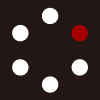
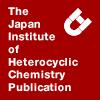
HETEROCYCLES
An International Journal for Reviews and Communications in Heterocyclic ChemistryWeb Edition ISSN: 1881-0942
Published online by The Japan Institute of Heterocyclic Chemistry
e-Journal
Full Text HTML
Received, 11th June, 2010, Accepted, 24th January, 2011, Published online, 2nd February, 2011.
DOI: 10.3987/REV-10-SR(E)2
■ Synthesis of Rhazinilam: A Comparative Review of Forty Years of Synthetic Endeavors
Inga Kholod, Olivier Vallat, Ana-Maria Buciumas, and Reinhard Neier*
Department of Chemistry, University of Neuchâtel, Avenue de Bellevaux 51, Case Postale 2, CH-2007 Neuchâtel, Switzerland
Abstract
R-(-)-Rhazinilam is a relatively simple but unusual monopyrrolic product isolated from nature. It is probable that R-(-)-rhazinilam is an artifact of the isolation procedure. Seven total syntheses of rhazinilam have been described in the literature. The comparison of the published syntheses clearly demonstrates the sensitivity of the pyrrole ring contained in the rhazinilam structure. Despite the spectacular progress in organic synthetic methods only a few synthetic strategies have been applied to the total synthesis of rhazinilam. Astonishingly the number of steps needed from commercial starting materials has stayed similar over the almost 40 year period since the first synthesis has been reported in 1973.INTRODUCTION
NATURAL PRODUCTS AS SOURCE FOR NEW DRUGS
The use of natural products for the treatment of human diseases has been documented very early in human history e.g. in the “Ebers Papyrus”, dating back to 1500 B.C.1-6 Many widely used drugs have been initially isolated from plants (Figure 1).
The capacity of chemists to isolate, to identify and to modify drug molecules has been a major driving force for the advancement of chemistry. Until the early sixties of last century, natural products chemistry was undoubtedly one of the central activities of academic and industrial laboratories (Figure 2). Natural products chemistry combined all the activities ranging from the isolation to the synthesis and testing of natural products. Often the problem posed during natural products chemistry was the motivation to develop new and more efficient methods and techniques. Natural products chemistry was undoubtedly a central activity and a field driving the progress of chemistry as a whole. The strong link between synthesis and natural products chemistry was loosened once physical and spectroscopic methods allowed identifying the structures of small quantities of products isolated from natural sources. The structure determination of Vitamin B12 by Dorothy Crowfoot Hodgkin based on X-ray crystallography was an impressive example of the power of physical methods to determine complicated structures.7-10
In this process spectroscopic methods gained in importance. Natural products chemistry on the other side lost to a large extend its unique position uniting under one single subject heading, isolation, separation techniques, characterization, identification, studies of reactivities and synthesis. The different subfields became independent and for several decades the importance attributed to natural products and their use was diminished or underestimated. Another factor which influenced the status of natural products chemistry in the canon of chemistry was the development of methods adapted to studies of very important classes of biopolymers like proteins and DNA. Starting from the seminal publication of Watson and Crick11 huge efforts were dedicated to deciphering the secrets of DNA, the essential molecule for the storage of information in living organisms.
In parallel to the studies of nucleic acids, efforts were devoted to the analysis of peptides,12 proteins and enzymes and to the determination of their sequence first and then their three-dimensional structure.13 The development of solid state synthesis by Bruce Merrifield14 opened the opportunity to make peptides on demand using automated synthesizer. During a long period the ground work had to be done by many natural products chemists, who had specialized in peptide chemistry.15 The synthetic transformations, the protecting groups and the analytics had to be optimized before the automated solid state synthesis could become a routine technique. During this period natural products chemistry obviously spread over much wider territory but it lost at the same time its central position. Especially the interest in small natural products diminished or it was overshadowed by the many other activities, which were the focus of important research projects in the field between chemistry, biology and medicine.
Recent years have seen a revival of natural products chemistry in its classical sense. Several factors have contributed to the recovery of the interest in small to medium sized natural products. The challenge of finding new effective drugs has become enormous and the financial risks have put a huge pressure on the pharmaceutical industry. Despite the use of new and efficient scientific, organisational and management methods the number of drugs approved has fallen steadily. The hopes that the increased knowledge (especially from the completion of the human genome project), coupled with more efficient methodologies (synthesis of libraries, parallel synthesis and better separation techniques) would create again higher numbers of drugs have not been fulfilled. Astonishingly in these difficult times natural products have kept their position and many new drugs coming on the market are either natural products or compounds derived from natural products16-23 (Figure 3).
This is all the more astonishing as searching for drugs from natural sources is the oldest approach to drug discovery. Therefore much hope and huge investments went into new methods, believing that modern science would make the tedious process of finding drug candidates more efficient and faster. Despite enormous efforts natural products still seem to be one of the best bets, if one wants to find a drug. It is not obvious why nature provides humans with drug molecules. Scientists analyse the natural role of small molecules with the hope to find out why this exquisite class of compounds called natural products have been such an extraordinary source of scientific knowledge and economic wealth.24-30 Interesting and important questions are raised about the functions of the collection of these natural products in their natural environment. Many of these questions cannot be answered yet.
A parallel development tries to make use of the biosynthetic machinery, especially in the field of the polyketide biosynthesis, in order to get modified active compounds and/or to find compounds, which are usually synthesized in too tiny amounts to be isolated. Based on the analysis of the genetic information, combined with the knowledge of the function of the individual modules in the biosynthetic machinery modified “natural products” have been obtained.31-33
Finding and isolating even delicate natural products has become more efficient and much easier. Thanks to the much milder and faster separation methods available, many natural products, which did not survive the classical, tedious isolation process, can now be found and identified. It is clear, that natural products have gained again the interest of the science community and the pharmaceutical industry. There is still a lot of excitement in all activities related with natural products, as long as small molecules with unusual structures are capable to act and influence important biological processes with unsurpassed selectivity and efficiency.
NATURAL PRODUCTS CONTAINING PYRROLE RINGS
Until the middle of last century a great variety of nitrogen heterocycle containing natural products have been isolated and identified. The number of monopyrrolic natural products isolated was comparatively small34 (Figure 4).
