 3634; CrossRef b) D. C. Snyder, J. Org. Chem., 1995, 60, 2638. CrossRef
10. a) G. Baschang and V. Kvita, Angew. Chem., 1973, 44, Angew. Chem. Int. Ed. Engl., 1973, 12, 71; CrossRef b) G. S. Bajwa and W. G. Bentrude, Tetrtahedron Lett., 1978, 5, 421. CrossRef
11. A. Kraszewski and K. E. Norris, Nucleic Acids Res. Symp. Ser., 1997, 18, 177.
12. W. Dabkowski, I. Tworowska, J. Michalski, and F. Cramer, Chem. Commun., 1997, 877. CrossRef
13. J. Helinski, W. Dabkowski, and J. Michalski, Nucleosides and Nucleotides, 1993, 12, 597. CrossRef
14. Unpublished results from this laboratory.
15. E. Marsault and G. Just, Nucleosides & Nucleotides, 1998, 17, 939. CrossRef
16. a) S. Eisenhard, Doctoral Disseration, Dept. Org. Chem., Frankfurt University, 1997; c) I. Tworowska, Doctoral Disseration, Centre of Molecular and Macromolecular Studies, Polish Academy of Sciences, 2000.
17. W. Dabkowski, A. Ozarek, S. Olejniczak, M. Cypryk, J. Chojnowski, and J. Michalski, Chem. Eur. J., in press.
18. W. Dabkowski, I. Tworowska, J. Michalski, and F. Cramer, Tetrahedron Lett., 2000, 41, 7535. CrossRef
19. H. Tataku, T. Watanabe, and S. Hamamoto, Tetrahedron Lett., 1988, 29, 81. CrossRef
20. a) W. Dabkowski, I. Tworowska, L. Kazimierczak, and J. Michalski, Polish J. Chem., 2005, 79, 467; b) W. Dabkowski, A. Ozarek, and I. Tworowska, New J. Chem., 2005, 29, 1396. CrossRef
21. W. Dabkowski, A. Ozarek, and I. Tworowska, New J. Chem., 2005, 29, 1396. CrossRef
22. M. Mikolajczyk, J. Krzywanski, and B. Ziemnicka, Tetrahedron Lett., 1975, 16, 1607. CrossRef
23. M. Pabel, A. C. Willis, and S. B. Wild, Inorg. Chem., 1996, 35, 1244. CrossRef
24. L. D. Quin, ‘A Guide to Organophosphorus Chemistry’, John Wiley & Sons Inc Publication, New York, 2000.
25. a) R. J. P. Corriu, J.-P. Duthei, and G. F. Lanneau, J. Am. Chem. Soc., 1984, 106, 1060; CrossRef b) R. J. P. Corriu, G. F. Lanneau, and D. Leclerq, Tetrahedron, 1989, 45, 1959. CrossRef
26. W. Dabkowski and I. Tworowska, Chem. Lett., 1995, 8, 727. CrossRef
27. a) R. Wittmann, Chem. Ber., 1963, 96, 771; CrossRef b) C. Sund and J. Chattopadhyaya, Tetrahedron, 1989, 45, 7523; CrossRef c) J. Stawinski and M. Bollmark, Tetrahedron Lett., 1996, 37, 5739; CrossRef d) K. Misiura, D. Szymanowicz, and W. J. Stec, Chem. Commun., 1998, 515. CrossRef