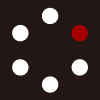
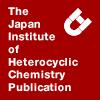
HETEROCYCLES
An International Journal for Reviews and Communications in Heterocyclic ChemistryWeb Edition ISSN: 1881-0942
Published online by The Japan Institute of Heterocyclic Chemistry
e-Journal
Full Text HTML
Received, 8th July, 2010, Accepted, 27th August, 2010, Published online, 30th August, 2010.
DOI: 10.3987/REV-10-SR(E)6
■ 4-Alkynoic Acids in the Synthesis of Biologically Important Tetrapyrroles
Peter A. Jacobi,* Harry L. Brielmann, Melanie Chiu, Indranath Ghosh, Sheila I. Hauck, Sandra Lanz, Sam Leung, Yongkai Li, Hui Liu, Franzisca Löwer, William G. O’Neal, Douglas Pippin, Elizabeth Pollina, Benjamin A. Pratt, Frédéric Robert, William P. Roberts, Carlos Tassa, and Hui Wang
6128 Burke Laboratory, Department of Chemistry, Dartmouth College, Hanover, New Hampshire 03755, U.S.A.
Abstract
In this review an account is given of the author's use of 4-alkynoic acid derivatives in the synthesis of members of the chlorin, bacteriochlorin and corrin classes of macrocyclic tetrapyrroles. In the case of chlorins, we employed a novel "2+2" condensation to prepare both C,D-symmetric and non-symmetric chlorins, made possible by the ready availability of semicorrins of type 25 derived from 4-alkynoic acids 1. Alkyne acids 1 also played a prominent role in a new 16π-electrocyclization route to bacteriochlorins, and in iterative syntheses of semicorrins and secocorrins related to vitamin B12. Mechanistic studies provided insight into the nature of these Pd(0)-catalyzed coupling/cyclization reactions. Finally, we describe enantioselective syntheses of ring-C and ring-D alkyne acids for an ongoing synthesis of cobyric acid.CONTENTS
1. INTRODUCTION
2. RESULTS AND DISCUSSION
I. Toward a General Synthesis of Chlorins
I.1 Background
I.2 Synthesis of the Key Dihydropyrrins 25
I.3 The AB + CD Route to Chlorins
II. A New Synthesis of Bacteriochlorins
II.1 Background
II.2 A 16π-Electrocyclization Route to Bacteriochlorins
III. An Iterative Synthesis of Semicorrins, Tripyrrolines and Higher Homologues
III.1 Background
III.2 Non-meso-Substituted Semicorrins and Higher Homologs
III.3 meso-Substituted Semicorrins and Higher Homologs
III.4 Semicorrins and Secocorrins Related to Vitamin B12
III.5 On the Mechanism of Pd(0)-Catalyzed Coupling/Cyclization Reactions
IV. The Alkyne Acids of Vitamin B12
IV.1 Background
IV.2 Ring C
IV.3 Ring D
3. CONCLUSIONS
4. ACKNOWLEDGEMENTS
5. REFERENCES (AND NOTES)
1. INTRODUCTION
For some time we have been exploring the synthetic utility of 4-alkynoic acid derivatives of general structure 1, which are versatile intermediates for the synthesis of diverse naturally occurring heterocycles. Much of their versatility stems from their ready accessibility, as well as the breadth of reactivity exhibited by the carbon-carbon triple bond.
In one mode of reactivity the alkyne bond in 1 is an excellent surrogate for a strategically placed carboxyl or ketone functionality. For example, Curtius rearrangement of acids 2, followed by oxidative cleavage, led smoothly to β-amino acid derivatives 3,1 which were employed in formal total syntheses of β-lactam antibiotics of the carbapenem class (Scheme 1). Similarly derived were members of the paraconic acid family of anti-fungal antibiotics,2 as well as the novel hydroxylactone derivative (+)-blastmycinone (4).3
In a second mode, acids 1 are also efficient precursors to a wide range of monocyclic enelactams 7 of the type found in naturally occurring tetrapyrroles (Scheme 2).4a This transformation can be accomplished in either of two ways, involving (1) preliminary cyclization of 1 to the corresponding enelactone 5 followed by aminolysis (path a); or (2) amidation to 6 followed by 5-exo-dig ring closure (path b). This last pathway is exemplified by the high yield conversion of homochiral acid 8, via amide 9, to enantiomerically pure enelactam 10, for which we found that n-Bu4NF (TBAF) is a particularly effective catalyst.4b
Finally, and most pertinent to the current topic, both alkyne acids 1 and amides 6 are excellent substrates for Sonogashira coupling, undergoing clean reaction with a variety of iodopyrroles and imidoyl triflates/chlorides. In the case of 1, Pd(0)-catalyzed coupling is invariably accompanied by enelactone cyclization, as illustrated for the general reaction of 1 with iodopyrrole 12 to yield 13 (Scheme 3). Aminolysis then provides the corresponding enelactams 14. Alternatively, the same products are obtained on initial coupling of 12 with alkyne amides 6, followed by TBAF-mediated cyclization.
This last methodology was highlighted in a recent paper detailing the synthesis of the linear tetrapyrrole derivative phytochrome (19),5 a key step of which involved Pd(0)-catalyzed coupling/ cyclization of homochiral 4-pentynoic acid 16 with iodopyrrole 17, followed by aminolysis (Scheme 4). After further elaboration, 18 was joined with a similarly derived C,D-ring pyrromethenone to complete the tetrapyrrole skeleton.
In the present review we describe our efforts at extending this chemistry to the synthesis of macrocyclic tetrapyrroles of the chlorin, bacteriochlorin and corrin families (Figure 1), each of which presents their own set of challenges.
2. RESULTS AND DISCUSSION
I. Toward a General Synthesis of Chlorins.
I. 1 Background. Most de novo syntheses of chlorins 21 are modeled on the methodology of Battersby6a and Montforts,6b involving either photochemical or alkali induced ring closure of properly substituted linear tetrapyrroles 20 (Scheme 5; X = OMe, Br, etc.).7 Tetrapyrroles 20 are derived from simpler ring systems employing techniques such as sulfide contraction,6b,8 thio-Wittig reaction,9 and reductive cyclization of pyrrole-substituted nitroketones.10 While elegant in concept, the cyclization of 20 to 21 can be problematic and is typically carried out on small scales, employing metal templates, and affording 21 in modest-good yields.6,8 In a noteworthy variant of this approach, Lindsey et al. have shown that condensation of fragments 22 and 23, followed by oxidative cyclization employing Zn as a template, provides Zn-chlorins 21 in up to 45% yields.11 As in the Battersby/Montforts strategies, though, a limiting factor in this approach is the availability of precursors of type 22 and 23, in particular with respect to synthesizing more highly substituted derivatives. Finally, while numerous other strategies for synthesizing chlorins have been devised, a general approach remains elusive.7
One means of addressing these issues would employ a "2+2" condensation of dihydrodipyrrins 25 with dipyrromethanes 26 (Scheme 6).12 The precursors 25 and 26 are in the proper oxidation state for direct condensation to afford chlorins 27, and macrocycle formation should be facile. Surprisingly however, this approach to chlorins had not been previously described, most likely due to difficulties in preparing A,B-ring diformyl derivatives of type 25. We set out to synthesize 25 from enelactams 24, themselves derived by aminolysis of the corresponding enelactones. The preparation of dipyrromethanes of type 26 was known to be straightforward (at least for the symmetrical case).11d,13
I. 2 Synthesis of the Key Dihydrodipyrrins 25. Method A. The requisite enelactones 35 were synthesized in good-excellent yield by Pd(0)-catalyzed coupling/cyclization between iodopyrroles 31 and alkyne acids 32, the latter either known from the literature or prepared in straightforward fashion (Scheme 7).14 The mechanism for this reaction, which is distinct from the Sonogashira protocol, presumably involves oxidative addition of Pd(0) to 31, followed by π-complexation and nucleophilic capture to give the vinyl Pd(II) species 34. Reductive elimination then provides the thermodynamic driving force for bonding the sterically crowded C4 and C5 positions. As required by this mechanism, enelactones 35 are obtained exclusively as the E-isomers under kinetic control. Once in hand, aminolysis of 35 gave high yields of the corresponding enelactams 24 as mixtures of E- and Z-isomers, dependent on the size of R1. We planned to build on 24 by substituting CHO groups at C1 and C9 (dihydrodipyrrin numbering). In principle the C9 formyl group could be introduced by initial conversion to activated imidoyl derivatives of type 36, followed by displacement with a "formyl anion" equivalent. Decarboxylative formylation at C1 in 37 would then give the desired 25 (TMOF = trimethyl orthoformate).
To evaluate this route, our initial studies were carried out with enelactam 24b, which gave a 92% yield of imidoyl triflate 36b with Tf2O and 2,6-di-tert-butyl-4-methylpyridine (Scheme 8).14d Surprisingly, though, triflate 36b was relatively unreactive toward nucleophilic displacement, returning mainly starting material with various "formyl anion" equivalents. We also investigated several Pd(0)-catalyzed formylations, all without success. Ultimately, only the Bertz reagent gave even modest yields of displacement products, affording 36% of the methyl substitution product 38b.15 We believed that 38b might be converted to the desired 37b by SeO2 oxidation. However, we were unable to isolate sufficient quantities of 38b by this route to test this idea.
Better results were obtained with the thioimidate derivatives 40 (E,Z-mixtures), which were obtained in 70-75% overall yield from lactams 24 by sulfonation with Lawesson's reagent followed by S-methylation (Scheme 9). We intended to convert both isomers of 40 to the corresponding dihydro- dipyrrins 38 by transition metal-catalyzed methylation, modeled on the elegant methodology of Liebeskind16b,c and Fukuyama.16a In practice this transformation worked well with Z-thioimidates Z-40 employing the reagent system Pd(0)/MeZnI.16d Surprisingly, however, the corresponding E-thioimidates E-40 were unreactive toward methylation using Pd(0)/MeZnI and most other commonly employed cross-coupling techniques (trace quantities of E-38 were produced with Ni(II) catalysts).
Eventually this difference was traced to a selective activating effect of Zn, which serves to polarize the thioimidate C-S bond in Z-40 by chelation (cf. Z-41 in Scheme 9).17 Control experiments showed that such activation is necessary for oxidative insertion of Pd(0). Chelation of this type is not possible for E-40, which is also sterically prohibited from simple complexation (cf. E-41; for a detailed discussion of this mechanism see Ref. 17). Consequently the desired cross-coupling reaction fails. Compounding this problem, the ratio of Z-40 : E-40 decreases with increasing size of the meso-substituent R1 (i.e. H > Me >> Ph), making it impractical to incorporate larger alkyl groups (for R1 = Ph the Z:E ratio drops to 50:50). This follows from the fact that with small R-groups the planar Z-configuration is stabilized by hydrogen bonding, but as R1 increases in size this effect becomes less important. Unfortunately, under the coupling conditions Z-40 and E-40 do not equilibrate.
With workable quantities of dihydrodipyrrins Z-38 in hand, we were next pleased to find that SeO2 oxidation produced formyl derivatives Z-37, albeit in modest overall yield (Scheme 10).18 Unexpectedly, however, we were unable to accomplish the decarboxylative formylation of Z-37 leading to Z-25, presumably due to the inductive influence of the C9-formyl group. After considerable experimentation, this last hurdle was remedied by reversing the order of decarboxylation and methylation. Thus, dihydrodipyrrins Z-40 were cleanly converted to the formyl compounds Z-42 (TFA/TMOF), which gave moderate-good yields of the desired diformyl derivatives Z-25 upon methylation followed by oxidation with SeO2 in dioxane. As will be seen later, we were also able at this point to validate the basic concept of our chlorin synthesis. However, with an unavoidable loss of material at such a late stage we set out to develop a more efficient synthesis of diformyl dihydrodipyrrins 25.