At this point in time the more complex tetrapyrrolic macrocyclic natural products had already been identified and many of their functions had been well recognized. The tetrapyrrolic “pigments of life” fulfill essential tasks for important processes like oxygen transport, electron transport, catalyzing oxidation processes, catalyzing unique transformations and most importantly playing central roles in photosynthesis. It was also well known that these macrocycles were synthesized in large quantities by nature. The pathways of the biosynthesis and the structures of the simpler precursors were however unknown. The structure of PBG was determined by Cookson and Rimington only in 1954.35 Porphobilinogen (PBG) is the dedicated pyrrolic intermediate for the biosynthesis of uroporphyrinogen III, the precursor of all tetrapyrrolic macrocycles (Figure 5A). In hindsight it is impressive to realize that almost at the same time chemists determined the structure of the universial precursor for the “pigments of life” the structure of a new class of pyrrole containing antibiotics was discovered. Only three years after the discovery of PBG the initial proposals for the structure of netropsin an oligopeptidic antibiotic isolated from Streptomyces netropsis were published36,37 (Figure 5B). It was only after intensive studies that scientists realized that this class of antibiotics acquires its activity due to the capacity to interact selectively with DNA.
Since these two important discoveries the number of monopyrrolic natural products isolated and determined has increased steadily. A systematic overview of all monopyrrolic natural products known before 2003 has been presented by Gossauer in his beautiful review article.34 The monopyrrolic natural products are incorporated in an astonishing variety of different skeletons. Most pyrrole containing natural products are decorated by a functional group stabilizing the electron rich heterocycle against oxidation and degradation. Esters, amides or lactam are directly bond to the heteroaromatic ring. Aromatic rings directly connected to the heterocycle can also stabilize the sensitive pyrrole. Only very few pyrrole containing natural products have not incorporated a stabilizing group into their structure. Pyrroles isolated from ants are a notable exception38 (Figure 6), but it is probably correct to assume that these special natural products are not produced in large quantities.
The other more remarkable exception is PBG, the second dedicated precursor of the tetrapyrrolic natural macrocycles. The amount of PBG synthesized by nature each year is huge. PBG is not accumulated during the biosynthetic process, but is synthesized in the quantities needed for the formation of the adequate quantities of the tetrapyrrolic macrocycles. PBG possesses a high reactivity, which is used for the synthesis of uroporphyrinogen III, the natural precursor of all “pigments of life”. The absence of stabilizing groups on the pyrrole ring of PBG makes sense. The enhanced reactivity is a crucial element for the next step of the biosynthesis. The electron withdrawing groups present in many monopyrrolic natural products increase the stability of these compounds and allow their isolation.
Pyrrole containing natural products have been isolated from many sources. The structural variety is impressive. The role played by the pyrrole ring for the function of these natural products can be crucial as in the case of PBG. In many cases the chemists and biologists are not yet able to attribute a precise function to the pyrrole ring within the skeleton of the natural product. The properties of the electron rich pyrrole ring and its strong hydrogen bond donor capacity bestow unique properties to this small heterocycle, which have been used by nature and which had to be taken into account, when natural products contain monopyrrolic units. It is therefore one of the challenges for chemists with an interest in this type of compounds to disclose the links between the structure of these monopyrrolic natural compounds and their properties. Still one of the best ways to reveal the connections inscribed into the structure of the natural products and to learn about the reactivity of these compounds are studies of the total synthesis.
ISOLATION AND STRUCTURE DETERMINATION OF RHAZINILAM AND ITS STRUCTURAL ANALOGUES
(R)-(-)-Rhazinilam (1) was first isolated by Linde from Melodinus australis in 1965.39 The isolation from Rhazya stricta (1970)40,41 some years later lead to the trivial name used today. The natural product has been found in other South-east Asian members of the family Apocynaceae. 42-50 The alkaloid 1 has also been obtained from somatic hybrid intergenic cell cultures.51 More recently, (R)-(-)-rhazinilam (1) was isolated from the plant species Kopsia arborea (2007).52,53
The structure of (R)-(-)-rhazinilam (1) was established in 1972 through spectroscopic analysis, chemical degradation studies41 and finally confirmed by X-ray crystallographic techniques43 (Figure 7). The complete infra red, mass spectra and 1H and 13C NMR analysis of compound 1 was reported later.54 The four rings are identified as rings A to D. The quaternary carbon center at C20 and a phenyl-pyrrole chirality axis are the two stereogenic elements of (R)-(-)-rhazinilam (1). The dihedral angle between rings A and C is almost 90°. The lactam bond is in the s-cisoid conformation. The nine membered ring adopts a boat-chair conformation imposed by the two aromatic bonds and the lactam bond contained in the medium sized ring rigidifying the ring. It was not possible to determine the absolute configuration (R, aR) by X-ray. The absolute configuration was deduced via semi-synthesis from an aspidosperma alkaloid, namely (+)-1,2-didehydroaspidospermidine (13) (Scheme 1).55
Other alkaloids with the same tetracyclic array as (R)-(-)-rhazinilam (1) have been isolated from different members of the family Apocynaceae: rhazinicine (2),60-63 3-oxo-14,15-dehydrorhazinilam (3),64 (-)-leuconolam (4),46,48,63,65,66 (+)-epi-leuconolam (5),46,48 N-methylleuconolam (6),67 (R)-(-)-rhazinal (7),63,68 kopsiyunnanines C1 (8), C2 (9), C3 (10)53 (Figure 7). The formation of all these alkaloids is postulated to occur via oxidative pathways from 5,21-dihydrorhazinilam (11), which is co-isolated with 1.46-48,52,59
POSTULATED FORMATION OF (R)-(-)-RHAZINILAM FROM INDOLE ALKALOIDS
(R)-(-)-Rhazinilam (1) is now considered to be an artifact of the extraction procedure. The unstable 5,21-dihydrorhazinilam (11)41 aromatises spontaneously on exposure to air.46,47 The dihydro-derivative 11 is believed to be the immediate natural precursor of (R)-(-)-1. More significantly the natural product 1 can also be synthesized starting from (+)-vincadifformine (12) (Scheme 1).55 The mechanism of the stepwise conversion was proposed by Smith55 and later experimentally confirmed by Guenard.56,57 The sequence starts from (+)-12 and comprises an acid-catalysed ester hydrolysis and decarboxylation followed by an oxidation-reduction-oxidation sequence. The key step in this sequence is the MCPBA-promoted oxidative cleavage of the C2-C3 indoline bond in (+)-13 producing the N-oxide 14. The N-oxide 4 was reduced with Fe(II) to give a 9 : 1 mixture of (R)-(-)-1 and (R)-11. The conversion of (R)-(11) to (R)-(-)-1 was slow. This observation suggests that the formation of 1 from N-oxide 14 occurred via a Polonovsky-type reaction.57,58 This is further circumstantial evidence for this hypothesis. (+)-1,2-Didehydroaspidospermidine (13) has never been detected in vivo together with 11 or (R)-(-)-1. If (+)-1,2-didehydroaspidospermidine (13) is the precursor of (R)-(-)-rhazinilam (1) remains an open question.
