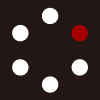
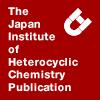
HETEROCYCLES
An International Journal for Reviews and Communications in Heterocyclic ChemistryWeb Edition ISSN: 1881-0942
Published online by The Japan Institute of Heterocyclic Chemistry
e-Journal
Full Text HTML
Received, 24th August, 2010, Accepted, 12th October, 2010, Published online, 13th October, 2010.
DOI: 10.3987/COM-10-S(E)112
■ Non Covalent Inclusion of Nucleosides and Nucleotides in Water-Soluble Molecular Clips
Frank Bastkowski, Jolanta Polkowska, Thomas Schrader,* and Frank-Gerrit Klärner*
Institute of Organic Chemistry, University of Duisburg-Essen, Universitätsstr. 5, 45141 Essen, Germany
Abstract
The dimethylene-bridged molecular clips having naphthalene sidewalls and bearing either lithium phosphate or lithium methanephosphonate groups in the central benzene-spacer-unit bind various nucleosides and nucleotides in buffered aqueous solution at pH = 7.2. The binding constants (Ka) and the complexation-induced 1H NMR shifts of the guest signals (Δδmax) were determined by NMR titration experiments. The host-guest complexes of the phosphate-substituted clip with caffeine and theophylline (Ka = 31400 or 16800 M-1) are more stable than those with cytidine and uridine (Ka = 5240 or 5390 M-1) and with adenosine and guanosine (Ka = 1470 or 1120 M-1). In the case of the phosphonate-substituted clip 2 the selectivity in the complex formation toward one type of nucleoside is, however, less pronounced. The complexes of the nucleotides such as AMP, GMP, CMP, or UMP with both clips are less stable than the corresponding complexes of the nucleosides. To understand the observed selectivities in the complex formation we discuss attractive and repulsive electrostatic interactions on the basis of electrostatic potential surfaces (EPS) calculated for host and guest molecules by quantum chemical methods and hydrophobic effects which contribute to the complex stability to a large extent. The observed large complexation-induced 1H NMR shifts (Δδmax) of the guest signals provide good evidence that in each complex the nucleobase is encapsulated inside the clip cavity.INTRODUCTION
Nucleosides and nucleotides play an important role in many biological systems. They serve as building blocks in DNA synthesis and as enzyme cofactors (for example (NAD(P)+/NAD(P)H in many redox processes. They regulate energy- and electron-transfer processes.1 Furthermore, derivatives are used in medicine, e. g. AZT as antiviral drug in the AIDS therapy.2 Many of these functions rely on the molecular recognition of specific protein binding sites by these compounds. Therefore, the development of synthetic host molecules for the specific complexation of nucleosides and nucleotides has been an important target in supramolecular chemistry. Earlier examples of such host molecules in organic media employ hydrogen bonding and aromatic π−π stacking motifs complementary to those of the nucleobases to be recognized.3 The selective recognition of nucleosides and nucleotides in aqueous media has been a more challenging task because hydrogen bonding interactions and salt bridges, which are frequently used as binding motifs in organic media, become relatively weak in water.4 In aqueous solution hydrophobic effects are important and in cooperation with the other noncovalent interactions (such as charge-charge, charge-dipol, π-stacking, and hydrogen bonding interactions) they provide a major contribution to the stability of supramolecular adducts in water. Macrocyclic and tweezer-like host molecules,5 peptide receptors,6 and macromolecular pores containing hydrophobic clefts and/or cationic binding sites7 have been reported to bind nucleotides at the phosphate unit additionally to the nucleobase. Only few host molecules are known which complex nucleosides (lacking of the phosphate groups), too. Most recently, we reported the water-soluble molecular clips 1 and 2 with naphthalene sidewalls and a central benzene spacer-unit bearing solubilizing dilithium phosphate or lithium methanephosphonate substituents.8,9 These clips bind electron-deficient pyridinium, thiazolium, and sulfonium salts such as N-methylnicotinamide iodide (NMNA), nicotinamide adenine dinucleotide (phosphate) (NAD(P)+), thiamine diphosphate (TPP), and S-adenosylmethionine as guest molecules inside their electron-rich cavity in buffered aqueous solution at neutral pH value. A detailed 1H NMR study of the complex of NAD+ with 1 and 2 provided first evidence that the adenine unit is also bound inside the clip cavity albeit weaker than the positively charged nicotinamide ring.10 This finding is supported by the observation that adenosine monophosphate (AMP) and NADH (the reduced form of NAD+) also forms host-guest complexes with the clips 1 and 2 by inclusion of the adenine unit inside the clip cavity. In this work we systematically studied the host-guest complex formation of nucleosides and nucleotides with aim to find out, if there is selectivity in the binding depending on the nature of the nucleobase (purine vs. pyrimidine) and which effects the negatively charged nucleotide phosphate groups and the nature of the sugar have on the complex stability (nucleosides vs. nucleotides and ribose vs. deoxyribose, respectively). Furthermore, we studied the complex formation of the related derivatives azidothymidine (AZT), caffeine (CAF), and theophylline (THE) with the molecular clips 1 and 2.
