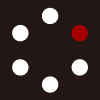
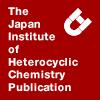
HETEROCYCLES
An International Journal for Reviews and Communications in Heterocyclic ChemistryWeb Edition ISSN: 1881-0942
Published online by The Japan Institute of Heterocyclic Chemistry
e-Journal
Full Text HTML
Received, 2nd July, 2012, Accepted, 10th September, 2012, Published online, 20th September, 2012.
DOI: 10.3987/COM-12-S(N)86
■ Computational Investigation of a Photo-Switchable Single-Molecule Magnet Based on a Porphyrin Terbium Double-Decker Complex
Tomoko Inose, Daisuke Tanaka, and Takuji Ogawa*
Department of Chemistry, Graduate School of Science, Osaka University, 1-1 Matikaneyama-cho, Toyonaka, Osaka 560-0043, Japan
Abstract
Based on the finding that switching of single molecule magnetic property (SMM) is possible for porphyrin-TbIII double-decker complexes by protonation and deprotonation, we performed a theoretical consideration of photo induced switching of SMM in a porphyrin-quinone dyad by density functional theory (DFT)-based calculations. In the excited state, proton (or hydrogen) transferred structure (*por-QH) is only 1.26 Kcal/mol higher in energy than that of the starting structure (*porH-Q), which suggests that the proton (or hydrogen) transfer in the excited state is possible.Single-molecule magnets (SMMs) are a unique class of compounds that show superparamagnetic behavior on the single-molecule scale. They have been attracting much attention because of the potential they have for applications such as nanoscale memory devices or quantum computers.1 In particular, a family of phthalocyanine (Pc)-based TbIII double-decker compounds is known to have a high blocking temperature (~50 K) and these compounds are therefore regarded as the most promising set of molecules.2
We have previously reported the synthesis of protonated and deprotonated (anionic) forms of porphyrin–TbIII double-decker complexes. Their X-ray crystallographic structures were determined for the first time, and their SMM properties were studied.3 The essential coordination structure of a porphyrin double-decker complex is similar to that of the Pc analog. The most significant finding is that only the deprotonated form of the porphyrin double-decker complex showed single-molecule magnetism (Figure 1). This result indicates that the SMM property can be controlled by merely manipulating a single proton. Although the reason has not been clarified yet, high symmetry for the local environment of the Tb ion is important to show SMM property for the double-decker complexes.
Here, the porphyrin–quinone dyad shown in Figure 2 is proposed for achieving photo-stimulated SMM switching by forming a double-decker complex with Tb. In this dyad, the porphyrin is an electron donor and the quinone works as an acceptor, therefore photo-induced electron transfer from the porphyrin to the quinone occurs.4 In addition, there is the possibility that proton-coupled electron transfer (PCET) or excited-state intramolecular proton transfer (ESIPT) can result.5,6 If ESIPT is possible in the porphyrin–quinone dyad, SMM switching in the corresponding Tb double-decker complex can be expected. Although there are several experimental and theoretical papers reporting on ESIPT,6d its occurrence in the molecular system proposed here has so far been unconfirmed.
In this work, density functional theory (DFT)-based calculations were performed relating to the molecular system shown in Figure 2. The structure in ground states were optimized with DFT, and those in the excited state were performed using CI-Singles (CIS) calculations to reveal their S1 states with the keywords “opt ucis=(nstates=6.root=1)”. All calculations were performed using the Gaussian09
software package,7 and the graphics were generated with the help of the Gauss View software.
Five structures were optimized with (U)B3LYP/6-311G++, and shown in Figure 2, along with the corresponding energies. PorH-Q and por-QH indicate the ground states before and after ESIPT, respectively, and *porH-Q and *por-QH represent the excited states before and after ESIPT, respectively. Por···H···Q indicates the transition state between porH-Q and por-QH, which was obtained by the QST2 procedure in the Gaussian09 program package, and optimized for the transition state. The assignment of the transition structure was confirmed by the presence of one imaginary harmonic frequency in the force calculation.