TOTAL SYNTHESES OF rac-RHAZINILAM
Four total syntheses of racemic rhazinilam (1) have been reported. The main motivation for these studies was the unusual structure of (±)-1. Over the almost forty years different strategies were applied to create the challenging elements contained in (±)-1: the direct A-C biaryl link, the nine-membered lactam B ring, and the quaternary-carbon at C20.
FIRST TOTAL SYNTHESIS OF rac-RHAZINILAM BY SMITH
The first total synthesis of the racemic rhazinilam (1) was reported by Smith and co-workers in 1973.55 Reacting ethyl magnesium bromide with diethyl 4-ketopimelate (15) gave the lactone in good yield, (Scheme 2). The resulting γ-lactone 16 was reduced in a two-step sequence: Rosenmund reduction followed by NaBH4 reduction. Tosylation of 17 with tosyl chloride in pyridine provided the desired tosyl derivative of γ-lactone 18 in 22% over six steps from commercially available 15.
The pyrrole 20 was prepared via Knorr-type condensation (Scheme 3). Vilsmeier formylation of compound 20 followed by silver oxidation and subsequent esterification with diazomethane generated the pyrrole 21 in 11% yield over four steps from 19.
The crucial combination of the two intermediates was achieved by the N-alkylation of the sodium salt of the pyrrole 21 with the tosyl derivative of the lactone 18. The intramolecular Friedel-Crafts cyclisation of the pyrrole 22 gave the tetrahydroindolizine derivative 23 in 50% yield. Catalytic reduction of the nitro group followed by lactamisation afforded compound 24. A two step sequence had to be applied to produce rac-rhazinilam (1) under harsh conditions but in good overall yield. The synthesis proceeds through ten steps starting from the commercially available acid 19 in a remarkable 3.6% overall yield.
TOTAL SYNTHESIS OF rac-RHAZINILAM BY SAMES
In 2000 a very elegant total syntheses of rac-rhazinilam (1) was reported by Sames and co-workers.70 The key intermediate 32 was synthesized in the efficient sequence depicted in Scheme 4.72,73 The Grigg-type 1,5-electrocyclisation reaction74 of 29 catalysed by silver carbonate produced the intermediate 30 in 70% yield. The sensitive pyrrole ring was then protected in two steps as methyl ester 31. Selective reduction of 31 provided the aniline 32 in 25% over seven steps from commercially available nitrile aldehyde 25. The second key step was the transformation of one of the ethyl groups into ethenyl group. The pivotal platinium complex 35 had to be synthesized, introducing a Schiff base first and treating the Schiff base 34 with the dimethyl platinum reagent [Me2Pt(µ-SMe2)]275 providing 35 in 88% yield. Treating the intermediate 35 successively with triflic acid followed by thermolysis in trifluroethanol at 70 °C and then by decomplexation with potassium cyanide and hydrolysis of the Schiff base 38 provided the racemic alkene 39 in 60% yield over four steps. The total synthesis was then completed via a formal one-carbon extension of the vinyl group and the subsequent closure of the medium sized lactam ring in six synthetic steps. Lemieux-Johnson oxidation provided the aldehyde 40 followed by Wittig olefination and selective reduction of the alkene 41 affording the racemic intermediate 42 in 70% over three steps. Reduction and lactamisation of compound 42 gave the previously reported ester 24, which was transformed to rac-1 according to Smith’s methodology.55 The synthesis proceeds through twenty steps in 3.5% overall yield starting from nitrile aldehyde 25. The sequence is considerably longer than the Smith synthesis, but the overall yield is almost identical with the yield of the first synthesis.
TOTAL SYNTHESIS OF rac-RHAZINILAM BY MAGNUS
In 2001 Magnus and co-workers published a straightforward and elegant synthesis of rac-rhazinilam (1)72 (Scheme 5).
The retrosynthetic analysis is very similar to the one used by Sames, with the difference that the two chains on the α-position of the pyrrole ring are already differentiated right from the start of the synthesis. The elegant but lengthy modification of one of the two ethyl groups can thereby be avoided, which reduces the number of steps. Starting from commercially available 2-piperidone 43 the ethyl and allyl group are sequentially introduced in a total of six synthetic operations. N-alkylation of this thiophenyl imino ether 45 with 2-nitrocinnamyl bromide 28 gave the corresponding iminium intermediate 46. This intermediate underwent a Grigg-type 1,5-electrocyclisation/thiophenol elimination reaction74 to provide compound 47 in 71% yield. The compound 47 was transformed into the natural product rac-1 in a sequence of six steps: hydroboration, Swern-type oxidation with pyridine/sulphur trioxide/dimethylsulfoxide,76 exhaustive oxidation using silver nitrate under alkaline condition, Raney nickel reduction and finally lactamisation. The reaction types used in the end game of the Sames and Magnus synthesis are very similar. It is therefore all the more surprising that the Magnus group did not need to introduce any protection on the pyrrole ring. The Magnus group could reduce considerably the number of steps. The synthesis proceeds through nine steps in an impressive 7.6% overall yield.
TOTAL SYNTHESIS OF rac-RHAZINILAM OF TRAUNER
The Trauner synthesis published in 2005 forms in the last step rac-rhazinilam (1) using an intramolecular Heck-type reaction77 starting from an intermediate 54 (Scheme 6). The difference to the Sames and Magnus synthesis is the presence of the amide bond directly connecting the A and the D rings. Trauner group starts with 2-carbomethoxy pyrrole (50) which is N-alkylated with γ-lactone tosylate 18. The alkylation and the intramolecular Friedel-Crafts cyclisation of 51 are almost identical to the Smith procedure.55 At this point the Trauner synthesis diverges from the Smith synthesis. Amide coupling introduces all the atoms of the rhazinilam skeleton. For the Heck type coupling, the amide had to be protected with the methoxymethyl (MOM) protecting group transforming compound 55, which was exposed to 10 mol % of Buchwald’s “DavePhos” ligand 5678 and Pd(OAc)2 in the presence of potassium carbonate as base to give the strained nine-membered ring 57 in 47% yield. Removing first the MOM protecting group55,70,71 followed by saponification and acid-catalysed decarboxylation produced rac-1. Only seven steps are needed starting from commercially available pyrrole 50 and an impressive 7.9% overall yield could be obtained. Starting from the commercially available diester 15, rac-rhazinilam (1) can be obtained in thirteen steps and in 1.7% overall yield.
