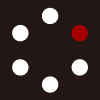
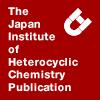
HETEROCYCLES
An International Journal for Reviews and Communications in Heterocyclic ChemistryWeb Edition ISSN: 1881-0942
Published online by The Japan Institute of Heterocyclic Chemistry
e-Journal
Full Text HTML
Received, 3rd June, 2012, Accepted, 8th August, 2012, Published online, 15th August, 2012.
DOI: 10.3987/REV-12-SR(N)3
■ CROSS-COUPLING REACTIONS FOR THE SYNTHESIS OF C-GLYCOSIDES AND RELATED COMPOUNDS
Pedro Merino,* Tomas Tejero, Eduardo Marca, Fernando Gomollón-Bel, Ignacio Delso, and Rosa Matute
Department of Organic Chemistry, University of Zaragoza, E-50009 Zaragoza, Aragon, Spain
Abstract
Cross-coupling reactions provide versatile strategies for preparing hydrolytically stable C-glycosides and C-nucleoside analogues, which are of interest in the search for new biologically active molecules. Several types of cross-coupling reactions have been studied, including Heck, Suzuki, Stille and Negishi couplings. The aim of this work is to review the state-of-the-art in that sort of reactions applied to the synthesis of C-glycosides and related compounds.CONTENTS
1. Introduction
2. Heck reactions and related processes
3. Suzuki reactions and related processes
4. Stille and Hiyama reactions and related processes
5. Negishi reactions and related processes
6. Other Reactions
7. Concluding remarks
Acknowledgments
References
1. INTRODUCTION
Cross-coupling reactions catalyzed by palladium and other transition metals are efficient and effective methods in the synthesis of a great variety of organic compounds. These transformations are of considerable interest due to their fundamental importance, as well as for their widespread occurrence in synthetic and catalytic processes.1 In fact, there are a number of well-known name reactions that feature palladium as an excellent catalyst for cross-coupling reactions, including the Heck,2 Suzuki,3 Stille,4 Negishi,5 Hiyama,6 Sonogashira,7 Buchwald-Hartwig8 and Heck-Matsuda9 reactions. The importance of all these processes became evident when fundamental contributions to that topic by Ei-ichi Negishi, Akira Suzuki and Richard F. Heck were recognized with the 2010 Nobel Prize in Chemistry "for palladium-catalyzed cross-coupling in organic synthesis".10 Among the myriad compounds that can be prepared by cross-coupling reactions, C-glycosides11 and C-nucleoside analogues12 are of particular interest because of their biological importance.
C-Glycosides are defined, by analogy with conventional O-glycosides, as those compounds in which the anomeric exo-oxygen has been replaced by a carbon atom, and they can be divided into aryl and alkyl C-glycosides. C-nucleosides are defined as those analogues to natural nucleosides in which the anomeric exo-nitrogen atom has also been replaced by a carbon atom. Usually, these analogues are limited to those bearing an (hetero)aromatic ring at the anomeric position. In both cases C-O and C-N bonds have been replaced by hydrolytically stable C-C bonds, which confer to the C-analogues particular and important biological activities.
Even though the biological properties of C-glycosides and C-nucleosides are quite different with very diverse implication to biological processes, from a synthetic point of view they are closely related. Both types of analogues can be prepared by the direct incorporation of the alkyl, aryl or hetaryl moiety to the sugar framework. This approach is rather adequate to consider cross-coupling reactions as a suitable methodology.
Depending on the reaction different substrates are required. Glycals are suitable starting materials for incorporating the sugar unit due to their availability.13 Glycals can be coupled with aryl halides (Heck coupling) and boronates (Suzuki coupling). Halogenated glycals can be employed as substrates in Stille reactions with organostannanes, the opposite functionalization (stannylglycals with halides) being also possible. In addition to glycals, glycosyl bromides and iodides are also adequate starting materials for the reaction with organozinc compounds (Negishi coupling). The synthesis of C-glycosides and C-nucleosides has been reviewed elsewhere,14 including the use of Heck reactions.15
In this report, the synthetic approaches outlined in Scheme 1 as well as related methodologies directed to the preparation of C-glycosides and related compounds will be discussed. Only approaches in which the sugar moiety is directed involved in the reaction are considered. Other syntheses where the carbohydrate is just a substituent are not considered. For the sake of clarity this review has been arranged by the type of reaction.
2. HECK REACTIONS AND RELATED PROCESSES
The Heck reaction involves an alkene containing at least one proton, an unsaturated halide (or triflate) and a base. It is carried out in the presence of an organopalladium catalyst.16 Some variations in the reagents are possible and several reviews have been reported.17 For general considerations and mechanistic discussion on Heck reaction the reader is directed to those revisions.
Coupling reactions between cyclic enol ether 1 and aryl compounds using a catalytic amount of palladium was reported for the first time by Daves and co-workers. Treatment of an excess of 1 with iodobenzene 2 and iodouracil 3 in the presence of 1 mol% of catalyst 4 afforded 5 and 6, respectively, in good yields (Scheme 2). The addition of both iodobenzene 218 and iodouracil 3 took place with migration of the double bond,19 which was attributed to the high temperature. For the reaction with iodobenzene the yield of 5 increased to 86% when catalyst was palladium on carbon.
