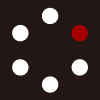
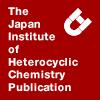
HETEROCYCLES
An International Journal for Reviews and Communications in Heterocyclic ChemistryWeb Edition ISSN: 1881-0942
Published online by The Japan Institute of Heterocyclic Chemistry
e-Journal
Full Text HTML
Received, 30th July, 2013, Accepted, 17th September, 2013, Published online, 30th September, 2013.
DOI: 10.3987/REV-13-779
■ Total Synthesis of Decahydrobenzo[d]xanthene Sesquiterpenoids Aureol, Strongylin A, and Stachyflin: Development of a New Strategy for the Construction of a Common Tetracyclic Core Structure
Tadashi Katoh*
Department of Synthetic Organic Chemistry, Tohoku Pharmaceutical University, 4-4-1 Komatsushima, Aoba-ku, Sendai 981-8558, Japan
Abstract
In this article, the total synthesis of rearranged sesquiterpenoid hydroquinones—aureol, strongylin A, and stachyflin—is reviewed with a particular focus on their methodology and strategy.CONTENTS
1. Introduction
2. Total synthesis of aureol
2.1. Total synthesis of (+)-aureol [Katoh et al.]
2.2. Total synthesis of (+)-aureol [George et al.]
2.3. Total synthesis of (−)-aureol [Marcos et al.]
3. Total synthesis of (+)-strongylin A [Katoh et al.]
4. Total synthesis of stachyflin
4.1. Total synthesis of (±)-stachyflin [Shionogi & Co., Ltd.]
4.2. Total synthesis of (+)-stachyflin [Katoh et al.]
5. Conclusion
1. INTRODUCTION
In recent years, a wide variety of natural products with unique structural features and attractive biological activities have been isolated from marine organism and microorganism.1 Several of these natural products have received considerable attention owing to their potential for use as new therapeutic agents.1 In most cases, however, further biological studies, including those focusing on structure–activity relationships (SARs), have been severely restricted probably because of the scarcity of samples and/or the structural diversity of the natural products derived from natural resources. As a consequence, the development of efficient and flexible methods for the synthesis of bioactive natural products and their analogues is highly desirable and worthwhile from the viewpoint of medicinal/pharmaceutical chemistry.2
(+)-Aureol (1, Figure 1) was originally isolated from the Caribbean sponge Smenospongia aurea by Faulkner et al. in 19803 and subsequently in 2000 from a different species of the Caribbean sponge Verongula gigantea.4 This marine natural product was found to exhibit selective antiproliferative activity against human tumor cells, including non-small-cell lung cancer A549 and colon adenocarcinoma HT-29 cells,5 and anti-influenza A virus activity.6 (+)-Strongylin A (2, Figure 1) was first isolated from the Caribbean sponge Strongylophora hartmani by Wright et al. in 19917 and subsequently from the Bahamian sponge Xestospongia wiedenmayeri by scientists from Schering–Plough Corporation (now Merck & Co., Inc.) in 1995.8 This marine natural product was found to exhibit antiproliferative activity against P388 murine leukemia cells (IC50 = 13 μg/mL) and antiviral activity against the human influenza A/PR/8/34 (H1N1) virus in vitro (IC50 = 6.5 μg/mL).7 (+)-Stachyflin (3, Figure 1) was isolated from the culture broth of Stachybotrys sp. RF-7260 by scientists from Shionogi & Co., Ltd., Japan, in 1997.9 This secondary metabolite was found to exhibit potent antiviral activity against the human influenza A/WSN/33 (H1N1) virus in vitro (IC50 = 3 nM) with a novel mechanism of action.9b The antiviral activity of 3 has been shown to result from interference with a low-pH-induced hemagglutinin conformation change, which is indispensable for the virus-cell membrane fusion process that occurs during influenza viral infection.10 Structurally, 1, 2, and 3 possess a novel tetracyclic
decahydrobenzo[d]xanthene skeleton (ABCD ring system) that consists of three asymmetric carbons at the C5, C9, and C10 positions, as depicted in structure 4 (Figure 1). Owing to the attractive biological properties and the unique structural features of these molecules, considerable attention has been devoted to the total synthesis of these decahydrobenzo[d]xanthene sesquiterpenoids (1–3).
In 2001, we embarked on a project directed at the total synthesis of this class of natural products. Our efforts culminated in the completion of the enantioselective total synthesis of 1 in 2002,11 2 in 2013,12 and 3 in 2010.13 The enantioselective total syntheses of 1 and its enantiomer (ent-1) were also reported by George et al. in 201214 and Marcos et al. in 2010,15 respectively. The total synthesis of racemic (±)-3 was presented by the Shionogi research group in 1998.16 Through this synthetic study, the anti-influenza A viral activity of (±)-3 was about half that of natural (+)-3.16 An enantioselective approach to the tetracyclic core structure (see structure 4) was published by Cramer et al. in 2010.17 In the present article, the total syntheses of 1–3 are reviewed with a particular focus on synthetic strategy.
2. TOTAL SYNTHESIS OF AUREOL
It is envisaged that 1 might be produced biogenetically by the acid-induced rearrangement of (+)-arenarol (5) (Scheme 1), which was first isolated from the marine sponge Dysidea arenaria by Schmitz et al. in 1984,18 and subsequently from a Fenestrasongia sp. by Faulkner et al. in 1985.19 This type of acid-induced rearrangement has been successfully applied to determine the absolute configuration of marine sesquiterpene quinones and hydroquinones.20,21 So far, to our knowledge, two examples of the acid-induced rearrangement of 5 have appeared in the literature (cf. Equations 1 and 2). In these examples, however, important synthetic chemical issues with respect to stereocontrol and efficiency were not considered. Thus, in 1990, Schmitz et al. described that the treatment of 5
with a large excess (~16 equiv) of p-toluenesulfonic acid (p-TsOH) in benzene at room temperature for 12 h followed by reflux for 30 min resulted in the rearrangement/cyclization of product 6 (ca. 60% yield),22 which corresponds to a stereoisomer of 1 with a trans-fused decalin ring system (Equation 1). On the other hand, in 1994, Capon et al. reported that the treatment of 5 in the same acid solution at reflux for 30 minutes provided 1 in 25% yield, along with a mixture of unidentified products (Equation 2).20a Considering these results, a more efficient and facile method for preparing 1 with a cis-fused decalin junction is highly required that it should provide a reliable and practical source for further biological evaluation.