Method B - The Petasis/Tebbe Route to Dihydrodipyrrins 25. We briefly explored the possibility that enelactams 24 might be directly converted to dihydrodipyrrins 38 by methylenation followed by tautomerization (Scheme 11). A number of closely related transformations have been reported with both amides and lactams, although activation by N-substitution is usually required.19 However, in the present case both unsubstituted (Y = H) and substituted lactams 24 (Y = CO2Me, CO2t-Bu, TMS) were either unreactive or suffered slow decomposition employing either the Petasis reagent (TiCp2Me2),20 or the generally more reactive Tebbe reagent [Cp2TiCH2ClAl(CH3)].21
In contrast, enelactones 35 underwent clean condensation with TiCp2Me2, affording high yields of enol ethers 45 incorporating a range of meso-substituents R1 (Scheme 11).22 This discovery provided the basis for a very streamlined synthesis of dihydrodipyrrins 38, which were formed in "one pot" by hydrolysis of 45 to the corresponding diketones 46, followed by condensation with an appropriate ammonia source. Importantly, the Petasis reagent was compatible with commonly occurring functional groups such as propionate esters (D = CH2CH2CO2Me), as well as regioisomeric substitution patterns in ring-A (A, B = Me2, H2).
It remained now only to effect the decarboxylative formylation of dihydrodipyrrins 38 to formyl derivatives 43 to intersect with our previously established route to diformyl dihydrodipyrrins 25 (Scheme 12, cf. also Scheme 10). As in the conversion of Z-40 to Z-42 we expected this transformation to be straightforward, involving pre-treatment of 38 with TFA followed shortly thereafter with TMOF (conditions i). Again though, this seemingly well-precedented step proved to be highly capricious, affording 43 in yields ranging from 8% to 69% depending partly upon the nature of meso-substituents R1. Only with R1 = H were we able to obtain reproducible yields in the range of ~70%. In part this behavior might be due to competitive protonation at the basic pyrroline ring nitrogen in 38 to give 47, thereby inhibiting decarboxylation and/or formylation. Battersby et al. experienced similar difficulties with a closely related ring system during the course of a synthesis of bonellin.23
We explored many approaches for improving the conversion of 38 to 43, initially employing meso- phenyl substrate 38c in combination with variants of the standard protocol (TFA/TMOF, Scheme 13).24 As above, these experiments gave erratic yields of the desired formylated compound 43c accompanied by significant decomposition. In most cases the only identifiable by-product was the trifluoromethyl ketone 51 (~15%). Interestingly, we could find no trace of the presumed intermediates 49 and 50 in the crude reaction mixtures, and it is an open question whether formylation precedes or follows decarboxylation.
Battersby et al. also observed trifluoromethyl ketone formation in their studies cited above, but the origin of this compound was not investigated.23 In the case of ketone 51 two observations provided insight into this question. First, 38c gave essentially identical yields of 51 upon treatment with TFA alone, seemingly eliminating any mechanistic role for TMOF in the formation of this compound. Second, 50 prepared independently (vide infra) reacted only very slowly with TFA under conditions that led to rapid conversion of 38c to 51. Together these results suggest that a likely intermediate in the conversion of 38c to 51 is the mixed anhydride 52, which might be transformed to 51 in either inter- or intramolecular fashion. It thus seemed clear that decarboxylation of dihydrodipyrrins 38 with TFA would inevitably lead to substantial loss of starting material to this side reaction.
Eventually we found that methylpyrroline 38c underwent clean decarboxylation with HBr/HOAc, affording a 96% yield of the α-free pyrrole 50c as a mixture of Z- and E-isomers (Table 1). With decarboxylation no longer an issue we examined a number of milder conditions for effecting the desired formylation of 50c to 43c. Of these, the Vilsmeier reagent POCl3/DMF exhibited moderate promise, affording a 43% yield of 43c as the Z-isomer only.25 However, these conditions did not prove to be general. Interestingly, employing TMOF/TFA (conditions i) the yields of 43c were actually lower than those obtained by direct decarboxylative formylation of 38c to 43c (cf. Scheme 13). Mainly this was because 50c was unstable to prolonged exposure to TFA. In experiments to minimize this problem we found that 43c was obtained in 73% overall yield when a solution of crude 50c in CH2Cl2/triethyl orthoformate (TEOF) was added to pre-cooled TFA (conditions ii). These conditions provided reproducible results and were also satisfactory for preparing formyl derivatives 43d (R = C5H11, 57%) and 43e (R = C10H21, 61%). However, for substrates bearing small meso-substituents (i.e. 43a,b) even these conditions proved to be too harsh.
Method C. In parallel studies we developed a third strategy for synthesizing 43 that provided additional flexibility. Based on mechanistic considerations we expected that enelactones 35 would be good substrates for decarboxylative formylation, since in these compounds there is no pyrroline ring to compete for protonation (Method C, Scheme 14). In agreement, 35a-e routinely afforded 75-95% yields of formyl derivatives 53 with TEOF/TFA (E-isomers only). We further hoped that 53 might undergo a selective Petasis reaction at the lactone carbonyl group, as previously observed with ester-lactones 35 (cf. Scheme 11). However, all attempts at effecting this conversion produced complex mixtures. In contrast, ring opening with MeLi exhibited remarkable selectivity, affording 74-80% yields of 55a-e upon slow addition at –78 oC. At present we have no rigorous explanation for this selectivity, although it is likely that the acidic pyrrole N-H group plays a role in both protecting the formyl group and in preventing multiple addition (two equivalents of MeLi are required for complete reaction). As a working hypothesis we suggest that the C6 ketone deriving from 53 is stabilized in a chelated structure of type 54, maintaining the enolate tautomer until workup.
Diketones 55 presented a number of hurdles to cyclization, partly because of the presence of the very sensitive formyl group (Scheme 14). Formylpyrroles as a class are generally unstable to acids,26 a property that was especially pronounced with 55. These substances underwent rapid decomposition with even weakly acidic ammonium salts. In contrast, working with substrate 55b (R1 = Me) we eventually found that the combination of (NH4)2CO3/basic Al2O3 in MeCN at 75 oC gave ~75% yields of the corresponding dihydrodipyrrin 43b, a significant improvement over Method B (cf. Table 1). In analogous fashion diketone 55d (R1 = n-pentyl) afforded 43d in much improved yield (77%). Dihydrodipyrrin 43a (R1 = H), though, was still best prepared using Method B, conditions I (cf. Scheme 12), and dihydrodipyrrins 43c (R1 = Ph) and 43e (R1 = n-decyl) were best prepared using conditions ii. In Methods B and C, we thus established two complementary routes to key intermediates 43 that together are far more efficient than Method A. All of these results are summarized in Scheme 15 below.
In our initial studies we carried out the conversion of formyl dihydrodipyrrins 43a-c to diformyl derivatives 25a-c by oxidation with selenium dioxide in dioxane (cf. Scheme 10).14d While this procedure generally afforded satisfactory yields of 25a-c, the oxidation products were invariably contaminated with selenium metal that was very difficult to separate. Among other consequences this made obtaining accurate analytical data difficult. Because of this, we spent considerable time exploring other means for accomplishing the desired oxidation. Generally speaking these fell into three categories: (1) direct oxidation using powerful reagents of the Cr(VI), Co(II), Cu(II), and Pb(IV) families;27 (2) oxidation initiated by bis-halogenation (NCS, NBS, SOCl2, etc.) followed by hydrolysis;28 and (3) methyl anion generation followed by in situ capture with oxidants including diselenides, disulfides, NBS, etc.28b The results were uniformly discouraging, with some reagents exhibiting little reactivity while others caused rapid decomposition. Ultimately we returned to the SeO2 procedure, exploring the effect of reagent purity, additives, and co-oxidants. To briefly summarize, freshly sublimed SeO2 provided no apparent advantage,29 nor did so-called "wet" solvents described in the literature as having beneficial effects (in our case even trace amounts of water accelerated decomposition).30 We did, however, observe very clean reactions using dry CH2Cl2 as solvent with 1-2 equivalents of pyridine as additive (Scheme 15).30 Employing 43b (R = Me) as a substrate we consistently obtained yields of 25b in the range of 65-70% following this protocol. The one issue remaining was that ICP-MS analysis indicated that the product was still contaminated with up to 0.61% by weight (2 mol%) selenium. This last problem was solved by briefly heating the crude 25b in DMF, which produces a black metallic allotrope that can be easily removed by filtration.31 In analogous fashion diformyl derivatives 25a-e were prepared in 60-70% yield without further optimization.
I.3 The AB + CD Route to Chlorins. 1. C,D-Symmetric Chlorins.
In our first experiments the condensation of 25 and 26 was carried out in neat TFA, which was deemed necessary to effect decarboxylation of 26 to the presumably more reactive α-unsubstituted dipyrromethanes (conditions a).14d This protocol afforded 35-45% yields of a limited number of chlorins 27, illustrated below for the specific case of 27bb (Scheme 16). However, with greater quantities of 25 available, we carried out more detailed studies that revealed that our assumptions pertaining to decarboxylation were invalid.
We began our optimization studies by examining the stability of dihydrodipyrrin 25b and dipyrromethane 26b in neat TFA, now cognizant of the potential side reactions that this solvent might induce (Scheme 16). Under these conditions 25b decomposed within minutes at ambient temperature. Dipyrromethane 26b afforded moderate yields of the bis-decarboxylated product 56a along with significant quantities of trifluoroacylated derivative 56b,32 mirroring our experience with dihydrodipyrrins 38 outlined in Scheme 13. Interestingly, isolated and purified dipyrromethane 56a, for which we expected trifluoroacylation to be minimized (vide supra), gave lower yields of chlorin 27bb than dicarboxylic acid 26b under otherwise identical conditions (33% vs 42%). To explore this result further we screened numerous solvent and acid combinations with 25b and 56a, including such Lewis acids as TiCl4, BF3, and Sc(Otf)3 that have been successfully employed in similar condensations.33 In the present case these catalysts caused significant decomposition and little or no chlorin 27bb could be detected by TLC and/or UV analysis. More promising results were obtained with Brönsted acids, in particular TsOH in MeOH/CH2Cl2 (conditions b),34 although these conditions proved unreliable for larger scale syntheses of 27bb.
Based on these experiments it was clear that decarboxylation was not a prerequisite to chlorin formation, raising the possibility that dicarboxylic acid 26b might be a superior condensation partner under more refined conditions. Working from this premise we eventually identified the combination of 5% TFA in CH2Cl2 as a particularly effective medium for condensation,35 affording chlorin 27bb in a remarkably high state of purity following washing with either 3M NH4OH or saturated KHCO3 (conditions c). Final purification was by silica gel chromatography, which consistently afforded 65-75% yields of 27bb on scales up to several hundred milligrams (however, reactions were typically run on 25-50 mg scale for convenience). In analogous fashion we prepared chlorin derivatives 27aa - 27ee bearing lipophilic substituents ranging up to n-decyl at C5, along with aliphatic esters of chain length 2-10 at C13 and C17 (Table 2). Yields employing conditions c ranged from 47-85% and are shown in bold. Where applicable yields employing conditions a and b are also included.
2. Non-Symmetric Chlorins.
To this point our synthetic objectives were simplified by the fact that it was unnecessary to differentiate either the formyl groups in 25 or the α-pyrrole positions in dipyrromethanes 26 (cf. Table 2). However, while versatile in many aspects, the methodology summarized above is unsuited to the broader goal of synthesizing non-symmetric chlorins. This requires regioselective control over the four reacting carbon centers in 25 and 26. To address this issue we explored a number of variations of our "2+2" condensation strategy that incorporate the desired level of selectivity, three of which are described below (Routes 1-3). For ease of comparison, each route is illustrated by the synthesis of the same chlorin 57b.
a. Route 1 to Non-Symmetric Chlorins.