A similar reaction with glycal 7 and 2 using Pd(II) acetate as a catalyst required more temperature and the corresponding product 8, showing migration of the double bond, was obtained in only 30% yield.20 By carrying out the reaction with 3 at lower temperature and in the presence of additional tetrabutylammonium chloride, a 1:2 mixture of isomers 9a and 9b were obtained after 6 days in 60% combined yield (Scheme 3).21 In the same paper it was also reported the efficient coupling of iododerivatives of anthracycline with furanoid and pyranoid glycals by using stoichiometric amounts of reactants, catalytic palladium(II) acetate and a tertiary amine using DMF as a solvent at room temperature.
Glycal-derived enones also underwent palladium-mediated arylation to give mixtures of C-glycosides bearing an arylated enone and an arylated ketone.22 Unprotected 5-iodouracil was coupled with glycal 10 in the presence of catalytic palladium(II) acetate and triphenylarsine. The corresponding adduct was not isolated but used in a one-pot procedure to prepare 2'-deoxypseudouridine 12 as shown in Scheme 4.23
Similar reactions leading to the same C-nucleosides have been reported by employing the mercuric acetate derivative of compound 3.24 Aryl iodides are excellent substrates for typical Heck reactions catalyzed by palladium(II) acetate in the presence of triphenylarsine and triethylamine. Cross-coupling reactions with furanoid derivatives provided important C-nucleoside analogues (Scheme 5). The reaction times can vary from 24 h25 to several days.26 The presence of protecting groups in the glycal should also be considered. Thus, it has also been reported a lack of reactivity for 4-hydroxymethyl furanoid glycals, bearing a bulky protecting group (i.e. tert-butyldimethylsilyl) at the 5'-hydroxyl group.27
The addition of silver salts as additives contributed to increase the chemical yield of the reaction. Excellent results were obtained in the palladium-catalyzed Heck reactions between glycal 18 and several aryl iodides when the reaction was carried out in the presence of silver carbonate and copper (II) acetate. It has been proposed that silver carbonate could play a dual role by acting as a base and as a silver source to scavenge the iodide (Scheme 6).28
Microwave activation also provided good results for the reaction. As an example, in Scheme 7 the cross-coupling reaction between glycal 21 and several aryl bromides is illustrated. The methodology was applied to several perbenzylated glycals of different configurations and the corresponding C-aryl glycosides were obtained in a rapid and totally stereoselective way.29
Aryldiazonium salts were used instead of aryl iodide in Heck reactions with five-membered enecarbamates en route to polyhydroxylated pyrrolidines, which can be considered iminosugar analogues. Correia and co-workers reported the Heck arylation of 24 to furnish Δ3-pyrroline 26 (Scheme 8).30
Arylation with arenediazonium salts is highly dependent on the substituents of the aromatic ring and on the nature of the group at C-5 on the enecarbamate. Whereas arylation of 27 is only feasible with salts bearing electron-donating groups, arylation of 29 took place rapidly providing 30 as a mixture of isomers but in good yields (Scheme 9).31 Compounds 28 and 30 were used in the synthesis of aza analogues of isoaltholactone and goniothalesdiol.
Arenediazonium salts are also highly reactive with cyclic enol-ethers32 including furanoid glycals bearing bulky protecting groups at 5'.31 As an example, treatment of glycal 31 with 25a in the presence of 2.5 mol% Pd2(dba)3·CHCl3 furnished C-aryl glycosides 32 in good yields as a single α-isomer, no undesired double bond migration being observed (Scheme 10).33
Enol triflates 34 effectively reacted with glycals 35 in cross-coupling reactions that took place at the anomeric carbon providing exclusively α-isomers. The reaction presented a broad scope in both glycals and enol triflates.34
A decarboxylative Heck coupling reaction between glycals and benzoic acids has been reported by Liu and co-workers.35 The reaction showed a broad scope and versatility affording the corresponding C-glycosides in moderate to good chemical yields but complete regio- and stereoselectivities (Scheme 12).
The intramolecular Heck reaction of glycosides 39-41 provided and entry to cis-fused bi- or tricyclic derivatives containing five- and six-membered oxa and carbocyclic rings in good yields (Scheme 13). Different reaction conditions were employed and similar results were obtained with acetonitrile and toluene as solvents. On the other hand, a mixture of isomers was obtained with 39 when triethylamine was used as a base but a complete selectivity was observed with silver carbonate. Indeed, the formation of isomeric mixtures was attributed to further epimerization after the cross-coupling reaction.36
The obtention of fused bicyclic compounds with exocyclic bonds like 43 was also observed with carbohydrate templates bearing the alkoxyalkenyl moiety at C-3 in a Heck reaction catalyzed by the system Pd(OAc)2/PPh3 in the presence of triethylamine and Bu4NHSO4.37
3. SUZUKI REACTIONS AND RELATED PROCESSES
The Suzuki coupling consists of the reaction between an aryl- or vinyl-boronic acid and an aryl or vinyl halide catalyzed by a palladium complex.38 The reaction also works with triflates instead of halides and some organoboranes can be used in place of boronoic acids.