2.1. Total Synthesis of (+)-Aureol [Katoh et al.]
2.1.1. Synthetic Strategy
We reported the first total synthesis of 1 in 200211c as well as its improved synthesis in 2003.11b Our retrosynthetic plan for the improved synthesis is outlined in Scheme 2. The key feature of this plan is the acid-induced rearrangement/cyclization of (−)-neoavarol (7) to produce target molecule 1 in one step. We envisioned that this rearrangement/cyclization event would proceed stereoselectively to install the requisite cis-fused decalin junction (see 7 → [I → II → III] → 1 in Scheme 4). The advanced key intermediate 7 would be readily derived from (−)-neoavarone (8) by the reduction of the quinone moiety. Intermediate 8 would be formed by the strategic salcomine oxidation23 of phenol 9, which is accessible from methyl ether 10. Intermediate 10 should, in turn, be derived by the
stereocontrolled reductive alkylation of (+)-5-methyl-Wieland–Miescher ketone (11)24 with 2-methoxybenzyl bromide (12)25 by applying protocols previously described in the literature.26
2.1.2. Total Synthesis
Initially, we pursued the synthesis of key intermediate 7 [(−)-neoavarol], as shown in Scheme 3. The stereocontrolled reductive alkylation of (+)-5-methyl-Wieland–Miescher ketone (11)24 with 2-methoxybenzyl bromide (12)25 by applying protocolsreductive alkylation of 1124 (>99% ee) with 1225, readily prepared from commercially available 2-methoxybenzyl alcohol, provided the expected coupling product 13 as a single diastereomer in 74% yield. The subsequent Wittig methylenation27 of 13 produced exo-olefin 14 in 86% yield. To form the C8 stereogenic center, the ethylene acetal moiety in 14 was first removed by acid treatment (97% yield),28 and the resulting ketone 15 was subjected to hydrogenation, forming the desired product 16 (80% yield) and its C8 epimer (13% yield) after separation by silica-gel column chromatography. The Wittig methylenation of 16 (quantitative yield) followed by the deprotection of the O-methyl group in the resulting exo-olefin 1029 produced phenol 9 (92% yield). To directly construct the quinone system, 9 was
subjected to salcomine oxidation,23 and 8 was formed in 91% yield. Finally, the reduction of 8 with NaBH4 provided 7 in 86% yield.
After obtaining the key intermediate 7, the crucial acid-induced rearrangement/cyclization was investigated as shown in Scheme 4. Thus, the treatment of 7 with BF3·Et2O (5 equiv) in CH2Cl2 from −50 to −5 °C for 5 h resulted in the formation of 1 in excellent yield (93%). This total synthesis was accomplished with an overall yield of 33.0% in 9 steps from starting material 11.
This cascade reaction proceeded smoothly and cleanly in a complete stereoselective manner. This can be elaborated via the mechanism shown in Scheme 4. An initial coordination–activation between the Lewis acid and the C4 olefinic double bond in 7 results in the formation of the first intermediate carbocation I, which is transformed into the second intermediate carbocation II by the migration of the C5 methyl group to the C4 carbocation center. Then, intermediate II undergoes a 1,2-hydride shift from the C10 position to the C5 carbocation center on the α-face of the molecule to form the third intermediate carbocation III. Finally, the C10 carbocation center in this intermediate is trapped by the C2’ hydroxy group in the aromatic moiety to form, after the protonolysis of the C–BF3 bond, the desired cyclized product 1. We believe that this cascade sequence proceeds in a stepwise manner under kinetically controlled conditions.
2.2. Total Synthesis of (+)-Aureol [George et al.]
2.2.1. Synthetic Strategy
In 2012, George et al. reported the second total synthesis of 1.14 Their synthetic plan is illustrated in Scheme 5. Target molecule 1 should be produced from intermediate 17 by acid-induced ether cyclization, presumably involving an intermediate carbocation (see structure IV in Scheme 7). This type of cyclization was previously reported by Marcos et al.15 in their total synthesis of unnatural (−)-aureol (ent-1) (vide infra, see section 2.3). Intermediate 17 would be formed by the addition of aryllithium 19 (accessible from known aryl bromide 2030) to aldehyde 18 followed by deoxygenation and deprotection. Intermediate 18 would be obtained from acetate 21 by the one-carbon dehomologation of the side chain. Intermediate 21 would be derived from tertiary alcohol 22 via a biomimetic sequence of 1,2-hydride and methyl shifts. Intermediate 22 would, in turn, be formed by the reduction and the monoprotection of commercially available (+)-sclareolide (23).