RESULTS AND DISCUSSION
Recently, we reported the complex formation of molecular clip 2 with various nucleosides of the deoxyribose family such as 2’-deoxyadenosine, 2’-deoxyguanosine, 2’-deoxythymidine, etc. Showing binding constants Ka between 1900 and 7400 M-1 in pure water.11 More recently, nucleotides such as NAD+ and AMP were found to form stable self-aggregates in pure water and not to be bound inside the clip cavity in their complexes with 2. In buffered aqueous solution at pH = 7.2 these nucleotides exist, however, as monomers and their nicotinamide ring and/or adenine-unit are, indeed, included inside the clip cavity. The phosphate-substituted clip 1 reacts slightly basic (pH = 8.5, [1] = 5.3 mM) and the phosphonate-substituted clip 2 almost neutral (pH = 7.2) in pure water.10 Therefore, 1 is partially protonated whereas 2 remains unchanged in buffered solution at pH = 7.2. The notation ʻclip 1ʼ in Tables 1-3 means, that 1 was used as starting material for the studies in buffer. From the concentration dependence of the 1H NMR spectra of 1 and 2 in buffered aqueous solution both clips were extrapolated to form relatively weak self-assembled dimers (25 °C: Kdim [M-1] = 140 (1) and ≤ 30 (2)). Thus, clips 1 and 2 having naphthalene sidewalls largely exist as monomers in dilute solution contrary to the corresponding clip molecule bearing phosphonate substituents in the central benzene spacer-unit and having extended anthracene sidewalls (25 °C: Kdim [M-1] = 1.6 x 105).12 The weak dimerization of 1 and 2 has not been considered for the study of their host-guest complex formation with nucleosides and nucleotides.
Due to the magnetic anisotropy of the clip arene-units 1H NMR spectroscopy represents a very sensitive probe for uncovering the complexation mode of guest molecules. The binding of the guest molecule inside the clip cavity can be easily detected by pronounced up-field shifts of the guest signals in the 1H NMR spectrum of a host-guest mixture. The maximum complexation-induced 1H NMR shifts of the guest protons, Δδmax (Δδmax = δ0,g − δΗg), and the binding constants, Ka, were determined by the methods of 1H NMR titration from the dependence of the observed complexation-induced 1H NMR shifts, Δδobs (Δδobs = δ0,g − δobs), of the guest protons on dilution of the host concentration at constant guest concentration.13 The results of the 1H NMR titration experiments with clips 1 and 2 as host molecules and various nucleosides and nucleotides containing purine or pyrimidine derivatives as guest molecules are shown in Tables 1-3.