Cross coupling of halo-exo-glycals with boronic acids was reported by Lopez and co-workers.39 The reaction took place in moderate to good yields upon catalysis with palladium (0). The reactivity of iodides is higher than that of bromides and alkenyl halides activated by the proximity of electron-withdrawing groups are more reactive than those with electron-donating groups (Scheme 14). The reaction was also carried out with six-membered iodo-exo-glycals and both boronic acids and alkyl boranes produced good results.40
Dibromosubstituted exo-glycals underwent Suzuki coupling with aryl- and hetaryl-boronic acids but mixtures of monoarylated isomers and substrates incorporating two aryl units were obtained.41 In the case of monosusbtituted exo-glycals, the remaining bromine atom was used to introduce a second aryl group through Pd-catalyzed cross-coupling. The approach can be considered highly versatile because of the possibility of preparing any isomer in a stereochemically pure form just by exchanging the order of introduction of the aryl groups.42 This is illustrated in Scheme 15 for compound 48.
Cross-coupling between organoboranes derived from exo-glycals and aryl halides was reported by Johnson and co-workers.43 Exo-glycal 55 underwent smooth hydroboration to give the alkylborane which was coupled in situ with aryl halides under standard Suzuki conditions (Scheme 16).
A similar approach was used for preparing C-glycoside analogues of phloriain, a specific inhibitor of sodium glucose co-transporter with antidiabetic properties.44 Coupling of alkylboranes derived from glycals with aryl triflates was affected by steric hindrance of the aryl moiety. While coupling of the organoborane derived from 57 with triflate 58 took place smoothly at room temperature in 2 h, the same reaction with triflate 59 required stirring overnight at 80 ºC (Scheme 17).45 The resulting adduct 61 was used in the preparation of the C-glycoside analogue of pseudopterosin A, a potent anti-inflammatory agent.46
odoglycal 62, easily available from the parent glycal, was converted into C1-alkyl glycals by Suzuki coupling with alkylboranes prepared in situ from the corresponding olefins (Scheme 18).47 1-Iodoglycals were also coupled in a similar way with both boronic acids and boronates.48
A variety of bromoheterocycles were made to react with C-glycosylpinacol boronate ester 64 under typical Suzuki conditions (Scheme 19).49
Compound 64 has also been employed for the synthesis of several 2-arylglycals through the Suzuki-coupling with aryl bromides (Scheme 20).50 The reaction took place with excellent yields and an anti-HIV active berginin derivative was prepared to illustrate the synthetic utility of the methodology.
2-Arylglycals have been prepared through phosphine-free Suzuki coupling of 2-iodoglycals in aqueous media. The reaction of compound 68 with arylboronic acids in the presence of palladium catalyst was carried out under microwave activation. Under these conditions excellent yields were achieved in 5 min except in two cases that required 40 min of reaction (Scheme 21).51 The scope of the reaction was also extended to various 2-iodoglycals differentially protected thus providing the methodology with a high versatility. Introduction of aryl groups at C-2 was also possible in iminoglycals (enecarbamates) by using phenylboronic acid and Pd(dppf)Cl2 as a catalyst, in the presence of potassium phosphate.52
The reaction of several glycals with various boronic acids using palladium(II) acetate as catalyst in the presence of various oxidants were reported by Ye and co-workers.53 Depending on the oxidizing system different products were obtained as illustrated with glycal 71 (Scheme 22). In the presence of benzoquinone, ketone 72 was obtained; with Cu(OAc)2/O2 the enol ether 73 was the product of the reaction and with DDQ compound 74 was the only product of the reaction. All the reactions showed a broad scope in both the glycal and the arylboronic acid, proceeding in all cases with high regio- and stereoselectivities.
4. STILLE AND HIYAMA REACTIONS AND RELATED PROCESSES
The Stille reaction is a coupling reaction between an organotin compound and a (hetero)aryl or vinyl halide, catalyzed by palladium.54 The reaction should be carried out in an inert atmosphere and degassed solvents to avoid oxidation of the catalyst. The mechanism of the Stille reaction has been studied in detail and the reader is referred to the excellent reports published in the literature.55
Lopez and co-workers reported the stereocontrolled synthesis of substituted exo-glycals by Stille cross-coupling of (Z)-halo-exo-glycals and aryl or alkenylstannanes (Scheme 23).56 The reaction was also applied to dibromo exo-glycals although mixtures of isomers were obtained.41
Stannylated glycals, easily available through direct metalation of glycals are excellent substrates for Stille coupling reactions with aryl and alkenyl halides. Friesen and Sturino reported the palladium-catalyzed coupling of aryl bromides and 1-(tributylstannyl)-D-glucal 78 (Scheme 24).48,57 The reaction took place with moderate yields in most cases and minor amounts (8-15%) of dimer 80 were also obtained.