In Route 1, the regio-differentiating step involves mild acid-catalyzed condensation of A,B-ring precursor 37b with the readily available C,D-ring precursor 58 (Scheme 17). Our plan was that seco-chlorins 59 would afford chlorin 57b upon decarboxylative formylation, with the stipulation that mono-formylation is followed rapidly by cyclodehydration as opposed to oligomerization. As precedent for this approach, porphyrins have been prepared by a similar method in fair yields,36 although in these examples post-cyclization oxidation was required to give the aromatic macrocycles. In the analogous cyclization of 59, the aromatic chlorin would be obtained directly upon cyclodehydration, with the same advantages as described above for our syntheses of C,D-symmetric chlorins. In practice this approach worked moderately well, and could be carried out in a single flask without isolation of intermediate 59 or its mono-formylation product (not shown). Thus, a solution of 37b and 58 in 5%TFA/CH2Cl2 was stirred at ambient temperature under Ar for a period of 5 h, when TLC
analysis indicated that formation of 59 was complete (in separate experiments 59 was isolated and characterized in 68% yield). The resulting deep purple reaction was then adjusted to a concentration of 20% TFA/CH2Cl2 by addition of neat TFA, followed by treatment with excess trimethyl orthoformate (TMOF). After stirring an additional 16 h at rt, chlorin 57b was isolated in 34% overall yield by straightforward concentration and chromatography. The substitution pattern of 57b was unequivocally established by NOE studies, as well as by direct comparison with its subsequently prepared regioisomer (vide infra).
To test the generality and effectiveness of Route 1 we synthesized two additional unsymmetrical chlorins 57a and 57c, together with three C,D-ring symmetric chlorins 27ab, 27bb and 27cb previously prepared as described above (cf. also Table 2). As can be seen, there was little effect of non-meso-substituents on the course of these syntheses.
However, the nature of the A,B-ring meso-substituents R1 had a strong influence on reaction efficiency, with chlorins 27ab and 57a (R1 = H) being formed in considerably lower yield than the corresponding meso-substituted derivatives (R1 = Me, Ph). These results are consistent with a reaction mechanism in which carbocation character at C5 is stabilized by electron donating groups. Interestingly, Route 1 proved to be less efficient than our original methodology for synthesizing C,D-ring symmetric chlorins of type 27, yields in each case being significantly lower (cf. Table 2 for comparison).
b. Route 2 to Non-Symmetric Chlorins.
Our synthetic design for Route 2 is similar in concept to Route 1 except the aldehyde destined to become C-10 is incorporated early on. To test this approach the aldehyde-acid 61 was synthesized from the known dipyrromethane 60,37 by a two step sequence consisting of decarboxylative formylation followed by benzyl ester hydrogenolysis (Scheme 18). Also prepared from 60 was the regioisomeric C,D-ring precursor 62, by the straightforward three step sequence indicated.38
The next step called for mild acid-catalyzed condensation of 61 with the A,B-ring precursor 37b, followed by t-butyl ester hydrolysis and cyclodehydration (Scheme 19). Each of these steps had close precedent in our previous work, although self-condensation of 61 was a potential complication. Blank experiments, however, indicated that side reactions of this nature could be controlled. In practice, condensation of 37b and 61 in 5% TFA/CH2Cl2, followed by adjustment to 25% TFA/CH2Cl2, afforded chlorin 57b in 28% yield, with no evidence for by-products arising from self-condensation. In analogous fashion, condensation of 37b with dipyrromethane 62 in 25% TFA/CH2Cl2 gave a 39% yield of the regioisomeric chlorin 64b (Scheme 19). Direct comparison of chlorins 57b and 64b, along with detailed NMR analysis, then demonstrated conclusively that there had been no crossover in regiochemical control.
During the condensation of 37b and 61 a polar intermediate was observable by TLC, whose crude NMR spectrum was consistent with the anticipated tetrapyrrole condensation product 63. However, several attempts at isolating and fully characterizing this compound were unsuccessful, making further optimization difficult. Thus, while clearly a viable means of synthesizing unsymmetrical chlorins, Route 2 had no advantages over Route 1 in terms of simplicity or effectiveness. In addition, evidence was accumulating that an even more straightforward approach to unsymmetrical chlorins was feasible employing diformyl ring-A,B precursors 25 (Route 3).
c. Route 3 to Non-Symmetric Chlorins.
In our previous chlorin studies we demonstrated that diformyl derivatives 25 are excellent A,B-ring precursors for synthesizing C,D-symmetric chlorins 27 (cf. Table 2). We had assumed, though, that these materials lacked the unambiguous differentiation at C10 and C20 (chlorin numbering) necessary to impart regioisomeric selectivity. The validity of this assumption was now called into question, based upon the substantial reactivity differences we had uncovered between pyrrole- and pyrroline-type formyl groups (vide supra). Thus, it now seemed clear that electron rich pyrroles would undergo selective condensation with the more reactive pyrroline aldehyde found on ring A in 25, as opposed to the vinylogous amide-like formyl group present in ring B. This approach was first tested with dialdehyde 25b and dipyrromethane 58, which were stirred together for 5 h at RT in 5% TFA/CH2Cl2 to effect decarboxylation at C19 and initial condensation to give 65 (Scheme 20). After stirring an additional 16 h in 25% TFA/CH2Cl2, chlorin 57b was isolated in 74% yield without any effort at optimization. Furthermore, this methodology proved to be quite general. Both C,D-ring symmetric and non-symmetric chlorins were accessible by this route, with yields ranging from 22-87% (cf. Table).
As can be seen, the C5 meso-substituent still exerted some influence on the reaction efficiency, with the trend mirroring that previously noted above. That is, meso-H derivatives 27ab, 57a, and 57d were consistently formed in lower yields than the corresponding meso-Me or meso-Ph chlorins, again suggesting the likelihood of carbocation intermediates. Importantly, the yields of all chlorins were significantly improved over those obtained by Routes 1 or 2, including the meso-H series which were brought into a preparatively useful range. Even the C,D-symmetric chlorins 27bb and 27cb were obtained in higher yield than previously (cf. Table 2), presumably due to better control over competing oligomerization.
Conclusion
In summary, we have built on our "2+2" synthesis of C,D-ring symmetric chlorins to develop three new strategies for the preparation of chlorins that are fully asymmetric in their substitution pattern. Route 3, which combines operational simplicity with moderate to high product yields, proved to be the most effective route, with reactivity differences between the two formyl groups of A,B-rings 25 imparting excellent regioselectivity. Routes 1 and 2 are also useful alternatives to Route 3 if the appropriately functionalized precursors are readily available. All three strategies generate single regioisomers of diversely substituted chlorins, and in every case the "2+2" condensation is accomplished in a simple, one-flask procedure without need for additives such as oxidizing agents or metals.14a Taken together, these methodologies provide expanded access to an array of chlorins for structure activity studies that may advance the effectiveness of photodynamic therapy and other applications.
II. A New Synthesis of Bacteriochlorins.
II. 1 Background. Compared to chlorins, the lower oxidation state of bacteriochlorins makes it difficult to design a system that can undergo cyclization directly to the aromatic tetrapyrrole. This is reflected in the fact that only a very limited number of de novo syntheses of bacteriochlorins has appeared. In 1997 Kishi et al. described the first total syntheses of members of the tolyporphin (71) class of dioxo-bacteriochlorins, a novel group of tetrapyrroles that has been shown to reverse tumor multi-drug resistance (MDR) (Scheme 21).39 The Harvard group employed an ingenious strategy for preparing 71a,b, in which the pyrrocorphin derivatives 70 were synthesized from monocyclic precursors 66-69 using Eschenmoser’s sulfide-contraction methodology.40 The bacteriochlorin oxidation state was then attained by a multi-step sequence including t-butyl ether hydrolysis, bis-retro-aldol cleavage (-2 H2C=O), and auto-oxidation. Further oxidation with CrO3 gave the target compounds 71 in good yield.
A second bacteriochlorin synthesis was developed by Lindsey et al., who in 2005 described the formation of three tetrapyrrole products on self-condensation of dihydrodipyrrin 72 with BF3·Et2O in MeCN (Scheme 22).41a Careful analysis showed that two of these products were the bacteriochlorins 73a and 73b, while the third corresponded to the ring-contracted bacteriochlorin 74 (a tetradehydrocorrin). The formation of 73b was a matter of some mechanistic interest, since it requires an in situ reduction starting from dihydrodipyrrin 72. While the authors noted that "Neither the source of the reductant nor the nature of the intermediate that undergoes reduction is known," careful optimization produced yields of 73-74 in the range of 30-66% on small scales (49% for 73b). The strength of this approach is that it utilizes simple starting materials and proceeds under mild conditions. However, it is unclear whether this methodology can be extended to the synthesis of unsymmetrical bacteriochlorins.
II. 2 A 16π-Electrocyclization Route to Bacteriochlorins.
We were intrigued with the possibility that 16π-electron tetrapyrroles of type 75 might undergo a symmetry-allowed ring closure to afford bacteriochlorins 76 (Scheme 23, following page). However, it seemed unlikely that reactive species of type 75 would be stable enough to permit isolation, but rather would have to be generated in situ. One solution came in the form of an observation made during our early efforts to synthesize diformyl dihydrodipyrrin derivatives of type 25. Thus, we initially planned to prepare 25 by decarboxylative formylation of t-butyl esters 37, but were surprised to find that the intermediate carboxylic acids 77 were relatively stable (bottom, Scheme 23).
With hindsight this result is understandable, since electron deficient pyrroles undergo decarboxylation only with great difficulty. Consequently, the possibility existed that precursors to a 16π-electron system might be generated concomitantly in solution, each in a form that limits self-condensation. A plausible mechanistic pathway is illustrated in Scheme 24, where chemoselective condensation of 37 and 78 generates the intermediate tetrapyrrole 79. Acid-catalyzed elimination of HOAc would then generate the 16π-electron intermediate 80, which on electrocyclization, followed by loss of CO2 and tautomerization, would afford the bacteriochlorin 83.
This concept was first tested employing the formyl-dihydrodipyrrin 37b and acetate derivative 78b, the latter prepared in good yield by low temperature reduction of 37b with Super-Hydride followed by quenching with acetic anhydride (Scheme 25). Screening of reaction conditions by UV spectroscopy was carried out employing a variety of solvents and acid catalysts. Of these, either neat TFA or the combination of TFA/CH2Cl2 appeared to be most effective, with the reaction exhibiting a characteristic bacteriochlorin spectrum almost immediately upon mixing at RT. On closer examination, though, it was clear that at least two pigments had been formed. One of these had NMR and UV data fully consistent with the anticipated bacteriochlorin 83bb. The other (minor) product, while not fully characterized, displayed a UV spectrum that was remarkably similar to that of Lindsey's tetradehydrocorrin 74 (cf. Scheme 22). On this basis, and also NMR data, we have tentatively assigned structure 84bb to this compound, which would be a reasonable by-product from cyclization of intermediate 79 in Scheme 24.
While this result was gratifying, the possibility existed that bacteriochlorin 83bb was forming via a reaction pathway involving self condensation of 37b, which would correspond to the Lindsey methodology outlined in Scheme 22.41 However, two experiments ruled out this possibility. In the first of these, self-condensation of 37b was attempted under identical conditions to those employed in Scheme 25. While trace amounts of bacteriochlorin 83bb could be detected by TLC, the amounts formed were far too small for this to be a major reaction pathway. Secondly, we carried out the mixed condensation of dihydrodipyrrin 37c and acetate derivative 78b (Scheme 26). Only a single bacteriochlorin product was observed, whose 1H NMR and UV spectra allowed us to unambiguously assign the structure 83bc.
In particular we could find no trace of the 37c self-condensation product 83cc, which for comparison was prepared on small scale by condensation of dihydrodipyrrins 78c and 37c in neat TFA (Scheme 27).