2.2.2. Total Synthesis
As shown in Scheme 6, the synthesis of intermediate 17, a precursor of the essential acid-induced ether cyclization, was carried out starting from 23 (>99% ee). The reduction of 23 using LiAlH4 generated a diol that was selectively protected at the primary hydroxyl group using Ac2O in pyridine to produce monoacetate 22 in 84% yield in two steps. The first crucial step was the BF3·Et2O-induced rearrangement of 22, which occurred via stereospecific 1,2-hydride and methyl shifts to form the desired product 21 as a single stereoisomer in 70% yield. The removal of the acetyl group from 21 produced alcohol 24 in 83% yield. Compound 24 was further converted to key intermediate 18 via a one-carbon dehomologation sequence involving dehydration using the Grieco–Sharpless protocol31 (67% yield in two steps) and the subsequent oxidative cleavage of the resulting terminal alkene 25 (45% yield in two steps). The second crucial coupling reaction between 18 with aryllithium 19 (see Scheme 5) was achieved by an initial bromine/lithium exchange of aryl bromide 2030 followed by the reaction with 18 from −78 °C to room temperature. The desired coupling product 26 was obtained as a mixture of epimeric alcohols, which, without separation, was then reduced under the Birch conditions to generate
deoxygenated product 27 with an overall yield of 78% from 18. The removal of the two O-TBS protecting groups from 27 produced hydroquinone 17 in 86% yield.
After obtaining the key intermediate 17, the final acid-induced ether cyclization step required to complete the synthesis was performed, as shown in Scheme 7. Thus, the treatment of 17 with BF3·Et2O (4.5 equiv) in CH2Cl2 from −60 to −20 °C for 3 h resulted in the formation of target 1 in 66% yield. In this reaction, the intermediate carbocation IV would be involved. This total synthesis was accomplished with an overall yield of 6.7% in 12 steps from starting material 23.
2.3. Total Synthesis of (−)-Aureol [Marcos et al.]
2.3.1. Synthetic Strategy
In 2010, Marcos et al. reported the total synthesis of unnatural (−)-aureol (ent-1).15 Their retrosynthetic plan is shown in Scheme 8. The first crucial step is expected to be the acid-induced ether cyclization of hydroquinone ent-17 via an intermediate carbocation (see structure ent-IV in Scheme 11). Intermediate ent-17 would be derived from quinone 28 by reduction with Raney®-nickel. An alternative essential step could be the Barton radical decarboxylation32/p-benzoquinone addition sequence to form intermediate 28 (29 + 30 → 28). This type of radical coupling reaction was originally explored by Theodorakis et al. in their total synthesis of marine sesquiterpenoid quinones and hydroquinones.33 Intermediate 29, in turn, would be derived from carboxylic acid 31, which is accessible from commercially available ent-halimic acid (32).
2.3.2. Total Synthesis
First, intermediate 31 was prepared starting from ent-halimic acid methyl ester (33), as shown in Scheme 9. The degradation of the side chain of 33 was achieved by OsO4 oxidation followed by reaction with Pb(OAc)4 to form the desired ketone 3434 in 94% yield in two steps. The subsequent Wittig methylenation of 34 (87% yield) and the olefin isomerization of the resulting exo-olefin 35 furnished endo-olefin 36 (99% yield). The C18 methyl ester function in 36 was efficiently converted to the C18 methyl group in 39 via a three-step sequence including LiAlH4 reduction (97% yield), the tetra-n-propylammonium perruthenate (TPAP) oxidation of the resulting alcohol 37 (97% yield), and the Wolff–Kishner reduction of the resulting aldehyde 38 (90% yield). The chemoselective epoxidation of the side-chain double bond in 39 (98% yield) using m-chloroperoxybenzoic acid (mCPBA) followed by the oxidative cleavage of the resulting epoxide by treatment with H5IO6 in H2O/THF produced aldehyde 40 (94% yield). Compound 40 was further converted to alcohol ent-24 via a four-step sequence including LiAlH4 reduction (99% yield), acetylation of the resulting alcohol 41 (99% yield), isomerization of the olefinic double bond in the resulting acetate 42 with HI (97% yield), and removal of the acetyl group (98% yield). Eventually, the twofold oxidation of ent-24 using pyridinium dichromate (PDC) provided the requisite carboxylic acid 31 (50% overall yield based on recovery of the starting material) via aldehyde 43.
After obtaining intermediate 31, the synthesis of hydroquinone ent-17, the key precursor of ent-1, was carried out as shown in Scheme 10. Treatment of 31 with 2-mercaptopyridine N-oxide in the presence of N,N’-dicyclohexylcarbodiimide (DCC)32 resulted in the corresponding 2-thiopyridon-1-yl ester 29. The crucial installation of the quinone moiety was performed by the light-induced decarboxylation of 29 (halogen lamp, 500 W) in the presence of p-benzoquinone (30), which formed the expected quinone addition product 28 in 65% yield from 31. The subsequent reduction of 28 with Raney®-nickel in EtOH provided ent-17 in 99% yield.
With the key precursor ent-17 synthesized, the final crucial step was examined as shown in Scheme 11. Thus, the treatment of ent-17 with BF3·Et2O (4.3 equiv) in CH2Cl2 from −50 to −5 °C for 2 h resulted in the formation of target (−)-aureol (ent-1) in 60% yield via the intermediate carbocation ent-IV. This total synthesis produced an overall yield of 10.3% in 19 steps from starting material 33.