The complexes of the clips 1 and 2 with adenine-containing nucleosides and nucleotides (Table 1) show large complexation-induced 1H NMR shifts for guest proton 8-H (Δδmax = 1.51 – 3.68 ppm) indicating, that the adenine ring is included inside the clip cavity (for a more detailed discussion of the complex structures vide infra). The binding constants, Ka, were determined to be significantly larger for the complexes of adenosine (A) and deoxyadenosine (DA) than those found for AMP, ADP, or ATP. Evidently, the repulsive interaction between the negatively charged phosphate groups in the nucleotides and the phosphate or phosphonate groups of the clips leads to a weakening of the complexes of AMP, ADP, ATP, or DAMP with clip 1 or 2. This explanation is supported by the finding that the complex of 1 with the singly charged nucleotide cAMP is more stable than the corresponding complexes with the doubly, triply, and quadruply charged nucleotides AMP, ADP, ATP, or DAMP. According to the data listed in Table 1, the increase in charges at the remote oxygen atoms of ADP and ATP does affect a further weakening of the complexes compared to that of AMP. Similar results were obtained for the complexation of the nucleosides and nucleotides containing guanine as nucleobase (Table 1). The complexation-induced 1H NMR shifts of guest proton 8-H at the guanine unit are substantially smaller than those observed for corresponding proton in the adenine-containing complexes. This finding already indicates that the position of guanine-containing guest molecule inside the clip cavity has to be different to that of the adenine-containing guest molecules. The binding constants of the complexes of 1 and 2 with the guanine-containing guest molecules are relatively small showing no clear tendency. Presumably, the interaction of the electron negative NH2 group attached to C-2 of the guanine unit with the electrostatically negatively polarized clip cavity is here repulsive resulting in a complex weakening.
The pyrimidine-containing nucleosides cytidine C and uridine U form substantially more stable complexes with phosphate clip 1 than with phosphonate clip 2 (Table 2). They are also more stable than the corresponding complexes of the purine-containing nucleosides A • 1 or G • 1 (Table 1). The complexes of both clips with the pyrimidine-containing nucleotides, however, are weak. Their stabilty is comparable to that of the coresponding complexes of the nucleotides containing adenine or guanine as nucleobase. This finding indicates again that the repulsive interaction between the nucleotide phosphate groups and negatively charged clips determines the complex stability to a significant extend. The little stability of the complexes of AZT with clips 1 and 2 is surprising and not well understood because AZT is a formally neutral nucleotide lacking of the negatively charged phosphate group. The relatively large complexation-induced 1H NMR shift of the 5-methyl signal of AZT (Δδmax = 1.82 ppm) in the complex with 1 may be, however, an indicator that this methyl group is located inside the clip cavity (contrary to the structure calculated by force field shown in Figure 3). All complexes of the pyrimidine-containing nucleosides and nucleotides again show relatively large complexation-induced 1H NMR shifts (Δδmax) of the guest protons attached to the six-membered pyrimidine ring. This finding is good evidence that also in these complexes the nucleobase is encapsulated inside the clip cavity.
Finally, the purine derivatives caffeine (CAF) and theophylline (THE) form stable complexes with both clips 1 and 2 (Table 3) which are more stable than the corresponding complexes with all the nucleosides and nucleotides discussed above. Their stability is comparable to that of host-guest complexes with other caffeine host molecules.14 In the 1H NMR spectra of the mixtures of the clip molecules with caffeine or theophylline the signal of 8-H becomes broad and could not be detected. Therefore, only the complexation-induced 1H NMR shifts of the signals (assigned to the methyl groups of CAF and THE) could be determined. The complexation-induced shifts of these guest signals were determined to be Δδmax ≤ 1 ppm: This finding suggests that the methyl groups of both guest molecule are positioned outside the clip cavity.
In most cases the complex of the phosphate clip 1 is more stable than the corresponding complex of the methanephosphonate clip 2. This finding is in agreement with the function of these clips as inhibitors of enzymes which use NAD(P)+ as cofactors. For example, clip 1 inhibits the oxidation of glucose-6-phosphate with NADP+ catalyzed by glucose-6-phosphate dehydrogenase much more efficiently than clip 2. There are two reasons why 1 may be a better host molecule than 2. (1) Clip 1 exhibits a larger over all negative charge than clip 2 even though the phosphate groups of 1 are partially protonated in buffer at pH =7.2). Thus, electron-poor guest molecules are electrostatically attracted by clip 1 more efficiently than by clip 2. (2) The methyl groups at the phosphorous atoms of clip 2 may sterically hinder the inclusion of the guest molecule inside the clip cavity particularly if the phophonate groups point toward the clip cavity. The O- or OH groups at the phosphorous atoms of clip 1 are sterically less bulky than the methyl groups of 2 and may undergo further attractive interactions with the guest molecule e. g. via hydrogen bonding. There are only few, hitherto not well understood exceptions of complexes of clip 2 which are more stable than the corresponding complexes of clip 1, for example the complexes AMP • 2, GMP • 2 and AZT • 2. In the case of AZT • 1 and AZT • 2 both complexes are relatively weak compared to the complexes of the other neutral nucleosides which is not understood either.