Similarly, stannyl-D-glucal 81 was coupled with alkenyl iodides 82 en route to avermectin (Scheme 25).58 The reaction of O-TBS protected 82a was catalyzed by 5 mol% Pd2(dba)3·CHCl3 and 83a was obtained in only 35% yield. By using unprotected alkenyl iodide 82b and Pd(MeCN)2Cl2 as catalyst the yield of the reaction was increased to 57%.
The reaction between stannyl-D-glucal 84 and α-iodostyrene using Pd(PPh3)4 as a catalyst in the presence of 10 mol% of CuI and CsF afforded adduct 85 in 66% yield (Scheme 26).59 Compound 85 was used as an advanced intermediate for the synthesis of the antibiotic and antitumoral altromycin B.
A desulfurative Stille coupling between glycal 86 and aryl or benzylsulfonyl chlorides was reported by Vogel and co-workers (Scheme 27).60
When the reaction was carried out with 86a and in the presence of carbon monoxide enone 88 was obtained together with some amounts (up to 15%) of an enone resulting from the homocoupling of 86a (Scheme 28). This carbonylative Stille cross-coupling was employed by the same authors for synthesizing C-(1,4)-linked disaccharides.61 The cross-coupling reaction between glycals 89 and triflate 90 afforded adducts 91 in good yields (Scheme 29). These adducts were further transformed into the target C-disaccharides.
The reaction showed to be sensitive to CO pressure. Indeed, no reaction took place and the starting materials decomposed below 40 bar. Also, the amount of LiCl is crucial and whereas with a Pd/LiCl ratio of 1:1 only a 23% yield is obtained, a 1:3 ratio increased the yield up to more than 70%. The reaction was also checked with iodoglycals 92 and the corresponding dienones 93 bearing two glycal units were obtained in good yields (Scheme 30).61 In this case, using THF as a solvent led to better results than using N-methylpyrrolidinone (NMP).
Falck and co-workers described the use of stannyl glucopyranosides in cross-coupling reactions with thiono and thiochloroformates catalyzed by PdCl2(dppf) and CuCN (8 mol% each) (Scheme 31).62
Diene 96 was obtained through Stille coupling of vinyltributyltin with the corresponding imino bromo glycal (Scheme 32).52
In a related process cyclic (α-alkoxyvinyl)silanol 97 was efficiently transformed into aryl vinyl ether 98 through a palladium(0)-catalyzed process. The reaction was activated by an excess of tetrabutylammonium fluoride and it took place with very good yields (Scheme 33).63 This reactivity was employed for the preparation of an intermediate in the synthesis of the fungicide (+)-papulacandin D.64
The initial reaction showed to be rather sensitive to the iodide. Whereas cross-coupling between 99 and 4-iodoanisole took place in 81% yield, no reaction was observed with aryl iodide 101 and only desilylated glycal 102 was obtained (Scheme 34). On the other hand, after an optimization study it was found that differentially protected 103 reacted with 104, in the presence of potassium tert-butoxide, to give the expected adducts in good yield (Scheme 35).63,64
5. NEGISHI REACTIONS AND RELATED PROCESSES
The Negishi coupling reaction involves an organozinc compound and an organic halide. The reaction is catalyzed by nickel or palladium catalysts, usually with phosphorous ligands.65 Both organozinc halides and diorganozinc compounds can be used in this reaction and detailed mechanistic studies have been published on this respect.66
Friesen and co-workers reported the cross-coupling reaction between iodoglucal 62 and several metalated aromatics. Among them PhZnCl provided the corresponding adduct in 30 min (Scheme 36).67 The reaction required an excess of 4.0 equiv of organozinc chloride to go on completion and it can also be catalyzed by Pd(PPh3)4 although the reaction time was increased to 16 h.48
An organozinc compound was formed in situ from glucal 64 and made to react with iodides 107 in the presence of a catalytic amount of Pd2(dba)3·CHCl3 and the bulky tris-(o-tolyl)phosphine (Scheme 37).68 In the case of compound 108b a 5% of homocoupling byproduct was also obtained.
A Negishi cross-coupling between glycosyl halides and both alkyl and arylorganozinc compounds at room temperature was reported by Gagne and co-workers (Scheme 38).69 Good yields of the corresponding C-glycosides were obtained by using unsubstituted Pybox ligands. The reaction showed a good selectivity particularly with mannosyl halides 109 (Scheme 38).