3. TOTAL SYNTHESIS OF (+)-STRONGYLIN A [Katoh et al.]
3.1. Synthetic Strategy
Recently, we accomplished the first total synthesis of naturally occurring (+)-strongylin A (2), which led to the determination of the absolute configuration of natural 2.12 Our retrosynthetic plan is outlined in Scheme 12. The key element of this approach is the acid-induced dehydroxylation/rearrangement/ cyclization of tertiary alcohol 44 to form the target molecule in one step. We believed that this cascade reaction would stereoselectively proceed to construct the requisite cis-fused decalin ring junction (see 44 → [V → VI → VII] → 2 in Scheme 14). The second crucial step was considered to be a coupling reaction of trans-decalin 46 (accessible from alcohol 47) with aryllithium 48 (accessible from known trioxybenzene derivative 4935) to form intermediate 45, which could be converted to 44 by deprotection and functional group manipulation. Intermediate 47 would be formed by the stereoselective hydrogenation of exo-olefin 50, which is accessible from known trans-decalone 51.36 As a starting material, 51 was readily prepared from 11 in two steps by applying the method reported by Smith et al.36
3.2. Total Synthesis
In Scheme 13, we initially pursued the synthesis of intermediate 44, a precursor of the key cascad reaction, starting from 5136 (>99% ee). The protection of the hydroxy group in 51 followed by the Wittig methylenation of the resulting triethylsilyl (TES) ether 52 and the deprotection of the TES group in the resulting exo-olefin 53 provided the desired alcohol 50 in 69% yield in three steps. To establish the C8 stereogenic center, 50 was subjected to hydroxyl-group-directed hydrogenation using Crabtree’s catalyst {[Ir(COD)(PCy3)(py)]+[PF6]−}37 (2.5 mol%), which produced the requisite product 47 with complete stereoselectivity in almost quantitative yield (98%). The oxidation of 47 with TPAP then generated decalin aldehyde 46 in 94% yield.
The crucial coupling reaction between the decalin and aromatic portions was efficiently achieved by site-selective lithiation at the C4 position in 49 (see Scheme 12) using t-BuLi in THF at 0 °C followed by the treatment of aryllithium 48, generated in situ with aldehyde 46 at 0 °C to room temperature for 1 h. The expected coupling product 54 was obtained in excellent yield (94%) as an inseparable mixture of epimeric alcohols (ca. 4:1). The hydroxyl group of 54 was removed by applying the Barton–McCombie procedure,38 and the desired deoxygenated product 45 was formed with an overall yield of 78% via methyl xanthate 55. The exposure of 45 to concentrated hydrochloric acid in refluxing EtOH caused the simultaneous deprotection of the acetonide and ethylene acetal moieties, resulting in the formation of the desired ketone 56 in 71% yield. Finally, the reaction of 56 with MeMgBr (5 equiv) in THF from 0 °C to room temperature for 1 h, formed the requisite intermediate 44 in 70% yield as an inseparable mixture of epimeric alcohols (ca. 1.7:1).
With the key intermediate 44 in hand, we then investigated the crucial acid-induced cascade of dehydroxylation/rearrangement/ cyclization to complete the projected synthesis (Scheme 14). The desired cascade event was successfully achieved by treating 44 with excess of BF3·Et2O (10 equiv) in CH2Cl2 from −78 to 0 °C for 3 h, which resulted in the formation of 2 as a single stereoisomer in high yield (84%). The optical rotation of synthetic 2 {[α]23D = +83.3} was in good agreement with that of natural 2 {[α]20D = +72}, verifying the absolute configuration of 2. We believe that this cascade sequence would involve intermediate carbocations such as V, VI, and VII in a manner similar to that described in section 2.1 (see 7 → [I → II → III] → 1 in Scheme 4). This total synthesis was accomplished with an overall yield of 19.0% in 11 steps from starting material 11.
4. TOTAL SYNTHESIS OF STACHYFLIN
4.1. Total Synthesis of (±)-Stachyflin [Shionogi & Co., Ltd.]
4.1.1. Synthetic Strategy
In 1998, scientists from the Shionogi research group reported the first total synthesis of racemic (±)-3.16 Through their synthetic study, they discovered that the anti-influenza A virus activity of (±)-3 is approximately half that of natural (+)-3.16 Their retrosynthetic plan for (±)-3 is illustrated in Scheme 15. The first crucial transformation in this contemplated scheme is expected to be the construction of the tetracyclic intermediate 57 (ABCD ring system) from the tricyclic intermediate 59 (BCD ring system) via enone 58 (see 59 → 58 → 57). Hydrogenation of 58 would stereoselectively produce the desired cis-fused decalin (AB ring) due to the steric hindrance caused by the C9 methyl group (see 58 → 57). Advanced key intermediate 57 would be converted to target (±)-3 via lactam (E ring) formation. The second critical step is believed to involve the iodoetherification of intermediate 60 to construct tricyclic intermediate 59. Intermediate 60 would be derived from intermediate 61 by functional group manipulation. Intermediate 61, in turn, would be formed by the coupling reaction of the D-ring portion 62 [accessible from commercially available 3,5-dihydroxybenzoic acid (64)] with the B-ring portion 63.
4.4.2. Total Synthesis
As shown in Scheme 16, the synthesis of intermediate 62, the coupling partner of 63, was carried out starting from 65, which was pr epared from 64 in two steps. Thus, the chemoselective reduction of the C4 methyl ester function in 65 using NaBH4 provided the corresponding triol 66, which was subjected to acetonide formation followed by methyl etherification to generate acetonide 67 with an overall yield of 61% from 65. Compound 67 was then converted to the requisite intermediate 62 with an overall yield of 74% via a three-step procedure including the removal of the acetonide moiety, the selective O-benzylation of the resulting diol 68, and the pyridinium chlorochromate (PCC) oxidation of the resulting alcohol 69.
Scheme 17 shows the synthesis of trimethylsilyl enol ether 63 and alkylzinc reagent 7239, both of which are necessary for the following synthesis of key intermediate 59 (see Scheme 18). Compounds 63 and 72 were prepared from 2,3-dimethylcyclohexanone (70) and alkyl iodide 71, respectively (the yields were not described in the literature16).