Finally, on preparative scales the condensation of dihydrodipyrrins 78b,c and 37b,c was best carried out in 25% TFA/CH2Cl2, which appeared to minimize the formation of by-products 84. Typical yields ranged from 20-40%, which will undoubtedly improve with further optimization. As a caveat, we should note that we have thus far been unsuccessful at effecting this condensation with the meso-H substituted dihydrodipyrrins 37a and 78a (R1, R2 = H in Scheme 24). This reactivity pattern follows the same general trend observed in our chlorin syntheses (vide supra) and is consistent with a carbocation reaction pathway leading to intermediate 80 (cf. Scheme 24). As a strong point, the described methodology proceeds in a single flask under straightforward conditions, and does not involve redox chemistry or the use of a metal template. Also, to the best of our knowledge it is the only bacteriochlorin synthesis in which the starting materials are in the proper oxidation state to lead directly to the aromatic macrocycle.41b As such it should provide access to a variety of synthetic bacteriochlorins, including derivatives that are unsymmetrically substituted at C5 and C15.
III. An Iterative Synthesis of Semicorrins, Tripyrrolines and Higher Homologues.
III. 1 Background. The most complex of the naturally occurring tetrapyrroles are members of the corrin class, which incorporate up to ten chiral centers within the macrocycle (cf. 89 in Scheme 28).42 Not surprisingly, these compounds also present the greatest synthetic challenge.
In 1969 Eschenmoser et al. published an entirely new synthesis of corrins,43 providing in the process a dramatic illustration of the power of the recently discovered Woodward-Hoffmann rules.44 This strategy is outlined in Scheme 28 for the general case of corrin 89. Thus, rings A-D (84-87) were assembled mainly employing sulfide contraction methodology40 to afford the secocorrin derivative 88, which was maintained in the all Z-geometry by complexation with an appropriate metal M. Secocorrin 88 then underwent a symmetry-allowed, antarafacial 1,16-hydrogen transfer upon exposure to visible light, and the resultant intermediate, upon cycloisomerization, afforded corrin 89 in excellent yield and with over 95% stereo- selectivity. This strategy remains the "gold standard" to the present day and its implementation was an extraordinary achievement.
The versatility of this strategy is limited only by the availability of the corresponding monocyclic intermediates 84-87, and the fact that the sulfide contraction procedure is not well suited for introducing meso-substituents. In the following sections we describe our efforts at developing new methodology to supplement the sulfide contraction procedure, and its utility in synthesizing semicorrins, tripyrrolines and higher homologues.
III. 2 Non-meso-Substituted Semicorrins and Higher Homologues.
In Scheme 2 we described the facile conversion of alkyne acids 1 to enelactams 7, employing either of two efficient pathways (vide supra). We hoped that cyclizations of this type might be employed in an iterative fashion to prepare semicorrins of general structure 92 (Scheme 29). To accomplish this goal, alkyne acids 1 would first be converted to imidoyl chlorides or triflates 90 by a four step sequence consisting of (1) Pd(II)-catalyzed cyclization; (2) aminolysis of the resultant enelactone to give lactam 7; (3) enamide protection (KCN);45 and (4) lactam activation employing either CCl4/PPh3 (X = Cl) or Tf2O/imidazole (X = OTf). Imidoyl derivatives 90 would then be transformed to semicorrins 92 by Pd(0)-catalyzed coupling with alkyne amides 91, followed by 5-exo-dig ring closure. For comparison, we also outline in Scheme 29 the sulfide contraction methodology leading to the same target.
In many instances these two approaches will likely complement each other. However, a potential advantage of the proposed route is the ready accessibility of alkyne amides of type 91. In addition, the Pd(0)-catalyzed coupling of alkynes to imidoyl halides is relatively insensitive to steric hindrance (vide infra).
This concept was first tested with the enelactam derivative 97, itself derived by 5-exo-dig cyclization of the alkyne acid 96,46 followed by aminolysis (87%).47 Enamide 97 was then readily converted to the imidoyl chloride 98 by initial protection with KCN (90%),45 followed by chlorination using Ph3P/CCl4 (85%).48 Finally, we were pleased to find that Sonogashira coupling of 98 with the homochiral alkyne amide 99 afforded a 70% yield of the pyrrolinoalkyne 100,49,4 which underwent clean cyclization with TBAF to afford the semicorrin 101 (72%; epimers at C2).
We next studied the coupling of the imidoyl chloride 98 with the alkyne amide 102, which was accomplished in 89% yield using the reagent system Pd(0)/CuI. The resultant pyrrolinoalkyne 103 was then cleanly converted to the Z-semicorrin 104 upon treatment with TBAF in THF (96% yield). Both of these transformations were effected at rt and under essentially neutral conditions. Furthermore, repetition of this sequence of enamide activation, alkyne coupling, and cyclization led directly to the Z,Z-tripyrroline 106,50a which had identical physical and spectral properties as the material previously reported by Eschenmoser in his model studies for the synthesis of cobyric acid.43,50b
It was important to explore the effect of adjacent substituents on both the coupling and cyclization steps. Steric crowding turned out not to be a problem, as demonstrated for the case of imidoyl triflate 107 and alkyne amide 108 (Scheme 32). Once again, Sonogashira coupling of 107 with 108 afforded an excellent yield of the corresponding pyrrolinoalkyne 109, which underwent cyclization at rt with TBAF to give a 96% yield of the Z-semicorrin 110. Chlorination of 110 with Ph3P/CCl4 then gave an 81% yield of the imidoyl chloride 111. Interestingly, Sonogashira coupling of 111 with 108 led directly to the iminolactone 112 (Y = O, Z = NH), which was isolated in 46% yield as the Z,Z-isomer. This divergence in reaction pathway might be due to complexation of Cu with the A,B-rings of 111, since concomitant coupling and cyclization of terminal alkynes is not observed with non-chelating substrates. In the event, acid catalyzed Dimroth rearrangement of 112 with TsOH/H2O/CHCl3 then gave a 65% yield of the desired tripyrroline 113 (Y = NH, Z = O) as an inseparable mixture of E- and Z-isomers.
Finally, we have evaluated both imidoyl chlorides and triflates as substrates for Pd(0)-catalyzed coupling. Once in hand these species generally serve equally well for terminal alkyne acids and amides (cf. 107, above). However, triflate formation is occasionally complicated by competing reaction at nitrogen. For example, treatment of Z-semicorrin 110 with Tf2O/2,6-di-t-butyl-4-methylpyridine led directly to the N,O-ditriflate derivative 114, obtained exclusively as the E-isomer due to steric repulsion (Scheme 33). Diversions of this type are not observed in imidoyl chloride formation, since the reagent system Ph3P/CCl4 reacts exclusively at the enamide carbonyl (cf. 110 → 111, above). Interestingly, bis-triflation did not forestall subsequent elaboration. Thus, Sonogashira coupling of 114 with the acetylenic amide 108, followed by 5-exo-dig cyclization, gave an excellent yield of the corresponding tripyrroline 115, whose E,Z-geometry was confirmed by X-ray analysis (hydrogen bond between rings B and C).52 Repetition of this sequence of activation, coupling, and cyclization then afforded the secocorphin derivative 117. In 117, the E,E,Z-geometry derives from hydrogen bonding between rings C and D, and steric repulsion between rings A-C.
In conclusion, we have described an iterative process for the synthesis of semicorrins and higher analogs that complements the sulfide contraction methodology and in some cases might prove advantageous. In the next section we describe the extension of this methodology to the incorporation of meso-substituents, which required only slight modification.
III. 3 meso-Substituted Semicorrins and Higher Homologues.
Vitamin B12 (118) is a member of the corrin class of hydroporphyrins,42 which incorporate a vinylogous amidine chromophore within a tetrapyrroline skeleton (Figure 2). Members of this class have been the subject of extensive studies, not only because of their biological importance, but also due to their intriguing biosynthetic pathway.9b,53 Recently this area has seen a resurgence of interest from synthetic chemists, mostly directed toward the degradation product cobyric acid (119).4,54,67 This is because 119 is a known synthetic precursor to the more complex naturally occurring 118.
Two of the greatest challenges in this area are the synthesis of the A, B, C and D-ring precursors in enantiomerically pure form, and the introduction of the meso-methyl groups at C5,15. In most cases, the amidine building blocks for corrins have been prepared by a combination of iminoether condensations and sulfide contraction steps, both of which were pioneered by Eschenmoser in his extraordinary synthesis of vitamin B12.55 This methodology can be very effective, as summarized for the hypothetical coupling of enamide derivatives 120 and lactam derivatives 121a (R = H) to give semicorrins 122 (Scheme 34). However, the sulfide contraction procedure is highly sensitive to steric hindrance, and it is generally unsuited to synthesizing meso-substituted semicorrins of type 123 (vide infra).56
The cross-coupling methodology outlined in Scheme 7 was readily extended to the synthesis of meso-substituted semicorrins.57 For example, Pd(0)-catalyzed coupling of imidoyl chloride 98a with alkyne acid 124a afforded a 91% yield of the enelactone 125a, which was obtained as a 1:1 mixture of E- and Z-isomers (Scheme 35). In this case intramolecular hydrogen bonding is not possible, and the E:Z-ratio reflects steric interactions. Ring opening of 125a with NH3/dioxane then gave a 97% yield of the ketoamide 126a, which upon dehydration was cleanly converted to the desired semicorrin 127a (P2O5, 77%). Not surprisingly, 127a was obtained exclusively as the Z-isomer due to internal hydrogen bonding. In identical fashion, imidoyl chloride 98b underwent coupling with 124a to afford
a 77% yield of the enelactone 125b, which because of strong steric crowding exists predominantly in the E-configura- tion (E:Z = 10:1). Once again, however, aminolysis followed by dehydration provided only the Z-semicorrin 127b, in 85% overall yield.
It was important to test the compatibility of this methodology with steric hindrance in ring-B, which is a critical issue in the synthesis of semicorrins related to cobyric acid (119). This question was explored using the alkyne acid 124b, which gave a 69% yield of enelactone 128 on Pd(0)-catalyzed coupling/cyclization with 98a (Scheme 36). Aminolysis and subsequent dehydration then afforded semicorrin 130 in 92% yield. In contrast, all attempts at preparing 130 employing the sulfide contraction procedure failed, returning only the starting materials 131 and 132.
Finally, the iterative capability of this strategy was demonstrated by converting 130 to the imidoyl chloride 133, which was cleanly accomplished using CCl4/Ph3P (72%) (Scheme 37). Sonogashira coupling of 133 with the alkyne amide 102 then led directly to the iminolactone derivative 134 (69%), via a reaction pathway that most likely involves chelation of Cu (vide supra). As described previously (cf. Scheme 32), acid-catalyzed Dimroth rearrangement of 134 then gave an 83% yield of the Z,Z-tripyrroline 135, which has a similar substitution pattern to that found in rings A-C in cobyric acid (119).57
Further iterations of this process are possible, to produce tetrapyrroles and higher homologs. However, it is sometimes preferable to employ a more convergent approach. For example, for a synthesis of the di-meso-substituted secocorphin 138, we envisioned linking semicorrins 136 and 137, for which the sulfide contraction methodology is well suited (Scheme 38). Partly this is because the bridging meso-carbon (C10) is unsubstituted, and therefore steric hindrance is less pronounced. Of equal importance, metal chelation can be utilized to facilitate tetrapyrrole formation.
The required coupling partners 136 and 137 were derived from enamide 130 following the routes indicated. For 136 this involved straightforward thiolactamization with P4S10 (82%). Vinyl iodide 137 was obtained from 130 by initial base-induced elimination of HCN (57%), followed by iodination with I2/K2CO3 (95%). Coupling of 136 and 137 was then effected using DBU, which gave an 83% yield of an intermediate vinyl sulfide after stirring 2 h in MeCN at rt. Finally, sulfide contraction to produce secocorphin 138 was accomplished in 69% yield,57 using identical conditions to those employed by Eschenmoser et al. for synthesizing the non-meso-substituted analog of 138 (Ni[ClO4]2/Ph3P).58
The ability to incorporate meso-substituents in secocorphins of type 138 provides a useful new tool in tetrapyrrole synthesis. In the section that follows we describe the application of similar methodology to the synthesis of hexahydrodipyrrins (H6-dipyrrins) and secocorrins, which are in the proper oxidation state for cyclization to corrins.