With glucosyl halides similar amounts of α- and β-anomers were obtained in the case of alkylzinc reagents. With aryl derivatives, D-glucosyl bromide 112 provided β-anomers with excellent selectivity (Scheme 39).70 In all cases small amounts of the corresponding glycal were obtained. This technology was further applied to the total synthesis of salmochelins, a family of bacterial siderophores.71
O-Glycosides reacted with functionalized aryl Grignard derivatives in the presence of catalytic amounts of Pd(dppf)Cl2 to give C-arylglycosides having an α-configuration in excellent yields When the reaction was carried out with Ni(dppe)Cl2 as a catalyst only the formation of α-isomers was observed. Both cross-coupling reactions proceeded under mild conditions and with complete regio- and stereoselectivities (Scheme 40).72 The methodology was used for the synthesis of functionalized C-glycosides,73 including analogues of phenylalanine.74
6. OTHER REACTIONS
Maddaford and co-workers reported the direct coupling between peracetylated glycals and aryl boronic acid in the presence of catalytic palladium(II) acetate.75 The reaction took place through a syn addition of a σ-aryl-Pd complex to the glycal double bond followed by anti elimination of palladium(II) acetate, which close the catalytic cycle. The final result of the reaction is the C-arylation and migration of the double bond with loss of the acetoxy group at C-3 showing a Ferrier-type substitution reaction (Scheme 41). In some cases, particularly with aryl derivatives substituted with an electron-donor group, an open-chain byproduct was observed.76a Such a byproduct was also observed with furyl-3-boronic acid.76b
The reactivity of furanoid and pyranoid glycals toward Grignard reagents in the presence of a nickel catalyst was studied by Tingoli and co-workers.77 Only aryl Grignard reagents reacted, and with pyranoid systems open-chain adducts were obtained. On the other hand, with furanoid glycal 119 the adducts 120 were obtained in a similar reaction to that showed in Scheme 21 (Scheme 42).
The direct addition of organozinc compounds to glycals was reported by DuBois and co-workers.78 Both aryl and alkyl organozinc compounds led to the corresponding C-glycosides in good yields and stereoselectivities (Scheme 43).
The Pd-catalyzed opening of 123 with glycal 124 in the presence of Zn afforded 125, which was further employed in the formal synthesis of galtamycinone (Scheme 44).79
Glycosyl bromides 126 were coupled with alkenes in a radical-based reaction mediated by a readily available nickel catalyst. The reaction provided C-glycosides in good yields with alkenes bearing electron-deficient groups. Coupling with electron-rich alkenes was less successful. In general, the yields in the glucose series (Scheme 45) were slightly higher than those for the mannose series.80 The reaction required 2.0 equiv of Mn and it was carried out in THF at reflux.
Highly substituted chromans were prepared from carbohydrates through a domino process consisting of oxidative addition followed by two carbopalladation steps. The synthesis was completed by a cyclization to annelate the aromatic moiety (Scheme 46).81 The corresponding isochromans were also prepared by following a similar strategy.
7. CONCLUDING REMARKS
Cross-coupling reactions are one of the most efficient methods for creating carbon-carbon bonds. The past decades have witnessed remarkable developments of those reactions for preparing compounds of biological interest. Amongst these compounds, C-glycosides are of interest because of their stability against hydrolysis, which makes them excellent candidates for mimicking natural substrates and acting as competitive inhibitors of a variety of enzymes. With an eye toward biological relevance, cross-coupling reactions will undoubtedly continue to advance in a direction that benefits not only the organic chemical community but also biological and medicinal chemists.
ACKNOWLEDGEMENTS
Thanks are due to the Spanish Ministry of Science and Innovation (MICINN. Project CTQ2010-19606, Madrid, Spain), FEDER Program and the Government of Aragón (Group E-10, Zaragoza, Spain). E.M. and F.G.-B. thank MECD (FPU Program) and CSIC (JAE-Pre Program), respectively, for pre-doctoral grants.
References
1. (a) Metal-catalyzed cross-coupling reactions, F. Diederich and P. Stang (Eds.). Wiley-VCH: Weinheim, 1998; CrossRef (b) Cross-coupling reactions. A practical guide, N. Miyaura. Springer: Berlin, 2002; (c) Metal-catalyzed cross-coupling reactions, A. De Mejiere and F. Diederich (Eds.), Wiley-VCH: Weinheim, 2004, 2 Vol. set. 2 Ed., 2008; (d) Applications of transition metal catalysis in drug discovery and development: An industrial perspective M. L. Crawley and B. M. Trost (Eds.), John Wiley & sons: New York, 2012; (e) T. Takahashi and K. Kanno In Modern organonickel chemistry, Y. Tamaru (Ed.), Wiley-VCH: Weinheim, 2005; (f) Handbook of organopalladium chemistry for organic synthesis, E.-I. Negishi (Ed), Wiley Interscience: New York, 2002; (g) D. A. Alonso and C. Najera, In Science of Synthesis, 2010, 47, 439.