Figure 3 shows the calculated optimized structure of the transition state. As shown, the N–H distance is 2.20 Å, the O–H distance is 1.01 Å, and the angle formed by N–H–O is 157°. This structure indicates that the transition state is more similar to por-QH than to the starting porH-Q.
Figure 4 illustrates the highest occupied molecular orbitals (HOMOs) and lowest unoccupied molecular orbitals (LUMOs) of the four states of the porphyrin–quinone dyads. Figure 4(a) shows the ground state before ESIPT (porH-Q). The HOMO can be assigned to the π-orbital located mostly on the porphyrin of the dyad, and the LUMO to the π-orbital located entirely on the quinone of the dyad. Figure 4(b) shows the excited state before ESIPT (*porH-Q). Here, the HOMO π-orbital was located entirely on the porphyrin of the dyad, but the LUMO π-orbital was partially placed on the quinone. Figures 4(c) and (d) show the ground and excited states after ESIPT has occurred (por-QH and *por-QH). The electron was spread over the entire porphyrin–quinone dyad.
The most probable process in the excited state is PCET, which will possibly generate neutral diradical centered at the nitrogen atom in the porphyrin moiety and the oxygen atom of the quinone part as marked “*” in the molecular structure in Figure 2. However, in this calculation, there is no clear difference between α spin orbitals from those of β spin in *por-QH nor por-QH, which means that they are closed shell structures. The most reasonable electronic structure deduced from the calculations can be depicted as the resonance structures shown in Figure 5. The resonance structure has double bonding character between the porphyrin ring and the quinone ring, which is consistent with the calculated dihedral angles shown in Table 1. In porH-Q, two planes cross almost perpendicularly to each other mainly because of the sterical hindrance. In contrast, ground state structure of por-QH has smaller dihedral angle, which is the consequence of the double bonding character of the bond between the porphyrin ring and the quinone ring.
In the ground state, the structure after the ESIPT (por-QH) is 59.7 kcal/mol less stable than the starting structure (porH-Q). However, in the excited state, the energy difference decreases to only 1.26 kcal/mol, which can be easily overcome by thermal activation at room temperature. The activation energy for the backward reaction in the ground state was estimated from the energy of the transition state (por···H···Q) to be 1.90 kcal/mol.
The above energy estimations mean that although the por-QH is less stable than the starting porH-Q, the PCET can readily proceed as a result of photo-excitation, and the ground state of por-QH has some life-time because of the activation energy of 1.90 kcal/mol. If this activation energy can be increased by molecular design, the por-QH state could have a long enough life-time to be used as an optical memory molecule. Moreover, in our previous research, using infrared (IR) spectroscopy, it was shown that the strength of the N–H bond of the porphyrin double-decker was weaker than that of a single porphyrin molecule.3 In combination, these results suggest that SMM switching in the solid state can be expected for this TbIII–porphyrin double-decker complex using a porphyrin–quinone dyad.
ACKNOWLEGEMENTS
This work was supported in part by Grants-in-Aid for Scientific Research (Nos. 23310076 and 24651142) and for Scientific Research on Innovative Areas “Emergence in Chemistry” from the Ministry of Education, Culture, Science, Sports, and Technology of Japan (No. 20111012). Computations were performed at the Research Center for Computational Science, Okazaki, Japan.
References
1. (a) K. Katoh, H. Isshiki, T. Komeda, and M. Yamashita, Chem. Asian J., 2012, 7, 1154; CrossRef (b) J. R. Friedman and M. P. Sarachik, In Annual Review of Condensed Matter Physics, Vol. 1, ed. by J. S. Langer, 2010, Vol. 1, p. 109; (c) K. Katoh, Y. Yoshida, M. Yamashita, H. Miyasaka, B. K. Breedlove, T. Kajiwara, S. Takaishi, N. Ishikawa, H. Isshiki, Y. F. Zhang, T. Komeda, M. Yamagishi, and J. