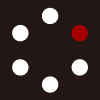
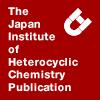
HETEROCYCLES
An International Journal for Reviews and Communications in Heterocyclic ChemistryWeb Edition ISSN: 1881-0942
Published online by The Japan Institute of Heterocyclic Chemistry
e-Journal
Full Text HTML
Received, 14th August, 2010, Accepted, 21st October, 2010, Published online, 2nd November, 2010.
DOI: 10.3987/COM-10-S(E)106
■ α,α-Dichloroisoxazolidinones for the Synthesis and Chemoselective Peptide Ligation of α-Peptide α-Ketoacids
Tetsuo Narumi and Jeffrey W. Bode*
Laboratory of Organic Chemistry, ETH Zürich, Wolfgang-Pauli-Strasse 10,
CH-8093 Zuerich, Switzerland
Abstract
In seeking to develop an iterative approach to the preparation of α-oligopeptides by the chemoselective amide-forming coupling of α-ketoacids and hydroxylamines, we have designed and synthesized novel enantiopure monomers. Key to our approach is the use of α,α-dichloroacids as masked α-ketoacids. The preparation of these monomers, their coupling with α-ketoacids, and the conversion of the α,α-dichloroacids to α-ketoacids is described. These studies provide a first step to a conceptually unique approach to peptide synthesis that does not require activating reagents or produce chemical byproducts.INTRODUCTION
The chemical and biogenic synthesis of α-amino acids relies on the activation of the carboxylic acid moiety towards coupling with an amine nucleophile.1 The success and prevalence of this paradigm has obscured the consideration of alternative approaches to iterative peptide couplings that could have advantages in chemical synthesis or a potential role in the prebiotic or exobiotic assembly of oligopeptide chains. In addressing this, we have sought to develop methods for amide bond formation that are chemically distinct from classical peptide synthesis and have recently identified the coupling of α-ketoacids and hydroxylamines as a novel, chemoselective, and water-compatible approach to amide and peptide bond formation.2
We have previously documented the application of the α-ketoacid–hydroxylamine ligation (KAHA ligation) to the iterative synthesis of β3-oligopeptides.3 These reactions proceed in water, do not require coupling reagents, and produce only CO2 and MeOH as reaction byproducts (Figure 1). Provided that suitable enantiopure monomers for the preparation of α-oligopeptides could be identified, this chemistry could offer a novel and chemically orthogonal route to iterative peptide synthesis. Importantly, such an approach could obviate the problematic and wasteful coupling reagents typically employed in large excess during classical peptide synthesis.4
The difficulty in extending our earlier work to the iterative synthesis of α-oligopeptides lies in the identification and synthesis of a suitable monomer class to serve as the building blocks for the amino acid residues. These monomers must 1) cleanly afford the peptides by decarboxylative condensations with α-ketoacids, 2) result in products that can be converted to the next α-ketoacids following the ligation, and 3) be amenable to preparation in enantiopure form on a multigram scale. Initially, we envisioned an extention of the isoxazolidine approach we applied towards the preparation of β-peptides, which would require α-ketolactone monomers 2. However, such monomers are likely to be highly prone to epimerization, as such 5-membered ring heterocycles have a tendency to exist predominantly in the conjugated enol form.5 After considering several alternatives, we elected to pursue the use of α,α-dicholoroacids as masked α-ketoacid surrogates (Figure 2). In this article, we describe our preliminary investigations into the preparation of enantiomerically enriched α,α-dichloroisoxazolidinones and their use for the iterative synthesis of α-oligopeptides. These studies establish a synthetic entry into these monomers, their viability in the key amide-forming ligation reaction, and the conversion of the resulting α,α-dichloroacids into the corresponding α-ketoacids.
RESULTS AND DISCUSSION
Our synthetic route to the enantiomerically enriched valine-derived dichloro monomer is illustrated in Scheme 1. After exploring several different cycloaddition routes, we selected a modification of Vasella’s carbohydrate-derived nitrone as a viable synthesis of monomer 8.6 Simply heating a mixture of the nitrone, prepared from isobutyl aldehyde 4 and D-Gulose-derived hydroxylamine 5,7,8 with α,α-dichloroketene silyl acetal 69 containing a stoichiometric amount of ZnCl2 provided directly the α,α-dichloroisoxazolidin-5-ones 7 in 44% yield with high regio- and diastereoselectivity, rather than the expected α,α-dichloroisoxazolidine.10,11 Removal of the chiral auxiliary by perchloric acid-mediated hydrolysis provided L-α-Val monomer 8 in 72% yield. The enantiomer D-α-Val monomer ent-8 was prepared from L-Gulose-derived hydroxylamine by a similar sequence.