2. R. F. Heck and J. P. Nolley, J. Org. Chem., 1972, 37, 2320. For reviews see below. CrossRef
3. (a) N. Miyaura, K. Yamada, and A. Suzuki, Tetrahedron Lett., 1979, 20, 3437; CrossRef (b) N. Miyaura and A. Suzuki, Chem. Commun., 1979, 866. For reviews see below. CrossRef
4. D. Milstein and J. K. Stille, J. Am. Chem. Soc., 1978, 100, 3636. For reviews see below. CrossRef
5. A. O. King, N. Okukado, and E.-I. Negishi, Chem. Commun., 1977, 683. For reviews see below.
6. Y. Hatanaka and T. Hiyama, J. Org. Chem., 1988, 53, 918; CrossRef For a review see: S. E. Denmark and C. S. Regens, Acc. Chem. Res., 2008, 41, 1486. CrossRef
7. K. Sonogashira, J. Organomet. Chem., 2002, 653, 46; CrossRef For a review see: R. Chinchilla and C. Najera, Chem. Soc. Rev., 2011, 40, 5084. CrossRef
8. (a) F. Paul, J. Patt and J. F. Hartwig, J. Am. Chem. Soc., 1994, 116, 5969; CrossRef (b) A. S. Guram and S. L. Buchwald, J. Am. Chem. Soc., 1994, 116, 7901; CrossRef For a review see: D. S. Surry and S. L. Buchwald, Chem. Sci., 2011, 2, 27. CrossRef
9. K. Kikukawa and T. Matsuda, Chem. Lett., 1977, 159; CrossRef For a review see: J. G. Taylor, A. V. Moro, and C. R. D. Correia, Eur. J. Org. Chem., 2011, 1403. CrossRef
10. X.-F. Wu, P. Anbarasan, H. Neumann, and M. Beller, Angew. Chem. Int. Ed., 2010, 49, 9047; CrossRef For Nobel Lectures of two of the laureates see: (a) E.-I. Negishi, Angew. Chem. Int. Ed., 2011, 50, 6738; CrossRef (b) A. Suzuki, Angew. Chem. Int. Ed., 2011, 50, 6722. CrossRef
11. (a) N. Kishore, B. B. Mishra, V. Tripathi, and V. K. Tiwari, Trends Carbohydr. Res., 2011, 3, 1; (b) F. Nicotra, Topics Curr. Chem., 1997, 187, 55; CrossRef (c) W. Zou, Curr. Top. Med. Chem., 2005, 5, 1363; CrossRef (d) D. E. Levy and C. Tang, The Chemistry of C-glycosides. Tetrahedron Organic Chemistry Series. Vol. 13. Elsevier: Kidlington. 1995.
12. J. Stambasky, M. Hocek, and P. Kocovsky, Chem. Rev., 2009, 109, 6729. CrossRef
13. (a) R. J. Ferrier and J. O. Hober, Adv. Carbohydr. Chem., 2003, 58, 55; CrossRef (b) C. Taillefumier and Y. Chapleur, Chem. Rev., 2004, 104, 263; CrossRef (c) A. M. Gomez and J. C. Lopez, Carbohydr. Chem., 2009, 35, 289.
14. (a) M. H. D. Postema, Tetrahedron, 1992, 48, 8545; CrossRef (b) Y. G. Du, R. J. Lindhart, and I. R. Vlahov, Tetrahedron, 1998, 54, 9913; CrossRef (c) C. Jamarillo and S. Knapp, Synthesis, 1994, 1; CrossRef (d) D. Y. W. Lee and M. S. He, Curr. Top. Med. Chem., 2005, 5, 1333; CrossRef (e) T. Bililign, B. R. Griffith, and J. S. Thorson, Nat. Prod. Rep., 2005, 22, 742. CrossRef
15. K. W. Wellington and S. A. Benner, Nucleos. Nucleot., 2006, 25, 1309. CrossRef
16. (a) R. F. Heck, Org. React., 1982, 27, 345; (b) M. J. Fuchter, In Name reactions for homologations. Part I J. J. Li (Ed.), John Wiley & sons: Hoboken, 2009, pp. 2-32.
17. (a) A. DeMejiere and F. A. Meyer, Angew. Chem. Int. Ed., 1994, 33, 2379; CrossRef (b) I. P. Beletskaya and A. V. Cheprakov, Chem. Rev., 2000, 100, 3009; CrossRef (c) Y. Su and N. Jiao, Curr. Org. Chem., 2011, 15, 3362; CrossRef (d) D. McCartney and P. J. Guiry, Chem. Soc. Rev., 2011, 40, 5122; CrossRef (e) M. Shibasaki, T. Oshima, and W. Itano, In Science of Synthesis, 2011, 3, 483; (f) F.-X. Felpin, L. Nassar-Hardy, F. LeCallonnec, and E. Fouquet, Tetrahedron, 2011, 67, 2815; CrossRef (g) J. LeBras and J. Muzart, Chem. Rev., 2011, 111, 1170; CrossRef (h) D. Pan and N. Jiao, Synlett, 2010, 1577. CrossRef