Key intermediate 59 was synthesized as shown in Scheme 18. After the dimethylacetal formation of 62, Noyori’s aldol condensation40 of the resulting dimethyl acetal 73 with silyl enol ether 63 (see Scheme 17) was achieved to produce the expected coupling product 61 as a 1:1 diastereomixture. The subsequent deprotection of the benzyl group in 61 and the removal of the benzylic methoxy group from the resulting hemiacetal 74 formed deoxygenated hemiacetal 75 with an overall yield of 65% from 62. Compound 75 was then converted to phenolic alkene 60, a precursor for the key iodoetherification step, via a four-step sequence including the protection of the masked phenolic hydroxy group in 75 as its tert-butyldiphenylsilyl (TBDPS) ether (81% yield), the enol triflate formation of the resulting ketone 76 using Comins’ reagent41 (82% yield), the palladium-catalyzed coupling reaction42 of the resulting enol triflate 77 with alkylzinc reagent 72 (cf. Scheme 17) (85% yield), and the deprotection of the O-TBDPS group in the resulting coupling product 78 (91% yield). The crucial iodoetherification of 60 was efficiently achieved by treatment with I2 in THF in the presence of propylene oxide as an HI scavenger. The desired cyclization product 59 was formed in high yield (87%).
Completion of the total synthesis of (±)-3 is outlined in Scheme 19. The β-elimination of HI from 59 afforded alkene 79 (92% yield), which was then subjected to hydroboration and subsequent PCC oxidation to form ketone 80 with an overall yield of 45%. After the deprotection of the ethylene acetal moiety in 80 (90% yield), the resulting diketone 81 was converted to enone 58 with an overall yield of 75% via a three-step sequence including intramolecular aldol cyclization, the dehydration of the cyclized aldol product, and the base-induced isomerization of the resulting mixture of α,β- and β,γ-unsaturated ketones to the thermodynamically more stable enone 58. The critical stereoselective hydrogenation of 58 was best achieved under the optimized conditions [H2 (1 atm), 5% Rh/C, acetone]. The desired cis-fused decalin 82 was formed in 52% yield as the major stereoisomer (cis/trans 1.6:1). After the construction of the cis-fused decalin system, the regioselective introduction of a methyl group at the C4 position over the C2 position in 82 was performed by
the methylation of the thermodynamically more stable trimethylsilyl enol ether of 83 to form geminal dimethyl ketone 83 in 75% yield in two steps.
In the last stage of the synthesis, γ-lactam (E ring) formation was investigated as follows.43
The bromination of the C2’ position of the aromatic ring in 83 generated bromide 84 in 76% yield based on the recovery of the undesired regioisomer (the C4’-brominated product) and dibromide. These byproducts were efficiently reduced to reusable compound 83 by treatment with Pd(PPh3)4 and HCO2Na in DMF.44 The subsequent cyanation of 84 with CuCN produced nitrile 57 in 80% yield. The hydrogenation of the nitrile function of 57 delivered ammonium salt 85, which was then exposed to a MeOH solution of NaOMe at room temperature, resulting in the formation of γ-lactam 86 in 87% yield in two steps. In the hydrogenation step, the carbonyl group in 57 was simultaneously reduced to form the requisite β-alcohol in a highly stereoselective manner. Finally, the removal of the phenolic O-methyl moiety from 86 (n-BuSLi, HMPA)29 generated target (±)-3 in 83% yield. This total synthesis was completed with an overall yield of 0.9% in 31 steps from starting material 64.
4.2. Total Synthesis of (−)-Stachyflin [Katoh et al.]
4.2.1. Synthetic Strategy
We accomplished the first enantioselective total synthesis of naturally occurring 3 in 2010.13 Our retrosynthetic plan is outlined in Scheme 20. We envisioned that target molecule 3 would be derived from epoxide 87 through an acid-induced cascade reaction (see 87 → [VIII → IX → X] → 103a and 103b in Scheme 23), which was devised based on our previous studies described earlier.11 Key intermediate 87 would be prepared through the coupling reaction of 11 with bromide 88 (accessible from 64).
4.2.2. Total Synthesis
We initially pursued the synthesis of intermediate 88, the coupling partner of 11, starting from 64 (Scheme 21). The Kolbe–Schmitt reaction45 of 64 followed by methyl esterification provided dimethyl ester 65 in 64% yield in two steps. Compound 65 was then converted to acetonide 67 with an overall yield of 79% via methyl ester 89 by the selective reduction of the C4 methyl ester function to its corresponding hydroxymethyl congener followed by acetonide formation and methyl etherification. The subsequent regioselective bromination at C2 in 67 and cyanation with CuCN provided nitrile 90 in 66% yield in two steps. Isoindolinone 92 was formed with an overall yield of 98% from 90 by the hydrogenation [H2 (1 atm), PtO2, EtOH/CHCl3, rt] and subsequent lactamization of the resulting ammonium salt 91 following treatment with NaOMe in MeOH at room temperature. After the protection of the lactam amide moiety in 92 with a 3,4-dimethoxybenzyl (3,4DMB) group (78% yield), the resulting lactam 93 was transformed into bis-TBS ether 94 with an overall yield of 75% by the cleavage of the acetonide moiety and silylation of the liberated hydroxyl groups. Finally, the regioselective desilylation of 94 (48% aq. HF, MeCN, 0 °C) followed by the bromination of the resulting benzyl alcohol 95 formed the requisite intermediate 88 in 86% yield in two steps.
With the requisite intermediate 88 in hand, we next synthesized intermediate 87, the precursor of the key cascade reaction, as shown in
Scheme 22. Thus, the crucial reductive alkylation of 11 (>99% ee) with 88 under the Birch conditions proceeded smoothly and cleanly, forming the expected coupling product 96 in 76% yield as a single diastereomer. Cleavage of the TBS group in 96 followed by the Wittig methylenation of the resulting hemiacetal 97 produced exo-olefin 98 in 79% yield in two steps.