TOTAL SYNTHESES OF R-(-)-RHAZINILAM
Besides the four syntheses of racemic rhazinilam three of (-)-enantiomer have been reported. The chirality of the A-C biaryl axis is determined by the absolute configuration of the quaternary-carbon at C20. The challenge is therefore to obtain this quaternary center in enantiomerically pure form.
SAMES’ SECOND ENANTIOSELECTIVE TOTAL SYNTHESIS OF (R)-(-)-RHAZINILAM
The Sames group adapted their synthesis of rac-rhazinilam so that they could obtain the (R)-(-)-rhazinilam.71 Sames chose to introduce a chiral auxiliary 62, so as to functionalize diastereoselectively the intermediate 63 to the product 67 (see Scheme 8). The chiral auxiliary phenyl-(5R)-cyclohexyl-2-oxazolinone 62 was prepared in three steps from commercially available mandelonitrile 58 in 32 % overall yield (Scheme 7).
The desymmetrisation of the two enantiotopic ethyl groups in compound 32 was achieved by metal-induced C(sp3)-H activation via the six steps sequence leading to the alkene 39 (96% ee) (Scheme 8). After decomplexation of the platinum with potassium cyanide a mixture of the diastereomers of 67 were obtained with a de of 63-77%. In this sequence the diastereomers were separated by preparative HPLC to give after removal of the ligand the alkene (R)-39. The palladium-catalysed carbonylation of the alkene (R)-39 gave directly in 58% yield the required nine-membered lactam (R)-24 which had been reported55,70 previously in its racemic form. This metal catalysed transformation replaced favourably the five step sequence used in the synthesis of the racemate. The enantiomerically pure (R)-(-)-rhazinilam (1) was finally obtained in 90% yield and 96% ee following the procedure described by Smith.55 The synthesis proceeds through fifteen steps starting from nitrile aldehyde 25 in 1.6% overall yield.
TOTAL SYNTHESIS OF (R)-(-)-RHAZINILAM BY NELSON
In 2006 Nelson and co-workers reported an elegant, enantioselective total synthesis of (R)-(-)-rhazinilam (1) (Scheme 9).79 The enantioselective synthesis of the almost enantiopure β-lactone 69 via a cyclocondenstion80-82 using a quinine derived asymmetric catalyst installed the two chiral centers83 with high selectivity. From the β-lactone 69 the enantioenriched allene 72 was obtained by ring opening using the Grignard reagent of 70 via an SN2’-type reaction followed by a methylation with trimethylsilyldiazomethane. The intramolecular asymmetric Au(I)-catalyzed pyrrole addition to the allene installed the quaternary carbon stereocenter with good control and formed 73 including the ring D of rhazinilam. To make the pyrrole ring C resistant to the following reaction steps a methyl ester group was introduced first giving 74. In a four step sequence 74 the functionalized side chain was first degraded and then reconstructed to obtain 76. Electrophilic iodination84 followed by Suzuki-Miyaura cross-coupling85 of compound 77 with the commercially available 2-(N-Boc-amino)phenylboronic acid pinacol ester 78 using Buchwald’s S-Phos ligand86 afforded the 3-aryl pyrrole 79 containing rings A, C and D of rhazinilam. Chemoselective ester saponification and TFA-mediated aniline N-deprotection resulted in the formation of the amino acid 80, the precursor for the formation of the B ring. Lactamisation of this compound was carried out with the HATU coupling reagent to produce the previously described55,70,71,77 ester (R)-24 in 74% yield. Removal of the methyl ester group in pyrrole (R)-24, as previously reported by Smith,55 furnished (R)-(-)-rhazinilam (1) in 96% yield and 94% ee. Thus, the synthesis proceeds through fifteen steps starting from commercially available acyl aldehyde 68 in an impressive 19.8% overall yield.
TOTAL SYNTHESIS OF (R)-(-)-RHAZINILAM BY BANWELL
Banwell and co-workers reported a synthesis of rac-rhazinal (7)84 (Scheme 10) and based on this chemistry an enantioselective total synthesis of (R)-(-)-rhazinilam ((R)-1)87 (Scheme 11). The key step of the enantioselctive synthesis is a MacMillan’s chiral organocatalyst88 promoted intramolecular Michael addition reaction closing the D ring and the quaternary center in 74% enantiomeric excess. The synthetic route to the pivotal intermediate 99 started with the N-alkylation of pyrrole with γ-butyrolactone 82 under conditions defined by Li and Snyder.89 Conversion into the corresponding Weinreb amide 84 using modified Mukaiyama conditions90 and subsequent treatment with ethyl magnesium bromide furnished the ketone 85. Wadsworth-Horner-Emmons olefination provided the β,β-disubstituted methyl acrylate 86 followed by reduction with excess DIBAL-H to give the corresponding alcohol 98. The alcohol 98 was oxidized with barium manganate91 to the aldehyde 99 obtained in 76% as a roughly 1:1 mixture of E- and Z-isomers.
The pivotal intramolecular Michael addition reaction involved exposure of this mixture of aldehydes to (5S)-2,2,3-trimethyl-5-phenylmethyl-4-imidazolidinone monotrifluoroacetate 100 (MacMillan’s first generation organocatalyst) resulting in formation of the unstable indolizine aldehyde 101 in 81% yield. Subsequent reduction of compound 101 using sodium borohydride afforded the stable alcohol 88 in 84% yield in 74% ee. The completion of the synthesis of (R)-(-)-rhazinilam (1) involved the one-carbon homologation via SN2 reaction using nitrile as one carbon nucleophile and the installation of the aniline moiety via by Suzuki-Miyaura cross-coupling.85 During this sequence a Vilsmeier-Haack formylation had to be inserted. The aldehyde is at the same time a protecting group for the sensitive pyrrole ring and it dictates the regioselectivety of the following electrophilic iodination to give the iodo-pyrrole 94 in quantitative yield. The final steps were the saponification of the ester followed by lactamisation delivering synthetic (R)-(-)-rhazinal (7). Conversion of (R)-7 into (R)-(-)-rhazinilam (1) was achieved by heating of ((R)-7) with stoichiometric quantities of Wilkinsons “catalyst” in refluxing 1,4-dioxane. The synthesis proceeds through eighteen steps starting from pyrrole 81 and in 4.4% overall yield. Treatment of (R)-(-)-rhazinilam (1) with an excess of pyridinium chlorochromate (PCC) resulted in the conversion of this substrate into a chromatographically separable mixture of (-)-leuconolam (4) (28%) and (+)-epi-leuconolam (6) (46%).