III. 4 Semicorrins and Secocorrins Related to Vitamin B12
In Section III.3 we described a convergent synthesis of secocorphin 138 that took advantage of the ready availability of semicorrin 130 (cf. Scheme 38).50a,57 In principle, reduction of ring D in 138 could provide secocorrin 139 having both the proper oxidation state and substitution pattern for cyclization to corrin 140 (Scheme 39). In practice, though, transformations of this type have proven difficult to achieve.
We therefore investigated two strategies for preparing CD-ring semicorrins where ring D is in the pyrroline oxidation state.
Our initial approach is outlined in Scheme 40 and takes advantage of the nucleophilic character of alkyne amines 142 in Pd(0)–catalyzed coupling/cyclization reactions.59 Thus, oxidative addition of Pd(0) to imidoyl derivatives 141 would be followed by π-complexation, and then nucleophilic capture to generate vinyl–Pd(II) species of type 144 (Pd ligands have not been shown for clarity). Finally, reductive elimination would afford CD-ring hexahydrodipyrrins 145, presumably as the E-isomers (retention of configuration).60 However, E-145 should undergo rapid equilibration to the more stable Z-145, which has an internal hydrogen bond. As previously noted, the thermodynamic driving force for expulsion of Pd(0) in 144 should overcome any steric crowding in the transition state leading to 145.
Alkyne amines 142a-e were prepared by LAH reduction of the corresponding amides 146, and were used to evaluate the effects of steric hindrance and alkyne substitution pattern on reactivity (Scheme 41).61 Coupling experiments were then conducted with the imidoyl triflate 147, which turned out to
be far more reactive than the corresponding imidoyl chloride in couplings with alkyne amines (Scheme 42). The mechanistic rationale for this observation will be presented later. For the present, we were pleased to find that Pd(0)–catalyzed coupling/cyclization of 147 with alkyne amines 142 was readily effected, affording 70-85% yields of the corresponding (H6)-dipyrrins 148. For example, 147 combined at RT with terminal alkynes 142b and 142e (R = H), using the reagent system Pd(Ph3P)4/NEt3/THF (80% and 85% yields, respectively). Similarly, reaction of alkyne amine 142a (R = Me) with 147 gave a 70% yield of the meso-substituted (H6)-dipyrrin 148a. This last conversion required warming to 80 oC in MeCN and was accelerated by added BnNEt3Cl.60 Also, the most effective catalyst was the Pd2dba3/TFP system described by Farina.62
The results obtained with the isomeric imidoyl triflate 149 were less straightforward (Scheme 43). In 149 the geminal methyl groups at C2 are well removed from the reacting center at C4 and therefore provide little steric shielding. Consequently, direct nucleophilic displacement at C4 competes effectively with the Pd(0)-catalyzed process. In the best case, coupling of 149 with the alkyne amine 142a gave a 52% yield of the meso-substituted (H6)-dipyrrin 150a, accompanied by 37% of amidine 151a, the product of amine displacement. In analogous fashion, the more sterically hindered alkyne 142c afforded only 31% of the desired (H6)-dipyrrin 150c, and 62% of amidine 151c. Finally, alkyne amine 142d, which contains the most shielded triple bond and the least hindered amine, gave amidine 151d as the exclusive product (70% yield). To summarize this approach, steric crowding at C4 has little or no effect on the desired Pd(0)-catalyzed coupling/cyclization. In fact, it can be beneficial in retarding undesired side reactions (cf. Scheme 42). However, shielding of the alkyne bond by both terminal and adjacent methyl substituents has a pronounced effect on selectivity, and generally favors direct attack by the amine.
In principle, reduction of semicorrin 110 could provide an alternate route to the (H6)-dipyrrin 148b previously synthesized as described in Scheme 41 (Scheme 44). If successful, this approach might then be applied to the synthesis of less accessible (H6)-dipyrrins of type 150. Surprisingly, there appear to be no reports describing the reduction of semicorrins of type 110 to 1,2,3,7,8,9-hexahydrodipyrrins, and in fact this transformation proved to be very challenging. Eventually, though, we found that the desired reduction of imidoyl chloride 152 could be effected in 75% yield utilizing Super-Hydride (LiEt3BH) in THF at –78 oC. In identical fashion, semicorrin 130 gave the corresponding imidoyl chloride 153 (72%), which on reduction afforded (H6)-dipyrrin 150d in 53% yield.
Having in hand both semicorrin 130 and (H6)-dipyrrin 150c, we employed Eschenmoser’s methodology to synthesize the meso-substituted secocorrin 154 (Scheme 45).58 This required the initial preparation of thiolactam 136 and dipyrrin derivative 154, both of which were readily obtained following literature precedent.58,61 Oxidative coupling of 136 with 154 was then accomplished in 46% yield using NIS in the presence of DBU. Finally, treatment of the resulting vinyl sulfide with PPh3 and CdCl2 led directly to the secocorrin 154 (52%), which is in the correct oxidation state for photochemical cycloisomerization.61
III. 5 On the Mechanism of Pd(0)-Catalyzed Coupling/Cyclization Reactions63
In Section III.2 we noted that terminal alkyne acids undergo Pd(0)-catalyzed coupling/cyclization with both imidoyl chlorides and triflates with little difference in rate. Moreover, the same is true for terminal alkyne amines. These reactions are carried out under Sonogashira conditions [Pd(0)/CuI], where the reactive species are alkynyl cuprates.49 Accordingly, the most likely mechanistic pathway follows the sequence of oxidative addition, then transmetallation and reductive elimination. However, this pathway is not available for internal alkyne substrates, where substrate-dependent rate differences are common. For example, imidoyl chlorides 90a are much more reactive than triflates 90b in Pd(0)-catalyzed coupling/cyclizations with internal alkyne acids 1 (cf. Scheme 46, below). Conversely, imidoyl triflates 90b are better substrates for coupling/cyclization with internal alkyne amines 142 (vide infra). Clearly different mechanisms operate in the Pd(0)-catalyzed coupling/cyclization of internal alkyne acids 1 and alkyne amines 142.
The reactivity pattern of acids 1 is best explained on the basis of the relative Pd(II)-ligand bond strengths of RCO2- > Cl- > TfO-. This is illustrated in Scheme 46, employing imidoyl chloride 90a (X = Cl) and imidoyl triflate 90b (X = OTf) as substrates. In both cases, Pd(0)-catalyzed coupling/cyclization reactions were carried out under weakly alkaline conditions (base = NEt3), in which the alkyne acid 1 is substantially ionized. Starting with 90b, syn-oxidative addition leads initially to the square planar Pd(II) complex 156b, which is likely in equilibrium with the cationic species 157.64 However, a dissociative mechanism is not required. As pointed out by Arcadi et al.,60 carboxylate anions readily displace the labile triflate ligand from Pd(II) complexes. The resultant σ-Pd(II)-carboxylate complex 158 is then effectively removed from the catalytic cycle, due to the strength of the Pd(II)-O(C=O)R bond. Consequently little or no enelactone 155 is formed.
In contrast, under identical conditions the imidoyl chloride 90a rapidly affords the desired lactones 155. This change in reaction pathway is most likely due to the greater stability of the Pd-Cl bond in 156a,64a which is inert to both heterolytic cleavage (156a → 157) and direct anionic substitution (156a → 158). Instead, π-complexation takes place by dissociation of a neutral ligand L to form the alkyne complex 159.60,64 Ligand substitution is then followed by nucleophilic capture, affording the vinylpalladium intermediate 161 either by direct displacement of Cl- (159 → 161),64 or via an anionic, associative process (159 → 160 → 161).65 In either case σ-bond formation is facilitated by the high nucleophilicity of the participating carboxylate anion. Finally, cis-reductive elimination leads initially to the E-enelactone 155,64 which subsequently undergoes equilibration to the observed E,Z-mixture (bond isomerizations have been omitted for clarity).
A similar analysis applies to the Pd(0)-catalyzed coupling/cyclization of alkyne amines 142, which is sluggish when effected with imidoyl chlorides 90a, but rapid with triflates 90b (Scheme 47). This is the opposite chemoselectivity to that exhibited with alkyne acids 1, and presumably reflects the greatly reduced nucleophilicity of the alkyne amine π-complex 163, as compared to the alkyne carboxylate π-complex 159 (cf. Scheme 46). Thus, the amino alkyne group in 163 is slow to substitute Cl- by either direct displacement (163 → 165), or by an associative process (163 → 164 → 165).
Lastly we consider the coupling of imidoyl triflates 90b and alkyne amines 142, which is important for the synthesis of (H6)-dipyrrins 162 (Scheme 47). As described above, this transformation was much faster than that observed with chlorides 90a, and afforded mixtures of dipyrrins 162 and the corresponding amidines obtained by direct displacement.61 We considered the possibility that the displacement products were derived by a Pd(0)-catalyzed process.66 However, control experiments demonstrated that TfO- substitution occurred by direct nucleophilic displacement, and was not dependent on Pd(0). The more favorable Pd(0)-catalyzed coupling of triflates 90b and alkyne amines 142 is consistent with a mechanism in which syn-oxidative addition of Pd(0) affords the square planar complex 156b, followed by dissociation of the labile TfO- ligand to give cation 157. An identical sequence was postulated for steps 1-2 in the attempted coupling/cyclization of alkyne acids 1 and imidoyl triflates 90b (cf. Scheme 46). At this point, however, the reaction pathways diverge. With carboxylate anions 1, ion-pair bonding with cation 157 affords the neutral Pd(II)-σ-bond complex 158, a favorable, albeit non-productive step (Scheme 46).60 In contrast, primary alkyne amines 142 are much less likely to undergo Pd(II)-σ-bond formation under these conditions. This type of association typically requires concomitant deprotonation by strong base (LiNR2, NaOR, etc.), or additional activating functionality (SnNR2).66 Therefore, in the present case π-complexation of 142 with 157 is more favorable (Scheme 47), and affords the cationic species 166 which is highly activated toward nucleophilic capture (166 → 165).64 The resultant vinylpalladium species 165 then undergoes cis-reductive elimination to give the (H6)-dipyrrins 162, which undergo facile equilibration to afford the observed thermodynamically more stable Z-isomers (For a more detailed discussion of these mechanisms, including solvent and ligand effects, see reference 65).
IV. The Alkyne Acids of Vitamin B12.
IV. 1 Background. In Section III we described iterative syntheses of semicorrins, tripyrrolines and higher homologues, in which the pyrroline and lactam rings are derived from suitably functionalized alkyne acids. For the purpose of synthesizing cobyric acid (119), and thence vitamin B12 (118), we require access to alkyne acids of type 167 - 170, which would be employed in analogous fashion to construct the corrin nucleus (Scheme 48).67 Simplifying this goal is the fact that 167 - 170 share a number of features that could facilitate their preparation from a common precursor. Thus, each has a C3 quaternary center and at least one of these substituents is methyl. Also, in 167, 168, and 170 the orientation of the acetate and propionate groups is syn (although in 170 the regio- and absolute stereochemistry are reversed). Finally, acids 167 and 168 are identical except for the C5 alkyne substituent (H vs Me). Assembly of these acids as described above would produce the secocorrin derivative 171, which is properly functionalized for photochemical ring closure to produce a corrin precursor to 119.
IV. 2 Ring C
To accomplish these syntheses we are investigating variants of the Ireland–Claisen rearrangement, a powerful method for synthesizing 4-pentenoic acid derivatives.68 In principle the alkyne oxidation level found in 167-169 can be attained by incorporating a leaving group “X” in allylic esters of general structure 172 (Scheme 49). Following 3,3-sigmatropic rearrangement to 174, elimination of HX would provide the desired alkyne acid 175.