Although the condensation of the nitrone and the silyl ketene acetal could also be applied to give isoxazolidinones corresponding to leucine, phenylalanine, and alanine-monomers, the removal of the chiral auxiliary proved problematic. We believe that this can be addressed with optimization, but have instead pressed on with our exploration of the chemistry of these heterocycles using the valine-derived monomers.
With α,α-dichloro-isoxazolidinone 8 in hand, the decarboxylative amide bond formation with phenylpyruvic acid (9) was examined (Table 1). Polar solvents such as DMF or DMSO are ideal solvents for the coupling of O-unsubstituted hydroxylamines.2 On the other hand, non-polar or protic solvents including aqueous media are suitable for the coupling of isoxazolidine-based monomer.3 We therefore began our studies with a screen of solvents for the amide-formation using phenylpyruvic acid (1.5 equiv) and 0.1 M monomer 8 at 40 °C for 12 h. Non-polar solvents such as CH2Cl2, toluene and THF were unproductive (entries 1-3). Polar solvents, including DMF and DMSO, gave the desired amides in low conversion along with significant amounts of unidentified products (entries 4 and 5), probably due to the decomposition of the starting material. A 1:1 mixture of tBuOH and water gave the desired amide 10 cleanly, albeit with low conversion (entry 6). Reaction with higher substrate concentration (0.2 M) gave 10 with improved conversion (entry 7), but further increases in concentration did not improve the outcome (entry 8). Additional optimization of these conditions revealed that MeOH or EtOH were superior cosolvents (entries 9–11). Alcohol solvents alone, however, did not afford amide products (entry 12).
Having identified aqueous alcohol as the preferred solvent for amide-bond formation, we performed a second level optimization of the reaction pH (Table 2). The ligations occurred over a wide pH range, with slightly acidic oxalic acid buffers offering the best results.
The key underlying concept to the proposed iterative synthesis of α-oligopeptides is the use of the α,α-dicholoro carboxylic acid as a masked α-ketoacids. Although the conversions of dihaloacids and related compounds to α-ketoacids are rare, several encouraging examples were known12 including a thorough study from a group at Bristol-Myers Squibb that implicated the formation of an α-lactone in the hydrolysis.13 It was unclear, however, if any of these methods would be compatible with the proximal amide functionality and the epimerizable α-stereocenter.
Working from the BMS precedent for conversion of α,α-dichloroacids 10 to the α-ketoacids 11, we began our investigations with base promoted hydrolysis (Table 3). Strong bases including LiOH (entry 1), K3PO4 (entry 2), and Na2CO3 (entry 3) afforded exclusively undesired oxazole 12. Success was obtained with weaker bases (entries 4–8) and several conditions afforded the α-ketoacids as the major product. Although the oxazole byproduct could not be completely excluded, acceptable yields could be obtained with potassium bases including potassium oxalate at 80 °C (entry 8).
At this juncture, we sought to address the critical questions of the stereochemical integrity of the α-stereocenter under the basic conditions necessary for the unmasking of the α-ketoacids. In our experience, which is consistent with the chemical literature,14 α-ketoacids are configurationally stable in organic solvents and in the presence of aqueous acid, but are prone to racemization in the presence of base.15 They are generally stable under the ligation conditions.
α,α-Dichloroacid 10 and its enantiomer ent-10, prepared from the L-gulose-derived chiral auxiliary, were each hydrolyzed with 1.0 M potassium oxalate at 80 °C for 3 h and the resulting α-ketoacids isolated in 48–52% yield by preparative HPLC (Scheme 2). We were not able to assay the enantiopurity of α-ketoacid 11 directly and therefore elected to perform a second ligation prior to analysis. Each enantiomer of valine-derived α-ketoacid 11 was subjected to ligation with benzylhydroxylamine 13 in aqueous DMF under unoptimized conditions and the resulting amides analyzed by SFC on a AD-H column. Unfortunately, this study revealed significant epimerization of the valine residue, presumably due to the basic conditions employed in the hydrolysis of the α,α-dichloroacid.
In preliminary work, we have prepared dichloroisoxazoline monomers corresponding to other amino acid residues including L-leucine and L-phenylalanine and found that these examples are less prone to racemization during hydrolysis, suggesting that improvements to these protocols are possible. Further efforts to improve the hydrolysis conditions and the preparation of the monomers may provide an alternative route to the iterative synthesis of α-peptides that does not require coupling reagents or protecting groups and which operates under aqueous conditions
ACKNOWLEDGMENTS
T.N. was a postdoctoral fellow supported by the Japan Society for the Promotion of Science. Additional funding for this work was generous provided fellowships to J.W.B from the Arnold and Mabel Beckman Foundation and the David and Lucille Packard Foundation.