To explain the substantial differences in the stability of the complexes of clip 1 with purine-containing nucleosides adenosine A and guanosine G on the one hand and the pyrimidine-containing nucleosides cytidine C and uridine U on the other hand, we calculated the electrostatic potental surfaces (EPS) of the nucleobases (Table 4).15 EPS calculations helped us to understand why in organic media the molecular clips and the related molecular tweezers preferentially bind guest molecules having positive electrostatic potential surfaces which are complementary to the negative EPS inside the clip or tweezer cavity (for the EPS of clip 1 see Table 5, left).13 In organic media the complex stability correlates well with the magnitude of the positive guest EPS. From the structural data (vide infra) one can assume that in each complex observed here the six-membered ring of the nucleobase is encapsulated inside the clip cavity. Therefore, we calculated the molecular electrostatic potentials (MEP) in the center of six-membered ring of each nucleobase.16 The MEP at this position of uracil, thymine, caffeine, or theophylline is, indeed, more positive than that of adenine, guanine, or cytosine. This result seems to explain why the complexes of clip 1 with uridine U, caffeine CAF, and theophylline THE are more stable than those with adenine A and guanidine G but it does not explain why the complex with cytidine C is as stable as and the complex with AZT is much less stable than that with uridine U.
The comparison of the EPS of adenosine A and adenosine monophosphate AMP (Table 5) with the EPS of adenine (Table 4) shows that the substitution by ribose or ribose monophosphate has only a minor effect on the MEP of the adenine ring.16 This finding allows the conclusion, that the EPS of the adenine ring is of minor importance for the different stability of the complexes A • 1 and AMP • 1, and supports the assumption (mentioned earlier), that the repulsive interaction between the anionic host and guest phosphate or phosphonate groups causes the smaller stability of the complexes of the nucleotides (compared to those of the neutral nucleosides). In aqueous solution the complex stability is, however, essentially determined by hydrophobic host-guest interactions in addition to all other binding interactions. The hydrophobic effect is dependent of the size and shape of the interacting systems. Examples for the size-dependence of the hydrophobic forces are the already mentioned phosponate-substituted clips having either naphthalene sidewalls (compound 2) or anthracene sidewalls.12 Only the anthracene clip forms a highly stable dimer in water due to the stronger hydrophobic interactions of the extended sidewalls. Here, there is no obvious correlation between the size of the nucleobase and its complex stability, because the number and nature of the hydrophilic heteroatoms and functional groups of the nuleobases are certainly also important for the hydrophobic character of these compound. To gain further insight into the remarkable selectivity in the complex formation between molecular clips and nucleosides or nucleotides, quantum chemical calculations will be necessary which take the aqueous solvation into consideration.
The complex formation between the clips and nucleosides or nucleotides influences not only the chemical 1H NMR shifts of the guest protons but also those of the host protons. Figure 1 shows the 1H NMR signals assigned to the protons of the naphthalene sidewalls of clip 1 (a) in the mixture with adenosine A and (b) without A. In the presence of A a remarkable splitting of the 1H NMR signals is observed for the naphthalene protons which are equivalent in the free clip. In the complex the protons a-H and f-H show two separate singlets and the protons b-H to e-H an ABCD spectrum instead of one singlet and an A2B2 spectrum, respectively, in free clip. A similar splitting of the 1H NMR clip signals was also observed in the complexes with other chiral guest molecules such as NAD+ 10 or the nucleosides and nucleotides described here. Evidently, complex dissociation and association as well as conformational equilibration between the different complex structures proceed rapidly with respect to the “NMR time scale”. These dynamic processes lead to an averaging of the NMR signals of the complex and make the corresponding protons at the two naphthalene sidewalls of complexed 1 equivalent to each other, whereas the front- and backside protons of each naphthalene sidewall remain nonequivalent. This finding can be explained with a chirality transfer of the chiral guest adenosine A to the achiral clip 1.