In order to establish the C8 stereochemistry, the ethyleneacetal moiety in 98 was first removed (98% yield), and the resulting ketone 99 was subjected to stereoselective hydrogenation, forming the desired product 100 in 96% yield as a single stereoisomer. The subsequent Wittig methylenation of 100 (74% yield) followed by the isomerization of double bond in the resulting olefin 101 provided endo-olefin 102 (quantitative yield). Epoxidation of 102 with mCPBA afforded the desired intermediate 87 in 86% yield as an inseparable mixture of α- and β-epoxides (7:1).
After synthesizing 87, our efforts were directed toward the completion of total synthesis of (+)-3. To this end, as shown in Scheme 23, the key acid-induced cascade epoxide-opening/rearrangement/cyclization of 87 (α-/β-epoxide, 7:1) was investigated under the conditions similar to those described above (see Scheme 4 in section 2.1 and Scheme 14 in section 3). The desired products 103a (C3 α-OH) and 103b (C3 β-OH) were obtained in 66% and 9% yields, respectively, after separation by silica-gel column chromatography. In this cascade reaction, intermediate carbocations such as VIII, IX, and X would be involved in a manner similar to that described in section 3.2 (see 44 → [V → VI → VII] → 2 in Scheme 14). The subsequent inversion of the configuration at C3 of 103a was achieved by Dess–Martin oxidation (94% yield) followed by the LiAlH(t-BuO)3 reduction of the resulting ketone 104 (96% yield). Finally, compound 103b was successfully converted to target (+)-3 by the removal of the N-3,4DMB group with PIFA46 (54% yield) and the cleavage of the O-methyl moiety29 in the resulting lactam 105 (80% yield). This total synthesis was accomplished with an overall yield of 1.6% in 27 steps from 64 or with an overall yield of 10.0% in 13 steps from 11.
5. CONCLUSION
In this article, the total synthesis of three biologically attractive sesquiterpenoid natural products—aureol (1), strongylin A (2), and stachyflin (3)—has been summarized with particular focus on their synthetic strategies. It is of great interest to look at each respective methodology devised for constructing the requisite tetracyclic skeleton (ABCD ring system) and the whole carbon framework by controlling the correct stereochemistry. The key element of our approach is the BF3·Et2O-induced sequential carbocation rearrangement/ether cyclization reaction to stereoselectively construct the desired tetracyclic core structure, comprising cis-fused AB- and BC-ring junctions, in one step (7 → 1 in Scheme 4, 44 → 2 in Scheme 14, and 87 → 103a,b in Scheme 23). These synthetic studies hold promise for preparing additional analogues of 1–3 with the aim of exploring their SARs, which will be useful for the development of novel therapeutic agents.
ACKNOWLEDGEMENTS
The author thanks the contributions of my colleagues whose names are described in the references. Our study was financially supported in part by a Grant-in-Aid Scientific Research on Priority Area (No. 17035073 and No. 18032065), a Grant-in-Aid Scientific Research (C) (No. 18590013 and No. 21590018), a Grant-in-Aid for High Technology Research Program at Private Universities (2005–2009), and a Grant-in-Aid for the Strategic Research Foundation Program at Private Universities (2010–2014) from the Ministry of Education, Culture, Sports, Science and Technology, Japan (MEXT).
References
1. For recent reviews, see: (a) J. W. Blunt, B. R. Copp, R. A. Keyzers, M. H. G. Munro, and M. R. Prinsep, Nat. Prod. Rep., 2013, 30, 237; CrossRef (b) K.-D. Feussner, K. Ragini, R. Kumar, K. M. Soapi, W. G. Aalbersberg, M. K. Harper, B. Carte, and C. M. Ireland, Nat. Prod. Rep., 2012, 29, 1424; CrossRef (c) I. Abraham, K. E. Sayed, Z.-S. Chen, and H. Guo, Mar. Drugs, 2012, 10, 2312; CrossRef (d) S. N. Sunassee and M. T. Davies-Coleman, Nat. Prod. Rep., 2012, 29, 513; CrossRef (e) W. H. Gerwick and B. S. Moore, Chem. Biol., 2012, 19, 85; CrossRef (f) J. W. Blunt, B. R. Copp, R. A. Keyzers, M. H. G. Munro, and M. R. Prinsep, Nat. Prod. Rep., 2012, 29, 144. CrossRef
2. For recent reviews, see: (a) M. Gordaliza, Mar. Drugs, 2012, 10, 358; CrossRef (b) J. C. Morris and A. J. Phillips, Nat. Prod. Rep., 2011, 28, 269. CrossRef
3. P. Djura, D. B. Stierle, B. Sullivan, D. J. Faulkner, E. Arnold, and J. Clardy, J. Org. Chem., 1980, 45, 1435. CrossRef
4. P. Ciminiello, C. Dell’Aversano, E. Fattorusso, S. Magno, and M. Pansini, J. Nat. Prod., 2000, 63, 263. CrossRef
5. R. E. Longley, O. J. McConnel, E. Essich, and D. Harmody, J. Nat. Prod., 1993, 56, 915. CrossRef
6. A. E. Wright, S. S. Cross, N. S. Burres, and F. Koehn (Harbor Branch Oceanographics Institution, Inc., USA), PCT WO 9112250 A1, August 22, 1991.