The stereochemical outcome depicted in 175 has strong precedent. Diastereoselectivity in this transformation is controlled by both enolate- and double-bond geometry, with the stipulation that reaction occurs through the most stable chair conformation. As indicated, the desired syn-selectivity would be obtained from the Z-enolate-Z-alkene configuration of 173. Control of absolute stereochemistry is also precedented and might be accomplished in either of two ways. When R ≠ H, C3 is a chiral center (*) that can be introduced in enantioselective fashion (substrate control). Alternatively, with R = H facial selectivity might be achieved using a chiral Lewis acid (M*-Br; reagent control). Corey et al. have reported promising results in this area employing the boron reagent 176.69
Our initial experiments were carried out employing reagent control and were targeted toward the ring-C precursor 169 (cf. Scheme 48).67a However, these studies were only partly successful (Scheme 50). Thus, a range of allylic esters 177 (X = H) and 178 (X = Br) were subjected to Ireland–Claisen rearrangement employing the Corey reagent 176.69 Of the eight substrates examined only 177a (R = CH2OTPDPS) and 177b (R = CH2OBn) gave useful quantities of 4-pentenoic acids, affording 179a,b in 35-50% yields and ~85% ee. Unfortunately the bromo derivatives 178 were completely unreactive, thereby precluding their use in preparing alkyne acid 169. This reactivity difference most likely derives from a combination of steric crowding and in some cases competing complexation with reagent 176 (i.e. when R = CO2Me, CN).
In principle, substrate control offers a more practical means to control both enantio- and diastereoselectivity by avoiding the use of bulky and/or rigid chiral Lewis acids. Model studies showed this to be the case, working with the readily available racemic ester 181 (Scheme 51).67b Thus, following literature precedent,68 181 was treated with 1.1 equiv each of LiHMDS and TBSCl (tert-butyldimethylsilyl chloride) in THF/HMPA at –78 oC. Upon warming to rt the resultant ketene silyl enolate 182 underwent smooth rearrangement, affording a 74% yield of alkene acid 183 after 1 h at 25 oC. Interestingly, 183 was accompanied by varying amounts of alkyne acid 184, which increased to 71% after standing 96 h. Alternatively, 184 was obtained in 97% yield upon brief exposure of 183 to DBU (5 min). To better understand this remarkably facile elimination we prepared the alkene acid
185, which differs from 183 only in the substitution of a CH3 group for TMS. Compound 185 was inert to DBU at RT, and elimination of HBr was only partly complete after heating at 85 oC for 8 h with NaH/DME. Clearly the TMS group plays an important role in facilitating the conversion of 183 to 184.
We believe the enhanced reactivity of 183 can be traced to two factors: The structural rigidity imposed by the vinyl bond and the ability of Si to stabilize both α-anions (the α-effect) and β-cations (the β-effect) (Figure 3).70 For the general case of 187 → 189, C-H bond cleavage is assisted by "negative hyperconjugation," utilizing the low-lying Si-alkyl σ*-orbitals to stabilize developing negative charge. Concomitantly, the vinyl-Si bond is held coplanar to the breaking C-Br bond, where the β-effect is most pronounced (hyperconjugation). These interactions should significantly stabilize the E2-like transition state 188 leading to anti-periplanar elimination of HBr. It is worth noting that simultaneous activation of this type is not feasible for silyl alkyl bromides 190, since coplanarity of the Br, H, and Si-bonds is impossible (cf. conformer 190a). These species normally react via desilylbromination, which occurs readily from conformation 190b.
The model studies outlined in Scheme 51 were readily extended to the synthesis of 169, which was accomplished following two independent routes (Scheme 52).67a,d The first of these involved the preparation and Ireland–Claisen rearrangement of the allylic ester (S)-192, which afforded the desired alkene acid (S)-193 via the intermediacy of a Z-silylketene acetal (cf. also Scheme 49). Silicon-assisted elimination of HBr and de-silylation then afforded 169 in ~50% overall yield from (S)-192. While workable, though, the key Ireland-Claisen rearrangement leading from (S)-192 to (S)-193 was difficult to scale and required the use of carcinogenic HMPA as an additive. To address this issue, we also explored the possibility of effecting an E-selective ester-silylenolate rearrangement on the allylic ester (R)-192 as an alternative means of establishing the 2S-configuration in (S)-193 (Scheme 52). An important advantage to this route is that E-silylenolate formation is typically carried out in non- or weakly coordinating solvents such as Et2O, eliminating the need for HMPA as an additive. In fact, this route turned out to be much preferred.67d
It is worth considering the synthesis of (R)-192 in some detail, since it proceeds through an intermediate dibromoenone E-198 that could be common to each of our syntheses of the alkyne acids of vitamin B12 (Scheme 53) Our synthesis began with commercially available 2-butyne-1-ol (194), which, following a literature procedure, was first silylated employing n-BuLi/TBSCl.71 Without purification, the resulting silyl ether 195 was subjected to in situ retro-Brook rearrangement to produce TBS alcohol 196 in 70-85% yield.71 Swern oxidation of 196 then gave an 85-95% yield of the corresponding alkynone 197. Our plan was that 197 would undergo selective anti-bromination to afford the E-dibromoenone E-198, for which there was some precedent.72 Initially, however, this reaction presented difficulties, in that under kinetic control the undesired Z-isomer predominated. For example, bromination of 197 at –78 oC (CH2Cl2/Br2) gave a ~3:5 mixture of E-198 and Z-198. In contrast, higher temperatures appeared to favor thermodynamic control, with the best results
obtained at RT and in the presence of catalytic NaI. Under these conditions the desired E-isomer predominated by >2:1, and isolated yields of E-198 ranged from 60-65% (versus 25-30% for Z-198). Moreover, nearly the identical ratio of E-198 : Z-198 was obtained upon equilibration of isolated Z-198 with BF3.Et2O at RT, raising the effective yield of E-198 to ~75% after one recycle.67c In this way we were able to conveniently prepare multigram quantities of E-198 for subsequent conversion to TBS-ketone 199, which was accomplished in 85% yield upon treatment with MeCu(CN)Li/THF. With ample quantities of 199 now in hand, we devoted considerable effort to effecting the asymmetric reduction of 199 to give (R)-200. From among many reagent combinations screened, (–)-DIP-Cl provided the most satisfactory results,73 affording 70-75% yields of alcohol (R)-200 with ee ~95%. Finally, (R)-200 underwent smooth coupling with mono-methylglutarate to afford the desired allylic ester (R)-192 in 80-85% yield.
We had now reached the point of effecting the E-selective Ireland–Claisen rearrangement of (R)-192, for which the t-butyldimethylsilyl (TBS) group served two purposes: (1) as a proton surrogate providing the chirality necessary for inducing the 2S-configuration in 169; and (2), as an anchor for stabilizing the desired chair conformation leading from (R)-192 to (S)-193 (Scheme 54). After exploring many base/solvent combinations, excellent selectivity was achieved employing a slight modification of conditions recently reported by McIntosh et al.74 Following this precedent, E-enolate formation was carried out with freshly prepared KHMDS and TBSOTf in Et2O at –78 oC. After warming to RT, and aqueous hydrolysis, alkene acid (S)-193 was obtained in 80-85% yields. The material thus obtained was identical in all respects to (S)-193 prepared from (S)-192 (cf. Scheme 52), and was produced in ~25% overall yield from 2-butyne-1-ol (194). Finally, as described previously, (S)-193 was readily converted to the ring-C alkyne acid 169 by DBU-mediated dehydrobromination followed by de-silylation. The structure of 169 was confirmed by its two-step conversion to the known cyclic enamide 204, previously employed by Eschenmoser in his synthesis of cobyric acid (169).55a
IV. 3 Ring D
While the Ireland–Claisen (I–C) methodology was readily adapted to the synthesis of 169, its application to alkyne acids of type 167, 168 and 170 provides a more stringent test. This is especially the case with 170, which in addition to containing a second, quaternary chiral center (C3), requires differentiation of the three carboxylate functionalities.
In principle, the desired stereo- and regiochemical features in 170 might be introduced employing either of the allylic ester derivatives 207 or 210, themselves derived in enantioselective fashion from enones E- and Z-205 (Scheme 55). Thus, following path a, reduction of Z-205 from the Si face would afford the homochiral allylic alcohol 206, which on coupling with mono-methyl succinate (MeSuc) would give 207. Stereoselective Z-silylenolate formation, with in situ Ireland–Claisen rearrangement, would then produce the bromoalkene acid 208 having the 2R,3R-configuration found in 170. Alternatively, the identical intermediate 208 could be derived by Re face reduction of E-205 (path b), esterification with MeSuc, and stereoselective E-silylenolate formation. Elimination of HBr would then give 170 in four steps starting with 205.
To evaluate path a, enone Z-205 was synthesized by the route outlined in Scheme 56, which provided ample material to test the crucial Ireland–Claisen rearrangement.67c This synthesis began with the readily available β-bromoenone 211,75 which on Pd-catalyzed coupling with iodozinc reagent 212 afforded a 61% yield of the corresponding E-enone 213.76 Enone 213 was then converted in 92% yield to a mixture of α-bromoenones E- and Z-205 by a one pot sequence consisting of bromination followed by dehydrobromination (DBU). Not surprisingly, this procedure gave essentially 1:1 E,Z-mixtures of 205, which, however, were readily separated by chromatography. We were then pleased to find that Z-205 underwent efficient enantioselective reduction with the reagent system (S)-CBS-Me/BH3-DMS,77a giving a 76% yield of the chiral alcohol 206 (ee 93%), whose double bond geometry was established by NOE studies. With a ready supply of 206 now in hand, the remaining steps leading to the target ring-D precursor 170 followed as planned. These involved acylation with MeSuc to give ester 207 (82%), followed by stereoselective Z-silylenolate formation. We thus obtained a 66% yield of the alkene acid 208 with dr 10:1. Finally, HBr elimination using TBAF/DMSO produced 170 in 77% yield, completing a six step synthesis from enone 211. The syn-stereochemistry of 170 was corroborated by conversion to the corresponding enelactone (not shown), in this case prepared by CuI catalyzed ring closure of bromoalkene acid 208 to avoid competitive 6-membered ring lactone formation.77b NOE studies then permitted unambiguous assignment of the relative stereochemistry as shown.
Following path a we were routinely able to prepare alkyne acid 170 with high enantio- and diastereoselectivity, and it is likely that further improvements can be made. However, we were also interested in synthesizing the desmethyl analog 214 (Figure 4), in which case the C15 meso-methyl substituent would be added at a later stage. This approach was particularly attractive given the ease of synthesizing the starting dibromoenone E-198 (cf. Scheme 53).
Our synthesis of 214 began with the Pd-catalyzed cross-coupling of E-198 with the alkylzinc reagent 212, which afforded a 79% yield of the desired E-bromoenone 215 (Scheme 57). The next step called for the asymmetric reduction of 215 to the (S)-allylic alcohol 216, which proved to be a greater challenge than with the related methyl derivative Z-205 (cf. Scheme 56). Most likely this was because of a less definitive "size" difference in the substituents attached to the ketone, and also the bromoalkene in TBS-ketone E-198 is probably the "smaller" of the two ketone substituents. This represents a reversal of the ordering found in the methyl ketone Z-205. Working from this premise, our initial reduction studies on 215 were carried out with the same (S)-CBS-Me/BH3-DMS combination employed in the reduction of Z-205 to R-206, with the expectation that the S-enantiomer 216 would be favored. This turned out to be the case, as verified by careful inspection of the corresponding Mosher esters.78 However, the ee for this transformation was only 27%. Ultimately, the combination of (S)-CBS-Bu and catechol borane was found to give much better results,79 affording 216 in 62% yield and ee 91%. Alcohol 216 then gave a 90% yield of the allylic ester 217 on coupling with MeSuc.