The large complexation-induced 1H NMR shifts (Δδmax) of the guest signals assigned to the protons of the nucleobases (Table 1-3) already suggests that the nucleobase is included inside the clip cavity. This assumption is supported by the quantum chemical ab initio calculation of the chemical 1H NMR shifts of several nucleotides such as NAD+, NADH, or AMP in the complexes with clip 1 or 2. In Table 6 the experimental Δδmax values are compared with those calculated for the structure of complex AMP • 1 shown in Table 6a). The good agreement between experimental and calculated data strongly supports the proposed complex structure. Evidently, in this complex proton 8-H at the five-membered ring of adenine (pointing toward the central clip arene spacer-unit) experience the larger shielding caused by the magnetic anisotropy of clip arene-units than proton 2-H which is attached at the six-membered ring and positioned outside the clip cavity.
In complex A • 1 both adenine protons 2-H and 8-H show almost equal Δδmax values (2.20 and 2.15 ppm) indicating that both protons are equally influenced by the magnetic anisotropy of the clip arene-units. For the calculated structure shown in Figure 2 (a) one would expect a different shielding of these two protons as it was observed for complex AMP • 1. The finding of equal shielding of both protons 2-H and 8-H in complex A • 1 indicates a dynamic equilibrium between at least two complex structures (Figure 2 (a) and (b)) which is rapid on the ´NMR timescale`, so that averaged chemical shifts are observed for these two protons which are larger than the Δδmax value of 2-H and smaller than that of 8-H observed for complex AMP • 1. In the complexes of the guanosine derivatives only the shielding of the signal assigned to 2-H could be observed due to a specific broadening of the 8-H signal in presence of clip 1 or 2. The relatively small complexation-induced 1H NMR shifts of the 2-H signals (Δδmax = 0.17 – 1.48 ppm, Table 1) suggest complex structures in which this proton is positioned outside the clip cavity as it is shown for the example of complex G • 1 (Figure 2c). The large Δδmax values observed for the signals of 2-H and 3-H in the complexes of the cytidine and uridine derivatives (Table 3) indicate that these protons strongly experience the magnetic anisotropy of the clip arene-units and presumably point toward the clip central arene spacer-unit as it is shown for the calculated complex structures C • 1 and U • 1 (Figure 2d-f).
The relatively large Δδmax values observed for the signals of 2-H in the complexes AZT • 1 and AZT • 2 (Table 2) are in agreement with the calculated stucture of complex AZT • 1 shown in Figure 3a as one example. Relatively small Δδmax values were found for methyl signals of caffeine (CAF) and theophylline (THE) in their complexes with clip 1 or 2 indicating that the methyl groups of both guest molecules poit outside the clip cavity as it is shown in the examples of the calculated complex structures (Figure 3b,c).
Conclusion. The pyrimidine-containing nucleosides cytidine (C) and uridine (U) form host-guest complexes with the phosphate-substituted clip 1 in buffered aqueous solution at pH =7.2 which are substantially more stable than the corresponding complexes of the purine-containing nucleosides adenosine (A) and guanosine (G). The purine derivatives caffeine (CAF) and theophylline (THE) form even more stable complexes with clip 1 than cytidine and uridine. Since CAF and THE do not possses a ribofuranose moiety, this finding allows the conclusion that the nucleosides and nucleotides show a reduced binding to the clips just because of the steric bulk of the ribofuranose moiety. In the case of the phosphonate-substituted clip 2 the selectivtiy in the complex formation toward one type of nucleoside is, however, less pronounced. The stability of these host-guest complexes is determined by a combination of several attractive and repulsive noncovalent interactions which are difficult to be predicted. To understand the selectivity found here for the complex formation, the electrostatic potential surface (EPS) of the nucleobases and of caffeine and theophylline were calculated. Indeed uracil, caffeine, and theophylline show a more positive molecular electrostatic potential (MEP) in the center of each six-membered ring than adenine and guanidine. The positive MEP is complementary to the negative MEP inside the clip cavity leading to electrostatically attractive host-guest interactions. This seems to be an explanation of the selectivity. But the MEP calculated for thymine is also positive whereas the MEP of cytosine is negative comparable to that of adenine and guanine which disagree with the experimental result that complex AZT • 1 or AZT • 2 is relatively weak and C • 1 is as stable as U • 1. This finding indicates that in addition to the electrostatic host-guest interactions there are other binding forces which are also important for the complex stability here. In water the hydrophobic host-guest interactions have to be considered, too. They certainly contribute to the complex stability to a large extent. The strength of these binding forces is dependent from the size of the hydrophobic surfaces of the interacting molecules as it was found, for example, for the phosphonate-substituted clips having either naphthalene or anthracene sidewalls.12 The complexes of the nucleotides such as AMP, GMP, CMP, or UMP with both clips are less stable than the corresponding complexes of the nucleosides. This finding may be explained with the repulsive interaction of the negatively charged nucleotide phosphate groups with the negatively charged clip phosphate or phosphonate groups. The observed large complexation-induced 1H NMR shifts (Δδmax) of the guest signals provide good evidence that in each complex the nucleobase is encapsulated inside the clip cavity. The Δδmax values agree well with the complex structures calculated by Monte-Carlo conformer search using AMBER*/H2O or OPLS/H2O as force field.