18. I. Arai and G. D. Daves, J. Org. Chem., 1979, 44, 21. CrossRef
19. I. Arai and G. D. Daves, J. Org. Chem., 1978, 43, 4110. CrossRef
20. (a) S. Czernecki and V. Dechavanne, Can. J. Chem., 1983, 61, 533; CrossRef (b) V. Bellosta, S. Czernecki, D. Avenil, S. El-Bahis, and M. Gillier-Pandraud, Can. J. Chem., 1990, 68, 1364; CrossRef (c) S. Czernecki and F. Gruy, Tetrahedron Lett., 1981, 22, 437. CrossRef
21. R. N. Farr, R. A. Outte, J. C.-Y. Cheng, and G. D. Daves, Organometallics, 1990, 9, 3151. CrossRef
22. R. Benhaddou, S. Czernecki, and G. Ville, J. Org. Chem., 1992, 57, 4612. CrossRef
23. H.-C. Zhang and G. D. Daves, J. Org. Chem., 1992, 57, 4690. CrossRef
24. (a) J. C-Y. Chen, U. Hacksell, and G. D. Daves, J. Org. Chem., 1986, 51, 3093; CrossRef (b) I. Arai and G. D. Daves, J. Am. Chem. Soc., 1978, 100, 287. CrossRef
25. Z.-X. Wang, L. I. Wiebe, J. Balzarini, E. DeClercq, and E. E. Knaus, J. Org. Chem., 2000, 65, 9214. CrossRef
26. D. Hutter and S. A. Benner, J. Org. Chem., 2003, 68, 9839. CrossRef
27. R. Pohl, B. Klepetarova, and M. Hocek, J. Org. Chem., 2007, 72, 6797. CrossRef
28. H. H. Li and X.-S. Ye, Org. Biomol. Chem., 2009, 7, 3855. CrossRef
29. M. Lei, L. Gao, and J.-S. Yang, Tetrahedron Lett., 2009, 50 5135. CrossRef
30. A. L. L. Garcia and C. R. D. Correia, Tetrahedron Lett., 2003, 44, 1553. CrossRef
31. A. V. Moro, M. R. dos Santos, and C. R. D. Correia, Eur. J. Org. Chem., 2011, 7259. CrossRef
32. B. Schmidt, Chem. Commun., 2003, 1656. CrossRef
33. B. Schmidt and A. Biernat, Eur. J. Org. Chem., 2008, 5764. CrossRef
34. Y. Bai, M. Leow, J. Zeng, and X.-W. Liu, Org. Lett., 2011, 13, 5648. CrossRef
35. S. Xiang, S. Cai, J. Zeng, and X.-W. Liu, Org. Lett., 2011, 13, 4608. CrossRef
36. A. Tenaglia and F. Karl, Synlett, 1996, 327. CrossRef
37. J.-F. Nguefack, V. Bollit, and D. Sinou, J. Org. Chem., 1997, 62, 1341. CrossRef
38. (a) A. Suzuki, Pure Appl. Chem., 1991, 63, 419; CrossRef (b) N. Miyaura and A. Suzuki, Chem. Rev., 1995, 95, 2457; CrossRef (c) A. Suzuki, J. Organomet. Chem., 1999, 576, 147; CrossRef (d) S. Kotha, K. Lahiri, and D. Kashinath, Tetrahedron, 2002, 58, 9633. CrossRef
39. A. M. Gomez, G. A. Danelon, A. Pedregosa, S. Valverde, and J. C. Lopez, Chem. Commun., 2002, 2024. CrossRef
40. A. M. Gomez, A. Pedregosa, S. Valverde, and J. C. Lopez, Tetrahedron Lett., 2003, 44, 6111. CrossRef
41. A. Novoa, N. Pellegrini-Moise, S. Lamande-Langle, and Y. Chapleur, Tetrahedron Lett., 2009, 50, 6484. CrossRef
42. H.-T. T. Thien, A. Novoa, N. Pellegrini-Moise, F. Chretien, C. Didierjean, and Y. Chapleur, Eur. J. Org. Chem., 2011, 6939. CrossRef
43. C. R. Johnson and B. A. Johns, Synlett, 1997, 1406. CrossRef
44. J. T. Lin and B. K. Sorensen, Tetrahedron Lett., 2000, 41, 9213. CrossRef
45. W. Zhong, C. Moya, R. S. Jacobs, and R. D. Little, J. Org. Chem., 2008, 73, 7011. CrossRef
46. S. A. Look, W. FEnical, R. S. Jacobs, and J. Clardy, PNAS, 1986, 83, 6238. CrossRef
47. J. S. Potuzak and D. S. Tan, Tetrahedron Lett., 2004, 45, 1797. CrossRef
48. R. W. Friesen, R. W. Luo, and C. F. Sturino, Can. J. Chem., 1994, 72, 1262. CrossRef
49. H. Zhou, D. P. Danger, S. T. Dock, L. Hawley, S. G. Roller, C. D. Smith, and A. L. Handlon, ACS Med. Chem. Lett., 2010, 1, 19. CrossRef
50. S. Sakamaki, E. Kawanishi, S. Nomura, and T. Ishikawa, Tetrahedron, 2012, 68, 5744. CrossRef
51. I. Cobo, M. I. Matheu, S. Castillon, O. Boutureira, and B. G. Davis, Org. Lett., 2012, 14, 1728. CrossRef