TOTAL SYNTHESES OF ANALOGUES OF RHAZINILAM
Several publications describe efforts dedicated to the total syntheses of analogues of rhazinilam. Some publications report the total syntheses of analogues obtained as “side products” of the efforts dedicated to the total synthesis of rhazinilam. Four different analogues isolated from nature have been synthesized so far: rhazinal in its racemic (two synthesis) and in its enantio-enriched form (one synthesis, see Scheme 11), rhazinicine as racemate (one synthesis) and finally leuconolam and epi-leuconolam in enantioenriched form (one synthesis leading to the mixture of the two natural products, see Scheme 11).
TOTAL SYNTHESES OF RHAZINAL
Total Synthesis of rac-Rhazinal and of (R)-(-)-Rhazinal by Banwell
Both syntheses use an intramolecular Michael addition as key step to form the C-D ring unit (Scheme 10 and 11). In 2003 Banwell and co-workers reported the first total synthesis of racemic rac-rhazinal (rac-7)84 achieved in fifteen steps with 6.6% overall yield. In 2006 the Banwell group achieved the synthesis of the natural (R)-(-)-rhazinal ((R)-7)87 in seventeen steps with 5.0% overall yield.
Total Synthesis of rac-Rhazinal by Trauner
Trauner and co-workers reported92 a concise synthesis of rac-rhazinal (7) using Pd-catalyzed coupling reactions to form the ring D (cyclisation of 112 or alternatively of 109) and the ring B (cyclisation of 117) (Scheme 12). The synthesis of the aliphatic part proceeded through the E-trisubstituted ester 107 which was constructed from the known aldehyde 102.93 The α-methylation followed by treatment with methyllithium afforded the allylic alcohol 104. The Claisen rearrangement of the allylic alcohol produced the ester 105 in 94% yield. Deprotection of silyl ether with tetra-n-butylammonium fluoride and subsequent tosylation of 106 afforded the desired intermediate 107. Reaction of 107 with the potassium salt of pyrrole 111 gave the N-alkylated pyrrole 112 in 67% yield.
The oxidative cyclisation of compound 112 using Pd(OAc)2 in the presence of t-BuOOH resulted in the formation of tetrahydroindolizine 113 in 69% yield. The C-D precursor was then protected as aldehyde 110 by subsequent Vilsmeier-Haack formylation (41% over three steps from compound 107). The intermediate 110 was prepared in parallel by a very similar sequence, where the formyl group was introduced on the pyrrole ring at the desired position from the beginning and where a direct Heck coupling involving the iodopyrrole 108 formed the D-ring.
The chemoselective reduction of the double bond using Crabtree’s catalyst followed by hydrolysis of the ester yielded the corresponding acid 115 in excellent yield. Coupling of the acid 115 with 2-iodoaniline 53 under Mukaiyama’s conditions provided the amide 116,77 which was protected as the methoxymethyl (MOM) key intermediate 117 in 75% yield. The B-ring was formed using 10 mol % of Buchwald’s “DavePhos” ligand 5678 and Pd(OAc)2 in the presence of potassium carbonate to produce the N-MOM rhazinal 118 in 43% yield. After removal of the MOM protecting group with a large excess of boron trichloride racemic rac-(±)-rhazinal (7) was obtained in 45% yield. The synthesis proceeds through thirteen steps in 1.3% overall yield (Method A) or through fourteen steps in 0.8% overall yield (Method B). We have calculated the yield starting from aldehyde 102 which is not commercial. The method used for the preparation of this aldehyde is not indicated.
TOTAL SYNTHESIS OF rac-RHAZINICINE BY GAUNT
The first total synthesis of the rac-rhazinicine (2) was reported by Gaunt and co-workers in 2008.94 The key steps of this convergent synthesis use modern organometallic reactions to connect the rings A and C first and then to form the D-ring. The B-ring is fomed via a lactamization. The connection between the phenyl ring and the pyrrole is achieved by the one-pot IrI-catalyzed C-H bond borylation95,96 directly followed by subsequent Suzuki coupling reaction.97 The oxidative PdII-catalyzed pyrrole C-H bond cyclisation leads to the formation of the D-ring. The synthesis started with the preparation of the two intermediates 122 and 128 (Scheme 13). For the synthesis of the biaryl intermediate 122 containing the A-C rings Boc and TMS protecting groups had to be introduced to obtain the doubly protected precursor 120. The one-pot IrI-catalyzed borylation/Suzuki coupling formed the 3-arylated pyrrole 121 in 78% yield. Removal of Boc-protecting group under heating afforded the pyrrole 122 containing the A-C rings of rhazinicine.
The alkene monoester intermediate 128 was prepared from the commercially available diethyl-4-oxo-pimelate 123 via Wittig ethenylation followed by functional group manipulation to obtain the intermediate 128 containing all the carbons for the rings B and D. Reacting the lithium anion of 122 with acid chloride of 128 the N-acylated pyrrole 129 was obtained in 76% yield (Scheme 14). The intermediate 129 contains all the carbons present in the target molecule. Treating 129 with Pd(TFA)2 catalyst and t-butylperoxybenzoate resulted in cyclisation producing the pivotal tetrahydroindolizine 130 in 53% yield. Simultaneous reduction of the nitro and alkene group of compound 130 followed by AlCl3-mediated removal of the 2-trimethylsilylethyl and trimethylsilyl protecting groups gave the carboxylic acid 132. Lactamisation of this compound under Mukaiyama conditions produced the rac-rhazinicine (2) in 82% yield. The synthesis proceeds through eight steps starting from pyrrole 119 in 1.9% overall yield (through nine steps starting from diester 123 in 1.3% overall yield).
TOTAL SYNTEHSIS OF LEUCONOLAM AND Epi-LEUCONOLAM BY BANWELL
Banwell and co-workers reported the first total syntheses of (-)-leuconolam (4) achieved in nineteen steps with 1.2% overall yield, and (+)-epi-leuconolam (6)87 achieved in nineteen steps with 2.0% overall yield. Both syntheses involved as a key step an intramolecular Michael addition reaction. They were described in Scheme 11.