The remaining hurdle was in developing reliable conditions for effecting the E-silylenolate Ireland–Claisen rearrangement of 217. Once again, these were modeled on the conditions of McIntosh et al., employing KHMDS/TBSOTf in Et2O (–78 oC → rt). We thus obtained an 84% yield of the rearrangement product 218, incorporating an additional TBS group alpha to the nitrile (structure proof by X-ray analysis).80 This was of little consequence, however, since both silyl groups were cleanly removed on treatment with TBAF in DMSO, giving a 90% yield of ring-D precursor 214 with dr 11:1. As with alkyne acids 169 and 170 above, the structure of 214 was confirmed by cyclization to the corresponding enelactone (not shown), which was subjected to detailed NOE analysis.
The described six step route leading from enone E-198 to alkyne acid 214 proceeds with excellent enantio- and diastereoselectivity, and provides efficient access to this important ring-D precursor for cobyric acid (119). Moreover, we believe that E-198 could serve as a common precursor to each of the pyrroline rings of 119, and thence vitamin B12.
3. CONCLUSIONS
We hope to have made the case that alkyne acids 1 are versatile intermediates for the synthesis of a variety of biologically important tetrapyrroles, including hydroporphyrins of the chlorin, bacteriochlorin and corrin families. In particular the chlorin methodology is quite robust and has formed the basis of numerous undergraduate and Ph. D. theses. The bacteriochlorin syntheses, while less developed, offer a fresh approach and the promise of further improvement. Finally, in the corrin area, much work remains before these studies will achieve their goal of a total synthesis of vitamin B12. However, we are encouraged by the results thus far and look forward to making additional contributions.
4. ACKNOWLEDGEMENTS
Financial support of this work by the National Institutes of Health, NIGMS Grant No. GM38913, is gratefully acknowledged. I would also like to thank the dedicated undergraduate, graduate and postdoctoral students who made this work possible. They are listed as co-authors and I sincerely regret any inadvertent omissions. Finally, I gratefully acknowledge many stimulating conversations and the warm encouragement of Professor Doctor Albert Eschenmoser, ETH-Z, who in addition to being a great chemist is also an exemplary gentleman.
References
1. (a) P. A. Jacobi, S. Murphree, F. Rupprecht, and W. Zheng, J. Org. Chem., 1996, 61, 2413; CrossRef (b) P. A. Jacobi and W. Zheng in Enantioselective Synthesis of ß-Amino Acids, Eusebio Juaristi, Ed., VCH Publishers, Inc., New York, 1997.
2. (a) P. A. Jacobi and P. Herradura, J. Can. Chem. Soc., 2001, 1727; (b) P. A. Jacobi and P. Herradura, Tetrahedron Lett., 1996, 37, 8297. CrossRef
3. P. A. Jacobi and P. Herradura, Tetrahedron Lett., 1997, 38, 6621. CrossRef
4. (a) P. A. Jacobi, H. L. Brielmann, and S. I. Hauck, Tetrahedron Lett., 1995, 36, 1193; CrossRef (b) P. A. Jacobi, H. L. Brielmann, and S. I. Hauck, J. Org. Chem., 1996, 61, 5013. CrossRef
5. P. A. Jacobi, I. M. A. Odeh, S. C. Buddhu, G. Cai, S. Rajeswari, D. Fry, W. Zheng, R. D. W. DeSimone, J. Guo, L. D. Coutts, S. I. Hauck, S. H. Leung, I. Ghosh, and D. Pippin, Synlett., 2005, 19, 2861. CrossRef
6. (a) A. R. Battersby, C. J. Dutton, C. J. R. Fookes, and S. P. D. Turner, J. Chem. Soc., Perkin Trans. 1, 1988, 1557; CrossRef (b) F.-P. Montforts, Angew. Chem., Int. Ed. Engl., 1981, 20, 778; CrossRef (c) F.-P. Montforts, B. Gerlach, and F. Höper, Chem. Rev., 1994, 94, 327; CrossRef (d) F.-P. Montforts and M. Glasenapp-Breiling, Prog. Heterocycl. Chem., 1998, 10, 1. CrossRef
7. For other representative synthetic approaches to the chlorin macrocycle see: (a) D. H. Burns, Y. H. Li, D. C. Shi, and T. M. Caldwell, J. Org. Chem., 2002, 67, 4536; CrossRef (b) D. T. Gryko and M. Galęzowski, Org. Lett., 2005, 7, 1749. CrossRef
8. F.-P. Montforts and U. M. Schwartz, Liebigs Ann. Chem., 1991, 609.
9. (a) A. R. Battersby, S. P. D. Turner, M. H. Block, Z.-C. Sheng, and S. C. Zimmerman, J Chem. Soc., Perkin Trans. 1, 1988, 1577; CrossRef (b) D. M. Arnott, P. J. Harrison, G. B. Henderson, Z.-C. Sheng, F. J. Leeper, and A. R. Battersby, J. Chem. Soc., Perkin Trans. 1, 1989, 265. CrossRef
10. (a) P. J. Harrison, Z.-C. Sheng, C. J. R. Fookes, and A. R. Battersby, J Chem. Soc., Perkin Trans. 1, 1987, 1667; CrossRef (b) A. R. Battersby, C. J. Cutton, C. J. R. Fookes, and S. P. D. Turner, J Chem. Soc., Perkin Trans. 1, 1988, 1557; CrossRef (c) J.-P. Strachan, D. F. O’Shea, T. Balasubramanian, and J. S. Lindsey, J. Org. Chem., 2000, 65, 3160; CrossRef (d) T. Balasubramanian, J.-P. Strachan, P. D. Boyle, and J. S. Lindsey, J. Org. Chem, 2000, 65, 7919; CrossRef (e) M. Taniguchi, D. Ra, G. Mo, T. Balasubramanian, and J. S. Lindsey, J. Org. Chem., 2001, 66, 7342; CrossRef (f) M. Ptaszek, J. Bhaumik H.-J. Kim, M. Taniguchi, and J. S. Lindsey, Org. Process Res. Dev., 2005, 9, 651. CrossRef
11. (a) J. K. Laha, C. Muthiah, M Taniguchi, B. E. McDowell, M. Ptaszek, and J. S. Lindsey, J. Org. Chem., 2006, 71, 4092, and references cited therein; CrossRef (b) M. Taniguchi, M. Ptaszek, B. E. McDowell, P. D. Boyle, and J. S. Lindsey, Tetrahedron, 2007, 63, 3850; CrossRef (c) M. Taniguchi, D. Ra, G. Mo, T. Balasubramanian, and J. S. Lindsey, J. Org. Chem., 2001, 66, 7342; CrossRef (d) M. Ptaszek, B. E. McDowell, M. Taniguchi, H.-J. Kim, and J. S. Lindsey, Tetrahedron, 2007, 63, 3826; CrossRef (e) M. Taniguchi, M. N. Kim, D. Ra, and J. S. Lindsey, J. Org. Chem., 2005, 70, 275. CrossRef
12. G. P. Arsenault, E. Bullock, and S. F. MacDonald, J. Am. Chem. Soc., 1960, 82, 4384. CrossRef
13. See, for example, (a) K. Okada, K. Saburi, K. Nomura, K., and H. Tanino, Tetrahedron Lett., 1998, 39, 2365; CrossRef (b) B. J. Littler, M. A. Miller, C.-H. Hung, R. W. Wagner, D. F. O'Shea, P. D. Boyle, and J. S. Lindsey, J. Org. Chem., 1999, 64, 1391. CrossRef
14. (a) W. G. O'Neal and P. A. Jacobi, J. Am. Chem. Soc., 2008, 130, 1102; CrossRef (b) W. G. O'Neal, W. P. Roberts, I. Ghosh, H. Wang, and P. A. Jacobi, J. Org. Chem., 2006, 71, 3472; CrossRef (c) W. G. O'Neal, W. P. Roberts, I. Ghosh, and P. A. Jacobi, J. Org. Chem., 2005, 70, 7243; CrossRef (d) P. A. Jacobi, I. Ghosh, S. Lanz, and D. Pippin, Org. Lett., 2001, 3, 831. CrossRef
15. S. H. Bertz, M. Eriksson, G. Miao, and J. Snyder, J. Am. Chem. Soc., 1996, 118, 10906. The methyl transfer reagent was prepared following the procedure given for the corresponding n-butyl species. CrossRef
16. (a) H. Tokuyama, S. Yokoshima, T. Yamashita, and T. Fukuyama, Tetrahedron Lett., 1998, 39, 3189; CrossRef (b) J. Srogl, W. Liu, D. Marshall, and L. S. Liebeskind, J. Am. Chem. Soc., 1999, 121, 9949; CrossRef (c) C. Savarin, J. Srogl, and L. S. Liebeskind, Org. Lett., 2000, 2, 3229; We gratefully acknowledge stimulating discussions with Professor Liebeskind on this subject; CrossRef (d) For best results the MeZnI reagent must be prepared following the procedure of Fukuyama et al. (Ref. 16a). The reagent derived by treatment of MeMgCl with ZnI was considerably less reactive.
17. I. Ghosh and P. A. Jacobi, J. Org. Chem., 2002, 67, 9304. CrossRef
18. A. Werner, A. Sánchez-Migallón, A. Fruchier, J. Elguero, C. Fernández-Castaño, and C. Foces-Foces, Tetrahedron, 1995, 51, 4779. CrossRef
19. (a) N. A. Petasis and S.-P. Lu, Tetrahedron Lett., 1995, 36, 2393; CrossRef (b) C. Herdeis and E. Heller, Tetrahedron: Asymmetry, 1993, 4, 2085. CrossRef
20. N. A. Petasis and E. I. Bzowej, J. Am. Chem. Soc., 1990, 112, 6392. CrossRef
21. (a) S. H. Pine, R. J. Pettit, G. D. Geib, S. G. Cruz, C. H. Gallego, T. Tijerina, and R. D. Pine, J. Org. Chem., 1985, 50, 1212; CrossRef (b) F. N. Tebbe, G. W. Parshall, and G. S. Reddy, J. Am. Chem. Soc., 1978, 100, 3611. CrossRef
22. (a) J. F. Payack, D. L. Hughes, D. Cai, I. F. Cottrell, and T. R. Verhoeven, Org. Syn., 2002, 79, 19; (b) J. F. Payack, M. A. Huffman, D. Cai, D. L. Hughes, P. C. Collins, B. K. Johnson, I. F. Cottrell, and L. D. Tuma, Org. Proc. Res. Dev., 2004, 8, 256. CrossRef
23. A. R. Battersby, C. J. Dutton, and C. J. R. Fookes, J Chem. Soc., Perkin Trans. 1, 1988, 1569. CrossRef
24. P. S. Clezy, C. J. R. Fookes, and A. J. Liepa, Aust. J. Chem., 1972, 25, 1979. CrossRef
25. U. T. Mueller-Westerhoff and G. F. Swiegers, Synth. Commun., 1994, 24, 1389. CrossRef
26. A. H. Jackson, in Pyrroles; R. A. Jones, Ed.; The Chemistry of Heterocyclic Compounds; John Wiley & Sons: New York, 1990; Vol. 48, Part 1. p 316.
27. (a) Co(II): J. A. R. Salvador and J. H. Clark, Chem. Commun., 2001, 33; CrossRef (b) Cu(I): J. A. R. Salvador, M. L. Sá e Melo, and A. S. C. Neves, Tetrahedron Lett., 1997, 38, 119; CrossRef (c) Cu(I): E. S. Arsenou, A. I. Koutsourea, M. A. Fousteris, and S. S. Nikolaropoulos, Steroids, 2003, 68, 407; CrossRef (d) Cu(II): G. Rothenburg, L. Feldberg, H. Wiener, and Y. Sasson, J. Chem. Soc., Perkin Trans. 2, 1998, 2429; CrossRef (e) Pd/TBHP: J-Q. Yu and E. J. Corey, Org. Lett., 2002, 4, 2727; CrossRef (f) Pd(OAc)2/PhI(OAc)2: A. R. Dick, K. L. Hull, and M. S. Sanford, J. Am. Chem. Soc., 2004, 126, 2300; CrossRef (g) (SePh)2/PhIO2: D. H. R. Barton and D. Crich, Tetrahedron, 1985, 41, 4359; CrossRef (h) t-BuI/FeCl2/DMSO: E. Vismara, F. Fontana and F. Minisci, Gazz. Chim. Ital., 1987, 117, 135.