52. P. J. Dransfield, P. M. Gore, I. Prokes, M. Shipman, and A. M. Z. Slawin, Org. Biomol. Chem., 2003, 2723. CrossRef
53. O. C. Xiong, L.-H. Zhang, and X.-S. Ye, Org. Lett., 2009, 11, 1709. CrossRef
54. (a) J. K. Stille, Angew. Chem. Int. Ed., 1986, 25, 508; CrossRef (b) V. Farina, V. Krishnamurthy, and W. J. Scott, Org. React., 1998, 50, 1; (c) T. N. Mitchell, Synthesis, 1992, 803. CrossRef
55. (a) A. L. Casado and P. Espinet, Organometallics, 1998, 17, 954; CrossRef (b) A. L. Casado and P. Espinet, J. Am. Chem. Soc., 1998, 120, 8978; CrossRef (c) P. Espinet and A. Echavarren, Angew. Chem. Int. Ed., 2004, 43, 4704. CrossRef
56. A. Gomez, A. Barrio, I. Amurrio, S. Valverde, S. Jarosa, and J. C. Lopez, Tetrahedron Lett., 2006, 47, 6243. CrossRef
57. R. W. Friesen and C. F. Sturino, J. Org. Chem., 1990, 55, 2572. CrossRef
58. J. C. Conway, C. J. Urch, P. Quayle, and J. Xu, Synlett, 2006, 776. CrossRef
59. B. Koo and F. E. McDonald, Org. Lett., 2005, 7, 3621. CrossRef
60. S. R. Dubbaka, P. Steunenberg, and P. Vogel, Synlett, 2004, 1235. CrossRef
61. P. Steunenberg, V. Seanneret, Y.-H. Zhu, and P. Vogel, Tetrahedron: Asymmetry, 2005, 16, 337. CrossRef
62. Y. Y. Belosludtsev, R. K. Bhatt, and J. R. Falck, Tetrahedron Lett., 1995, 36, 5881. CrossRef
63. S. E. Denmark and L. Neville, Org. Lett., 2000, 2, 3221. CrossRef
64. S. E. Denmark, T. Kobayashi, and C. S. Regens, Tetrahedron, 2010, 66, 4745. CrossRef
65. (a) E.-I. Negishi, Acc. Chem. Res., 1982, 12, 340; CrossRef (b) E.-I. Negishi, Q. Hu, Z. Huang, M. Qian, and G. Wang, Aldrichimica Acta, 2005, 38, 71; (c) E.-I. Negishi, Z. Huang, G. Wang, S. Mohan, C. Wang, and H. Hattori, Acc. Chem. Res., 2008, 41, 1474. CrossRef
66. (a) J. A. Casares, P. Espinet, B. Fuentes, and G. Salas, J. Am. Chem. Soc., 2007, 129, 3508; CrossRef (b) Q. Liu, Y. Lan, J. Liu, G. Li, Y.-D. Wu, and A. Lei, J. Am. Chem. Soc., 2009, 131, 10201. CrossRef
67. R. W. Friesen and R. W. Loo, J. Org. Chem., 1991, 56, 4821. CrossRef
68. M. Ousmer, V. Boucard, N. Lubin-Germain, J. Uziel, and J. Auge, Eur. J. Org. Chem., 2006, 1216. CrossRef
69. H. Gong, R. Sinisi, and M. R. Gagne, J. Am. Chem. Soc., 2007, 129, 1908. CrossRef
70. H. Gong and M. R. Gagne, J. Am. Chem. Soc., 2008, 130, 12177. CrossRef
71. X. Yu, Y. Dai, T. Yang, M. R. Gagne, and H. Gong, Tetrahedron, 2011, 67, 144. CrossRef
72. C. Moineau, V. Bolitt, and D. Sinou, J. Org. Chem., 1998, 63, 582. CrossRef
73. X. Xu, G. Fakha, and D. Sinou, Carbohydr. Lett., 2001, 4, 91.
74. X. Xu, G. Fakha, and D. Sinou, Tetrahedron, 2002, 58, 7539. CrossRef
75. J. Rammauth, O. Poulin, S. Rakhit, and S. P. Maddaford, Org. Lett., 2001, 3, 2013. CrossRef
76. (a) N. de la Figuera, P. Forns, J.-C. Fernandez, J. Fiol, D. Fernandez-Forner, and F. Albericio, Tetrahedron Lett., 2005, 46, 7271; CrossRef (b) M. Oshida, M. Ono, A. Nakazaki, and S. Kobayashi, Heterocycles, 2010, 80, 313. CrossRef
77. M. Tingoli, B. Panunzi, and F. Santacroce, Tetrahedron Lett., 1999, 40, 9329. CrossRef
78. D. P. Stenhuebel, J. J. Fleming, and J. Du Bois, Org. Lett., 2002, 4, 293. CrossRef
79. B. Apsel, J. A. Bender, M. Escobar, D. E. Kaelin, Jr., O. D. Lopez, and S. F. Martin, Tetrahedron Lett., 2003, 44, 1075. CrossRef
80. S. K. Readman, S. P. Marsden, and A. Hodgson, Synlett, 2000, 1628. CrossRef
81. M. Leibeling, D. C. Koester, M. Pawliczek, S. C. Schild, and D. B. Werz, Nat. Chem. Biol., 2010, 6, 199 CrossRef