COMPARISON OF THE PUBLISHED RHAZINILAM SYNTHESES
GENERAL REMARKS
The synthesis of rhazinilam has been studied for now almost 40 years. Seven different syntheses have been reported so far: four of rac-rhazinilam and three of (R)-rhazinilam. Four analogues of rhazinilam have been synthesized by three different groups using three different approaches: rac-rhazinal, (R)-rhazinal, rac-rhazinicine, (R)-leuconolam and (R)-epi-leuconolam. The methods to construct the rhazinilam skeleton have changed over the years, however the retrosynthetic disconnections for all these synthesis have stayed rather similar. The major difference between the different syntheses is the sequence of the synthetic steps forming the tetracyclic structure and the methods used to create the different rings of rhazinilam. Most of the recent studies reported the synthesis of the enantiomerically enriched natural product (ee between 74% and 96%). The research leading to the very first synthesis had clearly been started before the structure of (R)-rhazinilam had been ascertained by the structure determination of the crystal by X-ray diffraction. This initial synthesis project follows therefore the logic of structure determination by total synthesis. For almost 30 years no new academic synthesis was reported. The recent synthesis reported since 2000 have been designed around one or several key steps. The goal of these syntheses is to showcase the efficiency of these key steps applying them to a “real” synthetic goal. The use of transition metals for the forming of C-C bonds is illustrated in several of the recent total synthesis. Another important feature illustrating the latest development in organic synthesis is the application of organocatalysis for the asymmetric synthesis of a key intermediate.
COMPARISON OF THE NUMBER OF SYNTHETIC STEPS AND THE OVERALL YIELD
The development of synthetic methodologies since 1973 has been spectacular and it seems almost unfair to compare the first synthesis published in 1973 with the most recent synthesis published in 2006. If one wants to compare the overall yields and the number of synthetic steps from commercially available starting material for all seven syntheses we had in some cases to go back to the literature reports cited by the authors to identify the commercial starting materials. In these cases we assumed that the authors have used the reported procedure and that the authors had obtained the yields of the cited procedures.
The number of steps from commercial starting material varies from 9 to 20 steps (Scheme 15). The lowest overall yield is 1.6% and the best 19.8%. The average yield per step is more than 70% for all the synthesis reported. The highest yielding synthesis reports an impressive average yield of 90% over 15 steps. The short syntheses tend to be more efficient, but it is interesting to emphasize that five of the seven syntheses have an overall yield between 1.6 and 4.4%, despite the fact that the shortest of these syntheses needs only 10 steps whereas the longest needs 20 steps, twice as many compared with the shortest synthesis.
Looking at these syntheses from the standpoint of overall yield the first synthesis is surprisingly efficient. In comparing the four syntheses of rac-rhazinilam the shortest synthesis with only 9 steps has been reported by Magnus. In the Magnus synthesis no protecting group on the pyrrole ring was introduced, which shortens the synthetic pathway by at least two steps. The three enantioselective syntheses are all considerably longer, 15 to 18 steps. In one case a chiral auxiliary had to be introduced and to be cleaved. In the enantioselective synthesis by Nelson and by Banwell an enantioselective catalytic process is applied. The overall lengths of these two syntheses are 15 and 18 steps, respectively. Based on the overall yield the synthesis reported by the Nelson group stands out with an overall yield, which is three times higher than the Magnus synthesis and at least a factor of five better than most of the other synthesis.
COMPARISON OF THE RETROSYNTHETIC ANALYSIS USED
The summary of the retrosynthetic analysis used in the different total syntheses leads to some interesting conclusions (Scheme 16). In all the syntheses the B-ring is formed last. Due to this common retrosynthetic analysis the end game of all syntheses is quite similar. In six of the seven syntheses the lactam formation is the last step finishing the construction of the skeleton of rhazinilam. Only in the Trauner synthesis the B-ring is closed via a Heck type C-C coupling. One has however to mention that the Trauner synthesis is an inversion of the sequence used by Nelson and Banwell. In Trauner’s synthesis the amide bond of the B-ring is formed first and the ring closure is achieved via the Heck type coupling second. In the syntheses of Nelson and Banwell the sequence is inverted. The Suzuki coupling is used for the creation of the biaryl unity.
The D-ring is formed in four syntheses just before closing the lactam ring (ring B). In the recent syntheses the aromatic A ring is introduced at the later stage of the synthesis by a coupling reaction (Banwell, Nelson, Trauner syntheses). In the two syntheses by Sames and the synthesis reported by Magnus the D-ring is part of the starting material and stays intact during the whole synthetic sequence.
Three of the seven syntheses use a commercial precursor containing the intact pyrrole ring (Banwell, Nelson, Trauner syntheses). The groups of Smith, Sames and of Magnus have applied synthetic schemes, where the pyrrole ring is constructed during the preparation of rhazinilam. Smith uses a variant of a Knorr-type pyrrole synthesis whereas Sames and Magnus use a Grigg-type 1,5-electrocyclization.
Another observation concerns the use of “protecting” or stabilizing on the pyrrole ring. With one exception, all syntheses have to introduce or to carry on a electron attracting substituent on the pyrrole ring. Most syntheses use an ester group in the α-position (Scheme 17). The Banwell synthesis uses an aldehyde function as protection group of the pyrrole. Very often two steps are needed to introduce the protecting group and one to two steps are again needed for the deprotection. The protecting group is in most of the syntheses carried along a good part of the synthetic sequence. In the Sames synthesis the ester protecting group is present during 14 steps. These substituents have to be removed towards the end of the synthesis. Even if it is not formally stated in most of the publications, their function is clearly to make the intermediates stable enough, that they can be manipulated. The exception is the Magnus synthesis. Despite the fact that the Magnus’ group uses an intermediate, which is identical with the one reported in the first synthesis of Sames, the Magnus group was able to avoid the use of electron attracting group on the pyrrole ring.
In summary the synthesis of rhazinilam is still a challenging synthetic problem. The syntheses reported are attractive and incorporate different interesting key steps. Despite the spectacular developments of organic synthesis even the very first reported synthesis is still a valid approach to this fascinating natural product.
References
1. B. Ebbell, The Papyrus Ebers; Levin & Munksgaard: Copenhagen, 1937.
2. G. M. Cragg, P. G. Grothaus, and D. J. Newman, Chem. Rev., 2009, 109, 3012. CrossRef
3. G. M. Ebers and L. Stern, Papyros Ebers; W. Englemann: Leipzig, 1875.
4. H. Joachim, Papyros Ebers: Das älteste Buch über die Heilkunde.; G. Reimer: Berlin, 1890.
5. W. Wreszinski, Der Papyrus Ebers: Umschrift, Übersetzung und Kommentar.; J. C. Hinrichs'sche Buchhandlung: Leipzig, 1913.
6. P. Ghalioungui, The Ebers Papyrus: A New English Translation, Commentaries, and Glossaries.; Academy of Scientific Research and Technology: Cairo, 1987.