EXPERIMENTAL
General Experimental Details: 1H-NMR titration experiments: DRX 500 (Bruker). The undeuterated amount of the solvent was used as an internal standard. The aqueous phosphate buffer at pH = 7.2 (used for the 1H NMR titration experiments) was prepared by dissolving NaOH (1.06 mmol) and KH2PO4 (1.33 mmol) in 20 mL of D2O.
The association constants Ka and the complexation-induced chemical 1H NMR shifts, Δδmax, were determined by 1H NMR titration as described in references.10 Host H and guest G are in equilibrium with the 1:1-complex HG. The association constant Ka is then defined by equation (1). [H]0 and [G]0 are the starting concentrations of host and guest, respectively.
The observed chemical shift δobs of the guest proton in the 1H NMR spectrum (Bruker instrument DRX 500, 500 MHz, 25 °C) of a host and guest mixture is an averaged value between free (δ0) and complexed guest (δHG), in the case of the exchange between free and complexed guest being fast with respect to the NMR time scale (equation (2). Combination of equations (1) and (2) and the use of differences in chemical shift (Δδobs = δ0 - δobs; Δδmax = δ0 - δHG) leads to equation (3).
Two types of titration experiments were performed: (i) in the case of Ka ≤ 5000 M-1, the total guest concentration [G]0 was kept constant, whereas the total host concentration [H]0 was varied (equation (3)); (ii) in the case of Ka > 5000 M-1, dilution titrations were performed by the use of solutions containing equimolar amounts of host and guest with concentrations in the range between 0.5 and 4 mM (equation (3)). In both experiments Δδobs was determined as the difference of the chemical shifts of the proton of pure guest (δ0) and of the guest in the various host-guest mixtures (δobs). The dependence of δobs on the host concentrations afforded the data pairs - Δδobs and [H]0 -. Fitting of these data to the 1:1 binding isotherm (equation (3) and (4) by iterative methods using the computer program TableCurve 2D, version 5.0 delivered the parameters Ka and Δδmax. Since the guest molecules (used in this work) possess more than one kind of nonequivalent protons, Ka and Δδmax were determined for the guest protons which can be unambiguously assigned over the total range of concentration and show substantial complexation-induced shifts, Δδobs. In these cases the average of the Ka values (determined independently) is given in Tables 1-3. If the guest proton signals cannot be unambiguously assigned at each concentration because they are broadened or superimposed by other NMR signals, the Δδmax values of these guest protons were calculated by the use of equation (5). The experimental error was estimated to be small for the Δδmax values (< 5%), but large for the binding constants Ka (≤ 20%), particularly in the case of the highly stable complexes for which these data has to be determined by NMR dilution titrations as described under (ii).
The detailed results of the 1H NMR titration experiments are given in the Supporting Information.
ACKNOWLEDGEMENTS
This work has been supported by the Deutsche Forschungsgemeinschaft. We thank Heinz Bandmann and Dr. Torsten Schaller for NMR measurements and Dr. Schaller also for performing the Monte-Carlo calculations of host-guest complex AZT • 1.