7. A. E. Wright, S. A. Rueth, and S. S. Cross, J. Nat. Prod., 1991, 54, 1108. CrossRef
8. S. J. Coval, M. A. Conover, R. Mierzwa, A. King, M. S. Puar, D. W. Phife, J.-K. Pai, R. E. Burrier, H.-S. Ahn, G. C. Boykow, M. Patel, and S. A. Pomponi, Bioorg. Med. Chem. Lett., 1995, 5, 605. CrossRef
9. (a) T. Kamigauchi, T. Fujiwara, H. Tani, Y. Kawamura, and I. Horibe (Shionogi & Co., Ltd., Japan), PCT WO 9711947 A1, April 3, 1997; (b) K. Minagawa, S. Kouzuki, J. Yoshimoto, Y. Kawamura, H. Tani, T. Iwata, Y. Terui, H. Nakai, S. Yagi, N. Hattori, T. Fujiwara, and T. Kamigauchi, J. Antibiot., 2002, 55, 155; CrossRef (c) K. Minagawa, S. Kouzuki, and T. Kamigauchi, J. Antibiot., 2002, 55, 165. CrossRef
10. (a) J. Yoshimoto, M. Kakui, H. Iwasaki, H. Sugimoto, T. Fujiwara, and N. Hattori, Microbiol. Immunol., 2000, 44, 677; (b) J. Yoshimoto, M. Kakui, H. Iwasaki, T. Fujiwara, H. Sugimoto, and N. Hattori, Arch. Virol., 1999, 144, 865. CrossRef
11. (a) J. Sakurai, T. Oguchi, K. Watanabe, H. Abe, S. Kanno, M. Ishikawa, and T. Katoh, Chem. Eur. J., 2008, 14, 829; CrossRef (b) A. Suzuki, M. Nakatani, M. Nakamura, K. Kawaguchi, M. Inoue, and T. Katoh, Synlett, 2003, 329; CrossRef (c) M. Nakamura, A. Suzuki, M. Nakatani, T. Fuchikami, M. Inoue, and T. Katoh, Tetrahedron Lett., 2002, 43, 6929. CrossRef
12. T. Kamishima, T. Kikuchi, and T. Katoh, Eur. J. Org. Chem., 2013, 4558. CrossRef
13. (a) J. Sakurai, T. Kikuchi, O. Takahashi, K. Watanabe, and T. Katoh, Eur. J. Org. Chem., 2011, 2948; CrossRef (b) K. Watanabe, J. Sakurai, H. Abe, and T. Katoh, Chem. Commun., 2010, 46, 4055; CrossRef (c) M. Nakatani, M. Nakamura, A. Suzuki, M. Inoue, and T. Katoh, Org. Lett., 2002, 4, 4055. CrossRef
14. K. K. W. Kuan, H. P. Pepper, W. M. Bloch, and J. H. George, Org. Lett., 2012, 14, 4710. CrossRef
15. I. S. Marcos, A. Conde, R. F. Moro, P. Basabe, D. Díez, and J. G. Urones, Tetrahedron, 2010, 66, 8280. CrossRef
16. T. Taishi, S. Takechi, and S. Mori, Tetrahedron Lett., 1998, 39, 4347. CrossRef
17. D. T. Ngoc, M. Albicker, L. Schneider, and N. Cramer, Org. Biomol. Chem., 2010, 8, 1781. CrossRef
18. F. J. Schmitz, V. Lakshmi, D. R. Powell, and D. van der Helm, J. Org. Chem., 1984, 49, 241. CrossRef
19. B. Carte, C. B. Rose, and D. J. Faulkner, J. Org. Chem., 1985, 50, 2785. CrossRef
20. (a) S. Urban and R. J. Capon, Aust. J. Chem., 1994, 47, 1023; CrossRef (b) S. Urban and R. J. Capon, J. Nat. Prod., 1992, 55, 1638; CrossRef (c) R. J. Capon, J. Nat. Prod., 1990, 53, 753. CrossRef
21. In 2001, Waldmann et al. reported an analogous rearrangement reaction in their total synthesis of nakijiquinones, novel marine sesquiterpenoid quinones. In this sutdy, the rearrangement products related to aureol (1) and its stereoisomer 6 were regarded as undesired and useless products; therefore, detailed information regarding the stereoselectivity, yield, and reaction conditions for this rearrangement was not given. See: P. Stahl, L. Kissau, R. Mazitschek, A. Huwe, P. Furet, A. Giannis, and H. Waldmann, J. Am. Chem. Soc., 2001, 123, 11586. CrossRef
22. V. Lakshmi, S. P. Gunasekera, F. J. Schmitz, X. Ji, and D. van der Helm, J. Org. Chem., 1990, 55, 4709. CrossRef
23. For representative examples of salcomine oxidation, see: (a) X. W. Liao, W. Liu, W. F. Dong, B. H. Guan, S. Z. Chen, and Z. Z. Liu, Tetrahedron, 2009, 65, 5709; CrossRef (b) S. Akai, K. Kakiguchi, Y. Nakamura, I. Kuriwaki, T. Dohi, S. Harada, O. Kubo, N. Morita, and Y. Kita, Angew. Chem. Int. Ed., 2007, 46, 7458; CrossRef (c) T. Katoh, M. Nakatani, S. Shikita, R. Sampe, A. Ishiwata, M. Nakamura, and S. Terashima, Org. Lett., 2001, 3, 2701; CrossRef (d) F. Miyata, S. Yoshida, T. Yamori, and T. Katoh, Heterocycles, 2001, 54, 619; CrossRef (e) N. Saito, Y. Obara, T. Aihara, S. Harada, Y. Shida, and A. Kubo, Tetrahedron, 1994, 50, 3915; CrossRef (f) K. Yoshida, S. Nakajima, T. Ohnuma, Y. Ban, M. Shibasaki, K. Aoe, and T. Date, J. Org. Chem., 1988, 53, 5355; CrossRef (g) T. Wakamatsu, T. Nishi, T. Ohnuma, and Y. Ban, Synth. Commun., 1984, 14, 1167. CrossRef