7. C. Brink, D. C. Hodgkin, J. Lindsey, J. Pickworth, J. H. Robertson, and J. G. White, Nature, 1954, 174, 1169. CrossRef
8. D. C. Hodgkin, J. Pickworth, J. H. Robertson, K. N. Trueblood, R. J. Prosen, and J. G. White, Nature, 1955, 176, 325. CrossRef
9. M. J. Kamper and D. C. Hodgkin, Nature, 1955, 176, 551. CrossRef
10. D. C. Hodgkin, A. W. Johnson, and A. R. Todd, Chem. Soc., 1955, 3, 109.
11. J. D. Watson and F. H. C. Crick, Nature, 1953, 171, 737. CrossRef
12. A. P. Ryle, F. Sanger, L. F. Smith, and R. Kitai, Biochem. J., 1955, 60, 541.
13. J. C. Kendrew and R. G. Parrish, Proc. R. Soc. London, Ser. A, 1957, 238, 305. CrossRef
14. R. B. Merrifield, J. Am. Chem. Soc., 1963, 85, 2149. CrossRef
15. M. Goodman, A. Felix, L. Moroder, and C. Toniolo, Synthesis of Peptides; Thieme Verlag: Stuttgart, 2002; Vol. E22a and E22b.
16. M. S. Butler, Nat. Prod. Rep., 2008, 25, 475. CrossRef
17. K.-H. Altmann and J. Gertsch, Nat. Prod. Rep., 2007, 24, 327. CrossRef
18. F. von Nussbaum, M. Brands, B. Hinzen, S. Weigand, and D. Haebich, Angew. Chem. Int. Ed., 2006, 45, 5072. CrossRef
19. Y.-W. Chin, M. J. Balunas, H. B. Chai, and A. D. Kinghorn, AAPS J., 2006, 8, E239.
20. M. Hamburger, Chimia, 2006, 60, 14. CrossRef
21. E. F. Queiroz, K. M. Ahua, and K. Hostettmann, Chimia, 2005, 59, 299. CrossRef
22. P. Proksch, R. Ebel, R. A. Edrada, P. Schupp, W. H. Lin, Sudarsono, V. Wray, and K. Steube, Pure Appl. Chem., 2003, 75, 343. CrossRef
23. I. Paterson and E. A. Anderson, Science, 2005, 310, 451. CrossRef
24. S. L. Schreiber, Nat. Chem. Biol., 2005, 1, 64. CrossRef
25. C. M. Dobson, Nature, 2004, 432, 824. CrossRef
26. J. Clardy and C. Walsh, Nature, 2004, 432, 829. CrossRef
27. S. J. Miller and J. Clardy, Nat. Chem., 2009, 1, 261. CrossRef
28. C. T. Walsh, Nat. Chem. Biol., 2005, 1, 122. CrossRef
29. J. Meinwald and T. Eisner, Helv. Chim. Acta, 2003, 86, 3633. CrossRef
30. T. Eisner, Proc. Natl. Acad. Sci. U. S. A., 2003, 100, 14517. CrossRef
31. C. Khosla, J. Org. Chem., 2009, 74, 6416. CrossRef
32. K. J. Weissman and P. F. Leadlay, Nat. Rev. Microbiol., 2005, 3, 925. CrossRef
33. P. F. Leadlay, J. Staunton, A. F. A Marsden, B. Wilkinson, N. J. Dunster, J. Cortes, M. Oliynyk, U. Hanefeld, and M. J. B. Brown, Dev. Ind. Microbiol. Ser., 1997, 34, 33.
34. A. Gossauer, Fortschr. Chem. Org. Naturst., 2003, 86, 1.
35. G. H. Cookson and C. Rimington, Biochem. J., 1954, 57, 476.
36. M. J. Weiss, J. S. Webb, and J. M. Smith, Jr., J. Am. Chem. Soc., 1957, 79, 1266. CrossRef
37. C. W. Waller, C. F. Wolf, W. J. Stein, and B. L. Hutchings, J. Am. Chem. Soc., 1957, 79, 1265. CrossRef
38. F. Schroeder, S. Franke, W. Francke, H. Baumann, M. Kaib, J. M. Pasteels, and D. Daloze, Tetrahedron, 1996, 52, 13539. CrossRef
39. H. H. A. Linde, Helv. Chim. Acta, 1965, 48, 1822. CrossRef
40. A. Banerji, P. L. Majumder, and A. Chatterjee, Phytochemistry, 1970, 9, 1491. CrossRef
41. K. T. De Silva, A. H. Ratcliffe, G. F. Smith, and G. N. Smith, Tetrahedron Lett., 1972, 10, 913. CrossRef
42. R. L. Lyon, H. H. S. Fong, N. R. Farnsworth, and G. H. Svoboda, J. Pharm. Sci., 1973, 62, 218. CrossRef
43. D. J. Abraham, R. D. Rosenstein, R. L. Lyon, and H. H. S. Fong, Tetrahedron Lett., 1972, 10, 909. CrossRef
44. P. S. Benoit, G. Angry, R. L. Lyon, H. H. S. Fong, and N. R. Farnsworth, J. Pharm. Sci., 1973, 62, 1889. CrossRef
45. G. M. T. Robert, A. Ahond, C. Poupat, P. Potier, C. Jolles, A. Jousselin, and H. Jacquemin, J. Nat. Prod., 1983, 46, 694. CrossRef
46. S. H. Goh and A. R. M. Ali, Tetrahedron Lett., 1986, 27, 2501. CrossRef
47. O. Thoison, D. Guenard, T. Sevenet, C. Kan-Fan, J. C. Quirion, H. P. Husson, J. R. Deverre, K. C. Chan, and P. Potier, C. R. Acad. Sci., Ser. 2, 1987, 304, 157.
48. S. H. Goh, A. R. M. Ali, and W. H. Wong, Tetrahedron, 1989, 45, 7899. CrossRef
49. T. Varea, C. Kan, F. Remy, T. Sevenet, J. C. Quirion, H. P. Husson, and H. A. Hadi, J. Nat. Prod., 1993, 56, 2166. CrossRef
50. M. Zeches, K. Mesbah, B. Richard, C. Moretti, J. M. Nuzillard, and L. Le Men-Olivier, Planta Med., 1995, 61, 89. CrossRef
51. Y. Sheludko, I. Gerasimenko, and O. Platonova, Planta Med., 2000, 66, 656. CrossRef
52. K.-H. Lim, O. Hiraku, K. Komiyama, T. Koyano, M. Hayashi, and T.-S. Kam, J. Nat. Prod., 2007, 70, 1302. CrossRef
53. Y. Wu, M. Suehiro, M. Kitajima, T. Matsuzaki, S. Hashimoto, M. Nagaoka, R. Zhang, and H. Takayama, J. Nat. Prod., 2009, 72, 204. CrossRef
54. K. Jewers, D. F. G. Pusey, S. R. Sharma, and Y. Ahmad, Planta Med., 1980, 38, 359. CrossRef
55. A. H. Ratcliffe, G. F. Smith, and G. N. Smith, Tetrahedron Lett., 1973, 52, 5179. CrossRef
56. B. David, T. Sevenet, O. Thoison, K. Awang, M. Pais, M. Wright, and D. Guenard, Bioorg. Med. Chem. Lett., 1997, 7, 2155. CrossRef