EXPERIMENTAL
General Methods. All reactions utilizing air- or moisture-sensitive reagents were performed in dried glassware under an atmosphere of dry nitrogen. Thin layer chromatography (TLC) was performed on EMD precoated plates (silica gel 60 F254, Art 5715) and were visualized by fluorescence quenching under UV light and by staining with phosphomolybdic acid and/or p-anisaldehyde, respectively. For flash column chromatography, EMD Silica Gel 60 (230–400 Mesh) and Silica Gel 60 N (Kanto Chemical Co., Inc.) were employed. 1H NMR (500 MHz) and 13C NMR (125 MHz) were measured on a Bruker Avance AVII-500 spectrometer with a CryoProbe. Chemical shifts are expressed in parts per million (ppm) with respect to the residual solvent peak. Coupling constants are reported as Hertz (Hz), signal shapes and splitting patterns are indicated as follows: br, broad; s, singlet; d, doublet; t, triplet; q, quartet; m, multiplet. Infrared (IR) spectra were recorded on a SHIMADZU IR Prestige-21 FTIR-8400S and are reported as wavenumber (cm–1). Low- and high-resolution mass spectra were recorded on a Brucker Daltonics microTOF-2focus in the positive and negative detection mode. Optical rotations were measured on JASCO DIP-370 polarimeter operating at the sodium D line with a 100 mm path length cell, and were reported as follows: [α]DT (concentration (g:100 mL), solvent).
HPLC Conditions. For analytical HPLC, a Cosmosil 5C18-ARII column (4.6 x 250 mm, Nacalai Tesque, Inc., Kyoto, Japan) was employed with a linear gradient of MeCN containing 0.1% (v/v) TFA at a flow rate of 1 cm3 min–1 on a LaChrom Elite HTA system (Hitachi High-Technologies corporation, Ltd., Tokyo, Japan) and JASCO PU-2086 plus (JASCO corporation, Ltd., Tokyo, Japan), and eluting products were detected by UV at 220 nm. Preparative HPLC was performed using a Cosmosil 5C18-AR II column (20 x 250 mm, Nacalai Tesque, Inc.) on a JASCO PU-2087 plus and PU-2089 plus (JASCO corporation, Ltd., Tokyo, Japan) in a suitable gradient mode of MeCN solution containing 0.1% (v/v) TFA at a flow rate of 7-10 cm3 min–1.
(2,2-Dichloro-1-methoxyvinyloxy)trimethylsilane 6. To a suspension of activated Zn dust (784.8 mg, 12.0 mmol) in THF (10 mL) was added dropwise a solution of Cl3CCO2Me (0.953 mL, 8.0 mmol) and TMSCl (1.23 mL, 9.6 mmol) at ambient temperature (< 40 °C). After stirring at room temperature overnight, the reaction mixture had changed to a clear cream-colored suspension containing the excess Zn dust. This mixture was used in the next step without further purification.
(S)-4,4-Dichloro-2-((3aR,4R,6S,6aR)-6-((R)-2,2-dimethyl-1,3-dioxolan-4-yl)-2,2-dimethyltetrahydrofuro[3,4-d][1,3]dioxol-4-yl)-3-isopropylisoxazolidin-5-one 7. To a solution of D-gulose derived chiral auxiliary 116 (562.6 mg, 2.0 mmol) in CH2Cl2 (10 mL) was added isobutylaldehyde (272.4 µL, 3.0 mmol) in the presence of MgSO4 (1 g). After stirring at rt for 3 h, the reaction mixture was filtered to remove MgSO4. Concentration under reduced pressure gave the nitrone as a white solid, which was used in the next step without further purification. To a solution of the nitrone in THF (10 mL) was added a solution of α,α-dichloroketene silyl acetal 6 (0.80 M, 8.0 mmol) containing a stoichiometric amount of ZnCl2 in THF (10 mL), from which the excess Zn dust was removed with a PTFE filter (0.45 µm). After stirring at rt for 30 min, the mixture was refluxed for 6 h. After cooling to room temperature, the reaction mixture was poured into ice-cold saturated aqueous NaHCO3 and extracted with EtOAc. The extract was washed with brine and dried over MgSO4. Concentration under reduced pressure followed by flash chromatography over silica gel with hexanes-EtOAc (9:1) gave 7 (382.7 mg, 44% yield) as a colorless oil: [α]25D +3.74 (c 0.710, CHCl3); 1H NMR (500 MHz, CDCl3) δ 5.39 (s, 1H), 4.96 (d, J = 6.0 Hz, 1H), 4.77 (dd, J = 6.0, 4.0 Hz, 1H), 4.39 (dt, J = 8.5, 6.5 Hz, 1H), 4.32 (d, J = 3.0 Hz, 1H), 4.23 (dd, J = 9.0, 7.0 Hz, 1H), 4.15 (dd, J = 8.5, 4.0 Hz, 1H), 3.76 (dd, J = 8.5, 6.5 Hz, 1H), 2.43-2.34 (m, 1H), 1.46 (s, 3H), 1.39 (s, 3H), 1.38 (s, 3H), 1.31 (s, 3H), 1.06 (d, J = 7.0 Hz, 3H), 0.882 (d, J = 6.5 Hz, 3H); 13C NMR (125 MHz, CDCl3) δ 168.5, 113.4, 109.9, 97.8, 85.6, 84.1, 80.2, 78.4, 75.4, 74.4, 65.9, 31.2, 26.5, 26.0, 25.1, 24.7, 21.0, 15.7; IR (KBr) ν 2981, 1807, 1209, 1080, 979, 948, 894, 864, 848, 759 cm-1; HRMS (ESI), m/z calcd for C18H28Cl2NO7 [M+H]+ 440.1243 and 442.1213, found 440.1245 and 442.1214.