28. (a) CuCl2/LiCl: J. A. Nobrega, S. M. C. Gonçalves, and C. Peppe, Synth. Commun., 2002, 32, 3711; CrossRef (b) NCS/NBS/SO2Cl2: K. A. Tehrani, D. Borremans, and N. De Kimpe, Tetrahedron, 1999, 55, 4133. CrossRef
29. H. Kaplan, J. Am. Chem. Soc., 1941, 63, 2654. CrossRef
30. E. N. Trachtenberg, in Oxidation; R. L. Augustine, Ed.; Marcel Dekker: New York, 1969; p 19.
31. S. R. Milstein and E. A. Coats, Aldrichimica Acta, 1978, 11, 10.
32. H. Xie, D. A. Lee, D. M. Wallace, M. O. Senge, and K. M. Smith, J. Org. Chem., 1996, 61, 8508. CrossRef
33. G. R. Geier, III, J. B. Callinan, P. D. Rao, and J. S. Lindsey, J. Porphyr. Phthalocya., 2001, 5, 810. CrossRef
34. (a) D. H. Burns, Y. H. Li, D. C. Shi, and T. M. Caldwell, J. Org. Chem., 2002, 67, 4536; CrossRef (b) L. T. Nguyen, M. O. Senge, and K. M. Smith, J. Org. Chem., 1996, 61, 998. CrossRef
35. For use of TFA/CH2Cl2 in a related context see: (a) T. D. Lash, J. Porphyr. Phthalocya., 1997, 1, 29; CrossRef (b) T. D. Lash, Chem. Eur. J., 1996, 2, 1197. CrossRef
36. (a) W. Li and T. D. Lash, Tetrahedron Lett., 1998, 39, 8571; CrossRef (b) B. Zhang and T. D. Lash, Tetrahedron Lett., 2003, 44, 7253. CrossRef
37. R. K. Pandey, I. N. Rezzano, and K. M. Smith, J. Chem. Res., Miniprint, 1987, 8, 2171.
38. R. Chong, P. S. Clezy, A. J. Liepa, and A. W. Nichol, Aust. J. Chem., 1969, 22, 229. CrossRef
39. T. G. Minehan and Y. Kishi, Tetrahedron Lett., 1997, 38, 6811. CrossRef
40. A. Eschenmoser, Pure Appl. Chem. Suppl., 1971, 2, 69.
41. (a) H.-J. Kim and J. S. Lindsey, J. Org. Chem., 2005, 70, 5475; CrossRef (b) W. G. O'Neal, Ph. D. thesis, Dartmouth College, Hanover, New Hampshire, 2007.
42. F.-P. Montforts, B. Gerlach, and F. Höper, Chem. Rev., 1994, 94, 327, and references cited therein. CrossRef
43. Y. Yamada, D. Miljkovic, P. Wehrli, B. Golding, P. Löliger, R. Keese, K. Müller, and A. Eschenmoser, Angew. Chem., Int. Ed. Engl., 1969, 8, 343. CrossRef
44. R. B. Woodward and R. Hoffmann, The Conservation of Orbital Symmetry, Verlag Chemie, GmbH, Weinheim/Berstr., Germany, 1970.
45. H. Liu, Ph. D. thesis, Wesleyan University, Middletown, Connecticut, 1999.
46. See, for example: A. Arcadi, A. Burini, S. Cacchi, M. Delmastro, F. Marinelli, and B. R. Pietrono, J. Org. Chem., 1992, 57, 976, and references cited therein. CrossRef
47. See, for example: J. Micklefield, R. L. Mackman, C. J. Aucken, M. Beckmann, M. H. Block, F. J. Leeper, and A. R. Battersby, J. Chem. Soc. Chem. Commun., 1993, 275, and references cited therein. CrossRef
48. R. Appel, Angew. Chem., Int. Ed. Engl., 1975, 14, 801. CrossRef
49. K. Sonogashira, Y. Tohda, and N. Hagihara, Tetrahedron Lett., 1975, 16, 4467. CrossRef
50. (a) P. A. Jacobi and H. Liu, J. Am. Chem. Soc., 1999, 121, 1958; CrossRef (b) We are grateful to Professor Doctor Albert Eschenmoser, ETH-Z, for providing us with experimental details and spectral data for several unpublished procedures.
51. A. R. Katritzky and J. M. Lagowski, in Comprehensive Heterocyclic Chemistry; A. R. Katritzky and C. W. Rees, Ed.; Pergamon Press Inc.: New York, 1984, Vol. 5, p 94.
52. We are grateful to Dr. Victor G. Young, X-ray Crystallographic Laboratory, Department of Chemistry, University of Minnesota, for carrying out this analysis.
53. (a) A. R. Battersby, Science, 1994, 264, 1551, and references cited therein; CrossRef (b) A. Eschenmoser, Angew. Chem., Int. Ed. Engl., 1988, 27, 5, and references cited therein. CrossRef
54. (a) D. Riether and J. Mulzer, Euro. J. Org. Chem., 2003, 30, and references therein; CrossRef (b) R. V. Stevens, N. Beaulieu, W. H. Chan, A. R. Daniewski, T. Takeda, A. Waldner, P. G. Williard, and U. Zutter, J. Am. Chem. Soc., 1986, 108, 1039. CrossRef
55. (a) A. Eschenmoser and C. E. Wintner, Science, 1977, 196, 1410, and references cited therein; CrossRef See also, (b) K. C. Nicolaou and E. J. Sorensen, Classics in Total Synthesis; VCH: Weinheim, Germany, 1996, p 99.
56. (a) J. E. Bishop, J. F. O'Connell, and H. Rapoport, J. Org. Chem., 1991, 56, 5079; CrossRef (b) A. Eschenmoser, Pure Appl. Chem. Suppl., 1971, 2, 69.
57. P. A. Jacobi and H. Liu, J. Org. Chem., 1999, 64, 1778. CrossRef
58. E. Götschi, W. Hunkeler, H.-J. Wild, P. Schneider, W. Fuhrer, J. Gleason, and A. Eschenmoser, Angew. Chem., Int. Ed. Engl., 1973, 12, 910. CrossRef
59. A. Arcadi, S. Cacchi, and F. Marinelli, Tetrahedron Lett., 1989, 30, 2581. CrossRef
60. A. Arcadi, A. Burini, S. Cacchi, M. Delmastro, F. Marinelli, and B. R. Pietrono, J. Org. Chem., 1992, 57, 976, and references cited therein. CrossRef
61. P. A. Jacobi and H. Liu, Org. Lett., 1999, 1, 341. CrossRef
62. V. Farina, S. R. Baker, D. A. Benigni, S. I. Hauck, and C. Sapino, Jr., J. Org. Chem., 1990, 55, 5833. CrossRef
63. P. A. Jacobi and H. Liu, J. Org. Chem., 2000, 65, 7676. CrossRef
64. (a) J. T. Link and L. E. Overman, in “Metal-catalyzed Cross-coupling Reactions”, R. Diederich and P. J. Stang, eds. Wiley-VCH, 1998, p 231; (b) W. Cabri, and I. Candiani, Acc. Chem. Res., 1995, 28, 2; CrossRef (c) G. P. Dekker, C. J. Elsevier, K. Vrieze, and P. W. van Leeuwen, Organometallics, 1992, 11, 1598; CrossRef (d) A. Madin, C. J. O'Donnell, T. Oh, D. W. Old, L. E. Overman, and M. J. Sharp, Angew. Chem. Int. Ed., 1999, 38, 2934. CrossRef
65. C. Amatore, A. Jutand, and A. Suarez, J. Am. Chem. Soc., 1993, 115, 9531. CrossRef
66. For reviews, see (a) J. F. Hartwig, Angew. Chem. Int. Ed., 1998, 37, 2046; CrossRef (b) J. P. Wolfe, S. Wagaw, J.-F. Marcoux, and S. L. Buchwald, Acc. Chem. Res., 1998, 31, 805. CrossRef
67. (a) P. A. Jacobi and Y. Li, Org. Lett., 2003, 5, 701; CrossRef (b) P. A. Jacobi and C. Tassa, Org. Lett., 2003, 5, 4879; CrossRef (c) H. Wang, C. Tassa, and P. A. Jacobi, Org. Lett., 2008, 10, 2837; CrossRef (d) H. Wang and P. A. Jacobi, ARKIVOC, 2010 (IV), 15.
68. (a) R. E. Ireland and R. H. Mueller, J. Am. Chem. Soc., 1972, 94, 5897; CrossRef (b) R. E. Ireland and M. D. Varney, J. Am. Chem. Soc., 1984, 106, 3668; CrossRef (c) R. E. Ireland, P. Wipf, and J. D. Armstrong, III, J. Org. Chem., 1991, 56, 650; CrossRef (d) G. Koch, P. Janser, G. Kottirsch, and E. Romero-Giron, Tetrahedron Lett., 2002, 43, 4837, and references therein. CrossRef
69. E. J. Corey and D. Lee, J. Am. Chem. Soc., 1991, 113, 4026. CrossRef
70. M. A. Brook, Silicon in Organic, Organometallic, and Polymer Chemistry, John Wiley & Sons, Inc., New York, New York; 2000.
71. K. Sakaguchi, M. Fujita, H. Suzuki, M. Higashino, and Y. Ohfune, Tetrahedron Lett., 2000, 41, 6589. CrossRef
72. A. G. Myers, M. M. Alauddin, M. A. M. Fuhry, P. S. Dragovich, N. S. Finney, and P. M. Harrington, Tetrahedron Lett., 1989, 30, 6997. CrossRef
73. (a) H. C. Brown, J. Chandrasekharan, and P. V. Ramachandran, J. Am. Chem. Soc., 1988, 110, 1539; CrossRef (b) P. V. Ramachandran, A. V. Teodorovic, M. V. Rangaishenvi, and H. C. Brown, J. Org. Chem., 1992, 57, 2379. CrossRef
74. C. McFarland, J. Hutchison, and M. C. McIntosh, Org. Lett., 2005, 7, 3641, and references therein. CrossRef
75. G. Buono, Synthesis, 1981, 872. CrossRef
76. T. Mandai, T. Matsumoto, J. Tsuji, and S. Saito, Tetrahedron Lett., 1993, 34, 2513. CrossRef
77. (a) E. J. Corey and C. J. Helal, Angew. Chem. Int. Ed., 1998, 37, 1986, and references therein; CrossRef (b) Modeled on the conditions of Buchwald et al. for amidation of vinyl bromides: L. Jiang, G. E. Job, A. Klapars, and S. L. Buchwald, Org. Lett., 2003, 5, 3667. In contrast to terminal alkyne acids, internal alkyne acids of type 170 give mixtures of 5- and 6-membered lactones with PdCl2. CrossRef
78. J. A. Dale and H. S. Mosher, J. Am. Chem. Soc., 1973, 95, 512, and references therein. CrossRef
79. E. J. Corey and R. Bakshi, Tetrahedron Lett., 1990, 31, 611. CrossRef
80. The structure of the predominate (S)-relative epimer was confirmed by X-ray analysis of a racemic sample. We thank the University of Massachusetts Amherst X-ray Structural Characterization Facility (NSF CHE 9974648) for providing diffractometer access and Mr. Travis Benanti of that facility for data collection and refinement.