References
1. Review: D. Voet and J. G. Voet, ‘Biochemistry’, 3rd Edition, Wiley, New York, 2005.
2. Reviews: H. Mitsuya and S. Broder, Nature, 1987, 325, 773; CrossRef E. de Clercq, Nat. Rev. Drug Discov., 2002, 1, 13. CrossRef
3. Early examples of host molecules for nucleosides are cited by P. Cudic, M. Žinic, V. Tomišic, V. Simeon, J.-P. Vigneron, and J.-M. Lehn, J. Chem. Soc., Chem. Commun., 1995, 1073 in reference 1. CrossRef
4. J. P. Gallivan and D. A. Dougherty, J. Am. Chem. Soc., 2000, 122, 870. CrossRef
5. M. C. Rotger, J. F. González, P. Ballester, P. M. Deyà, and A. Costa, J. Org. Chem., 1994, 59, 4501; CrossRef O. Baudoin, F. Gonnet, M.-P. Teulade-Fichou, J.-P. Vigneron, J.-C. Tabet, and J.-M. Lehn, Chem. Eur. J., 1999, 5, 2762; CrossRef Y. Shi and H.J. Schneider, J. Chem. Soc., Perkin Transactions 2, 1999, 8, 1797; CrossRef M. Sirish and H.-J. Schneider, J. Am. Chem. Soc., 2000, 122, 5881; CrossRef C. Aggelidou, I. M. Mavridis, and K. Yannakopoulou, Eur. J. Org. Chem., 2009, 2299; CrossRef A. T. Zill and S. C. Zimmerman, Israel J. Chem., 2009, 49, 71. CrossRef
6. S. E. Schneider, S. N. O’Neil, and E. V. Anslyn, J. Am. Chem. Soc., 2000, 122, 542; CrossRef S. M. Butterfield and M. L. Waters, J. Am. Chem. Soc., 2003, 125, 9580; CrossRef S. M. Butterfield, M. M. Sweeney, and M. L. Waters, J. Org. Chem., 2005, 70, 1105. CrossRef
7. H. Tanaka, G. Bollot, J. Mareda, S. Litvinchuk, D.-H. Tran, N. Sakai, and S. Matile, Org. Biomol. Chem., 2007, 5, 1369. CrossRef
8. T. Schrader, M. Fokkens, F.-G. Klärner, J. Polkowska, and F. Bastkowski, J. Org. Chem., 2005, 70, 10227; CrossRef Review: F.-G Klärner and M. Campaňa Kuchenbrandt, “Synthesis of molecular tweezers and clips by the use of a molecular Lego set and their supramolecular functions” Chapter 4, pp. 99-153, in “Strategies and Tactics in Organic Synthesis”, Vol. 7 (ed. Harmata, M.), Academic Press – Elsevier, Amsterdam, 2008.
9. C. Jasper, T. Schrader, J. Panitzky, and F.-G. Klärner, Angew. Chem. Int. Ed., 2002, 41, 1355; CrossRef M. Fokkens, C. Jasper, T. Schrader, F. Koziol, C. Ochsenfeld, J. Polkowska, M. Lobert, B. Kahlert, and F.-G. Klärner, Chem. Eur. J., 2005, 11, 477. CrossRef
10. J. Polkowska, F. Bastkowski, T. Schrader, F.-G. Klärner, J. Zienau, F. Koziol, and C. Ochsenfeld, J. Phys. Org. Chem., 2009, 22, 779. CrossRef
11. M. Fokkens, F.-G. Klärner, and T. Schrader, Sensors, 2006, 6, 680. CrossRef
12. F.-G. Klärner, B. Kahlert, A. Nellesen, J. Zienau, C. Ochsenfeld, and T. Schrader, J. Am. Chem. Soc., 2006, 128, 4831. CrossRef
13. Review: F.-G. Klärner and B. Kahlert, Acc. Chem. Res., 2003, 36, 919. CrossRef
14. C. Siering, H. Kerschbaumer, M. Nieger, and S. R. Waldvogel, Org. Lett., 2006, 8, 1471 and cited references. CrossRef
15. The quantum chemical calculations were performed with the program package SPARTAN ʼ04, Wavefunction Inc., 18401 Von Karman Avenue, Suite 370, Irvine, CA 92612.
16. Substiuent effects on the EPS of aromatic compounds were described by S. E. Wheeler and K. N. Houk, J. Chem. Theory Comput. 2009, 5, 2301. CrossRef
17. Macromodel, v. 9.0, Schrödinger, Inc., 1500 SW First Ave., Ste.1180, Portland, OR 97201; F. Mohamadi, N. G. J. Richards,W. C. Guida, R. Liskamp, M. Lipton, C. Caufield, G. Chang, T. Hendrickson, and W. C. Still, J. Comput. Chem., 1990, 11, 440. CrossRef