(S)-4,4-Dichloro-3-isopropylisoxazolidin-5-one 8 (L-Val monomer). To a solution of D-gulosyl L-Val monomer 7 (102.8 mg, 0.233 mmol) in MeCN (2.3 mL) was added 60% HClO4 (116.7 µL, 0.700 mmol), and the mixture was stirred at rt for 1.5 h. After being diluted with EtOAc, the mixture was washed with saturated aqueous NaHCO3 and brine, and dried over MgSO4. Concentration under reduced pressure followed by flash chromatography hexanes-EtOAc (9:1) gave 8 (33.3 mg, 72% yield) as a colorless oil: [α]25D –3.18 (c 1.27, CHCl3); 1H NMR (500 MHz, CDCl3) δ 6.70 (br, 1H), 3.69 (dd, J = 12.3, 8.9 Hz, 1H), 2.01-2.20 (br, 1H), 1.24 (d, J = 6.7 Hz, 3H), 1.24 (d, J = 6.7 Hz, 1H); 13C NMR (125 MHz, CDCl3) δ 170.0, 79.5, 76.0, 28.2, 19.0; IR (KBr) ν 3431, 3226, 1811, 1197, 759 cm-1; HRMS (ESI), m/z calcd for C6H10Cl2NO2 [M+H]+ 198.0089 and 200.0059, found 198.0082 and 200.0056.
(S)-2,2-Dichloro-4-methyl-3-(2-phenylacetamido)pentanoic acid 10. To a solution of L-Val monomer 8 (61.4 mg, 0.310 mmol) in a 1:1 mixture (1.55 mL) of MeOH/oxalic buffer (pH 6.1) was added phenylpyruvic acid (76.4 mg, 0.465 mmol). After being stirred at 40 oC for 41 h, purification by preparative HPLC (Gradient: 0 min, 30% MeCN in H2O; 90 min, 60% MeCN in H2O) followed by lyophilization afforded compound 10 (63.4 mg, 64% yield) as colorless powder: 1H NMR (500 MHz, CDCl3) δ 7.40-7.28 (m, 5H), 5.97 (d, J = 10.5 Hz, 1H), 4.82 (dd, J = 10.5, 3.5 Hz, 1H), 3.82 (d, J = 16.5, 1H), 3.74 (d, J = 16.5, 1H), 2.37-2.27 (m, 1H), 0.956 (d, J = 7.0, 3H), 0.745 (d, J = 6.5, 3H); 13C NMR (125 MHz, CDCl3) δ 173.6, 166.5, 133.4, 129.7, 129.4, 128.0, 87.1, 60.7, 43.2, 29.4, 22.0, 17.2; HRMS (ESI), m/z calcd for C14H18Cl2NO3 [M+H]+ 318.0664 and 320.0634, found 318.0653 and 320.0630.