57. C. Dupont, D. L. Guenard, Tchertanov, S. Thoret, and F. Gueritte, Bioorg. Med. Chem., 1999, 7, 2961. CrossRef
58. R. Kreher and J. Seubert, Angew. Chem., 1964, 76, 682. CrossRef
59. G. Subramaniam, O. Hiraku, M. Hayashi, T. Koyano, K. Komiyama, and T.-S. Kam, J. Nat. Prod., 2008, 71, 53. CrossRef
60. T. S. Kam and G. Subramaniam, Nat. Prod. Lett., 1998, 11, 131.
61. I. Gerasimenko, Y. Sheludko, and J. Stoeckigt, J. Nat. Prod., 2001, 64, 114. CrossRef
62. H. Zhou, H.-P. He, N.-C. Kong, Y.-H. Wang, X.-D. Liu, and X.-J. Hao, Helv. Chim. Acta, 2006, 89, 515. CrossRef
63. G. Subramaniam, O. Hiraku, M. Hayashi, T. Koyano, K. Komiyama, and T.-S. Kam, J. Nat. Prod., 2007, 70, 1783. CrossRef
64. N. Aimi, N. Uchida, N. Ohya, H. Hosokawa, H. Takayama, S. Sakai, L. A. Mendonza, L. Polz, and J. Stoeckigt, Tetrahedron Lett., 1991, 32, 4949. CrossRef
65. S. H. Goh, C. Wei, and A. R. M. Ali, Tetrahedron Lett., 1984, 25, 3483. CrossRef
66. F. Abe and T. Yamauchi, Phytochemistry, 1994, 35, 169. CrossRef
67. Atta-ur-Rahman, S. Abbas, K. Zaman, M. M. Qureshi, F. Nighat, and A. Muzaffar, Nat. Prod. Lett., 1995, 5, 245.
68. T.-S. Kam, Y.-M. Tee, and G. Subramaniam, Nat. Prod. Lett., 1998, 12, 307.
69. A. Gossauer, Fortschr. Chem. Org. Naturst., 2003, 86, 1.
70. J. A. Johnson and D. Sames, J. Am. Chem. Soc., 2000, 122, 6321. CrossRef
71. J. A. Johnson, N. Li, and D. Sames, J. Am. Chem. Soc., 2002, 124, 6900. CrossRef
72. P. Magnus and T. Rainey, Tetrahedron, 2001, 57, 8647. CrossRef
73. S. M. Liebowitz, E. J. Belair, D. T. Witiak, and D. Lednicer, Eur. J. Med. Chem.--Chim. Ther., 1986, 21, 439.
74. R. Grigg, P. Myers, A. Somasunderam, and V. Sridharan, Tetrahedron, 1992, 48, 9735. CrossRef
75. G. S. Hill, M. J. Irwin, C. J. Levy, L. M. Rendina, and R. J. Puddephatt, Inorg. Synth., 1998, 32, 149. CrossRef
76. J. R. Parikh and W. v. E. Doering, J. Am. Chem. Soc., 1967, 89, 5505. CrossRef
77. A. L. Bowie, Jr., C. C. Hughes, and D. Trauner, Org. Lett., 2005, 7, 5207. CrossRef
78. M. C. Harris, O. Geis, and S. L. Buchwald, J. Org. Chem., 1999, 64, 6019. CrossRef
79. Z. Liu, A. S. Wasmuth, and S. G. Nelson, J. Am. Chem. Soc., 2006, 128, 10352. CrossRef
80. S. G. Nelson, P. M. Mills, T. Ohshima, and M. Shibasaki, Org. Synth., 2005, 82, 170.
81. S. G. Nelson and Z. Wan, Org. Lett., 2000, 2, 1883. CrossRef
82. S. G. Nelson, T. J. Peelen, and Z. Wan, J. Am. Chem. Soc., 1999, 121, 9742. CrossRef
83. C. Zhu, X. Shen, and S. G. Nelson, J. Am. Chem. Soc., 2004, 126, 5352. CrossRef
84. M. G. Banwell, A. J. Edwards, K. A. Jolliffe, J. A. Smith, E. Hamel, and P. Verdier-Pinard, Org. Biomol. Chem., 2003, 1, 296. CrossRef
85. N. Miyaura and A. Suzuki, Chem. Rev., 1995, 95, 2457. CrossRef
86. T. E. Barder, S. D. Walker, J. R. Martinelli, and S. L. Buchwald, J. Am. Chem. Soc., 2005, 127, 4685. CrossRef
87. M. G. Banwell, D. A. S. Beck, and A. C. Willis, ARKIVOC, 2006, 3, 163.
88. N. A. Paras and D. W. C. MacMillan, J. Am. Chem. Soc., 2001, 123, 4370. CrossRef
89. J. H. Li and J. K. Snyder, J. Org. Chem., 1993, 58, 516. CrossRef
90. M. Banwell and J. Smith, Synth. Commun., 2001, 31, 2011. CrossRef
91. A. J. Fatiadi, Synthesis, 1987, 2, 85. CrossRef
92. A. L. Bowie, Jr. and D. Trauner, J. Org. Chem., 2009, 74, 1581. CrossRef
93. F. Freeman, D. S. H. L. Kim, and E. Rodriguez, J. Org. Chem., 1992, 57, 1722. CrossRef
94. E. M. Beck, R. Hatley, and M. J. Gaunt, Angew. Chem. Int. Ed., 2008, 47, 3004. CrossRef
95. J.-Y. Cho, M. K. Tse, D. Holmes, R. E. Maleczka, Jr., and M. R. Smith, III, Science, 2002, 295, 305. CrossRef
96. T. Ishiyama, J. Takagi, K. Ishida, N. Miyaura, N. R. Anastasi, and J. F. Hartwig, J. Am. Chem. Soc., 2002, 124, 390. CrossRef
97. S. D. Walker, T. E. Barder, J. R. Martinelli, and S. L. Buchwald, Angew. Chem. Int. Ed., 2004, 43, 1871. CrossRef
98. O. Vallat, PhD thesis, Université de Neuchâtel, Switzerland, 2004.
99. A.-M. Buciumas, PhD thesis, Université de Neuchâtel, Switzerland, 2008.