24. H. Hagiwara and H. Uda, J. Org. Chem., 1988, 53, 2308. CrossRef
25. J. L. Kelly, J. A. Linn, and J. W. T. Selway, J. Med. Chem., 1989, 32, 1757. CrossRef
26. his type of reductive alkylation, originally explored by Stork et al. in the 1960s (a representative study; see: G. Stork, P. Rosen, N. Goldman, R. V. Coombs, and J. Tsuji, J. Am. Chem. Soc., 1965, 87, 275), has been widely used as a critical step in the total synthesis of marine sesquiterpenoid quinones and hydroquinones. For examples; CrossRef see: (a) S. Poigny, M. Guyot, and M. Samadi, J. Org. Chem., 1998, 63, 5890; CrossRef (b) S. D. Bruner, H. S. Radeke, J. A. Tallarico, and M. L. Snapper, J. Org. Chem., 1995, 60, 1114. CrossRef
27. L. Fitjer and U. Quabeck, Synth. Commun., 1985, 15, 855. CrossRef
28. A. S. Sarma and P. Chattopadhyay, J. Org. Chem., 1982, 47, 1727. CrossRef
29. (a) M. Nakatani, M. Nakamura, A. Suzuki, M. Inoue, and T. Katoh, Org. Lett., 2002, 4, 4483; CrossRef (b) P. A. Aristoff, A. W. Harrison, and A. M. Huber, Tetrahedron Lett., 1984, 25, 3955; CrossRef (c) S. C. Welch and A. S. C. P. Rao, Tetrahedron Lett., 1977, 18, 505; CrossRef (d) G. I. Feutrill and R. N. Mirrington, Aust. J. Chem., 1972, 25, 1719; CrossRef (e) P. A. Bartlett and W. S. Johnson, Tetrahedron Lett., 1970, 11, 4459. CrossRef
30. J. P. Willis, K. A. Z. Gogins, and L. L. Miller, J. Org. Chem., 1981, 46, 3215. CrossRef
31. (a) G. Majetich, P. A. Grieco, and M. Nishizawa, J. Org. Chem., 1977, 42, 2327; CrossRef (b) K. B. Sharpless and M. W. Young, J. Org. Chem., 1975, 40, 947. CrossRef
32. (a) D. H. R. Barton and W. Sas, Tetrahedron, 1990, 46, 3419; CrossRef (b) D. H. R. Barton, B. Lacher, and S. Z. Zard, Tetrahedron, 1987, 43, 4321; CrossRef (c) D. H. R. Barton, D. Bridon, and S. Z. Zard, Tetrahedron, 1987, 43, 5307. CrossRef
33. (a) T. Ling, E. Poupon, E. J. Rueden, S. H. Kim, and E. A. Theodorakis, J. Am. Chem. Soc., 2002, 124, 12261; CrossRef (b) T. Ling, A. X. Xiang, and E. A. Theodorakis, Angew. Chem. Int. Ed., 1999, 38, 3089; CrossRef (c) T. Ling, E. Poupon, E. J. Rueden, and E. A. Theodorakis, Org. Lett., 2002, 4, 819. CrossRef
34. I. S. Marcos, F. A. Hernández, M. J. Sexmero, D. Díez, P. Basabe, A. B. Pedrero, N. García, F. Sanz, and J. G. Urones, Tetrahedron Lett., 2002, 43, 1243. CrossRef
35. A. Bernet and K. Seifert, Helv. Chim. Acta, 2006, 89, 784. CrossRef
36. A. B. Smith III, L. Kürti, A. H. Davulcu, and Y. S. Cho, Org. Process Res. Dev., 2007, 11, 19. CrossRef
37. R. H. Crabtree and M. W. Davis, J. Org. Chem., 1986, 51, 2655. CrossRef
38. (a) D. H. R. Barton and S. W. McCombie, J. Chem. Soc., Perkin Trans. 1, 1975, 1, 1574; CrossRef For representative synthetic applications, see: (b) T. Kikuchi, M. Mineta, J. Ohtaka, N. Matsumoto, and T. Katoh, Eur. J. Org. Chem., 2011, 5020; CrossRef (c) M. Inoue, W. Yokota, and T. Katoh, Synthesis, 2007, 622; CrossRef (d) T. Katoh, T. Izuhara, W. Yokota, M. Inoue, K. Watanabe, A. Nobeyama, and T. Suzuki, Tetrahedron, 2006, 62, 1590; CrossRef (e) M. Inoue, W. Yokota, M. G. Murugesh, T. Izuhara, and T. Katoh, Angew. Chem. Int. Ed., 2004, 43, 4207. CrossRef
39. K. Takai, T. Kakiuchi, and K. Utimoto, J. Org. Chem., 1994, 59, 2671. CrossRef
40. S. Murata, M. Suzuki, and R. Noyori, J. Am. Chem. Soc., 1980, 102, 3248. CrossRef
41. D. L. Comins and A. Dehghani, Tetrahedron Lett., 1992, 33, 6299. CrossRef
42. Y. Tamaru, H. Ochiai, T. Nakamura, and Z. Yoshida, Tetrahedron Lett., 1986, 27, 955. CrossRef
43. J. Wrobel, A. Dietrich, B. J. Gorham, and K. Sestanj, J. Org. Chem., 1990, 55, 2694. CrossRef
44. P. Helquist, Tetrahedron Lett., 1978, 19, 1913. CrossRef
45. C. M. Harris, J. J. Kibby, J. R. Fehlner, A. B. Raabe, T. A. Barber, and T. M. Harris, J. Am. Chem. Soc., 1979, 101, 437.. CrossRef
46. (a) K. Watanabe, H. Shibata, Y. Imai, and T. Katoh, Heterocycles, 2012, 84, 1355; CrossRef (b) K. Watanabe and T. Katoh, Tetrahedron Lett., 2011, 52, 5395. CrossRef