(S)-4-Methyl-2-oxo-3-(2-phenylacetamido)pentanoic acid 11. Dichloro-isoxazolidinone 10 (55.6 mg, 0.175 mmol) was treated with 1.0 M aqueous solution of potassium oxalate (3.5 mL) at 80 °C for 3 h. After cooling to room temperature, purification by preparative HPLC (Gradient: 0 min, 25% MeCN in H2O; 90 min, 55% MeCN in H2O) followed by lyophilization afforded 11 (23.8 mg, 52% yield) as a colorless oil: 1H NMR (500 MHz, CDCl3) δ 7.41-7.27 (m, 5H), 6.14 (br, 1H), 5.03 (br, 1H), 3.69 (s, 2H), 2.30-2.23 (m, 1H), 0.924 (d, J = 7.0 Hz, 3H), 0.683 (d, J = 7.0 Hz, 3H); 13C NMR (125 MHz, CDCl3) δ 193.1, 173.3, 160.5, 133.5, 129.4, 129.3, 127.9, 60.0, 43.0, 29.6, 19.6, 17.0; IR (KBr) ν 3388, 1724, 1647, 1531, 1215, 761 cm-1; HRMS (ESI), m/z calcd for C14H18NO4 [M+H]+ 264.1236, found 264.1239.
2-Benzyl-4-isopropyloxazole-5-carboxylic acid 12. Dichloro-isoxazolidine 10 (55.6 mg, 0.175 mmol) was converted into the title compound 12 (11.2 mg, 26% yield) as colorless freeze-dried powder: 1H NMR (500 MHz, CDCl3) δ 7.34-7.27 (m, 5H), 4.17 (s, 2H), 3.67-3.51 (m, 1H), 1.27 (d, J = 6.5, 6H); 13C NMR (125 MHz, CDCl3) δ 165.6, 162.6, 157.6, 135.39, 134.4, 128.9, 128.8, 127.3, 34.8, 26.0, 21.3; IR (KBr) ν 3412, 1612, 758 cm-1; HRMS (ESI), m/z calcd for C14H16NO3 [M+H]+ 246.1130, found 246.1127. This is the side product of the previous reaction.
References
1. J. Jones, “Amino Acid and Peptide Synthesis Second edition” Oxford University Press, Inc., New York, 2002; T. Sonke, B. Kaptein and H. E. Schoemaker, “Amino Acids, Peptides and Proteins in Organic Chemistry. Vol. 1-Origins and Synthesis of Amino Acids,” ed. by A. B. Hughes, Wiley-VCH Verlag GmbH & Co. KGaA, Weinheim, 2009, pp. 79-117.
2. J. W. Bode, R. M. Fox, and K. D. Boucom, Angew. Chem. Int. Ed., 2006, 45, 1248. CrossRef
3. N. Carrillo, E. A. Davalos, J. A. Russak, and J. W. Bode, J. Am. Chem. Soc., 2006, 128, 1452. CrossRef
4. W. C. Chan, and P. D. White, “Fmoc Solid Phase Peptide Synthesis” Oxford University Press, Inc. New York, 2000.
5. For example, Vitamin C has a five-membered ring containing two-carbonyl groups but normally exists predominently as a conjugated ene-diol, see: J. Clayden, N. Greeves, S. Warren and P. Worthers, “Organic Chemistry” Oxford University Press, Inc., New York, 2001, pp. 523-545.
6. A. Vasella, Helv. Chim. Acta, 1977, 60, 1273. CrossRef
7. K. Kasahara, H. Iida, and C. Kibayashi, J. Org. Chem., 1989, 54, 2225. CrossRef
8. S. Yu, H. Ishida, M. Juarez Garcia, and J. W. Bode, Chemical Sciences, 2010, in press. (DOI:10.1039/C0SC00317D). CrossRef
9. R. Imashiro and T. Kuroda, J. Org. Chem., 2003, 68, 974. CrossRef
10. S. Jost, Y. Gimbert, and A. E. Greene, J. Org. Chem., 1997, 62, 6672. CrossRef
11. M. Lombardo and C. Trombini, Cur. Org. Chem., 2002, 6, 695. CrossRef
12. G. Barger and A. Ewins, J. Chem. Soc., Trans., 1909, 95, 552; CrossRef G. Caprara, G. Lemetre, and A. Vercellone, Ann. Chim. (Rome), 1959, 49, 1167; M. Julia and M. Baillarge, Bull. Soc. Chim. Fr., 1959, 850.
13. J. D. Godfrey, Jr., R. T. Fox, F. G. Buono, J. Z. Gougoutas, and M. F. Malley, J. Org. Chem., 2006, 71, 8647. CrossRef
14. A. J. L. Cooper, J. Z. Ginos, and A. Meister, Chem. Rev., 1983, 83, 321. CrossRef
15. L. Ju, A. R. Lippert, and J. W. Bode, J. Am. Chem. Soc., 2008, 130, 4253. CrossRef
16. N. Mita, O. Tamura, H. Ishibashi, and M. Sakamoto, Org. Lett., 2002, 4, 1111; CrossRef For an improved synthetic procedure, see reference 8.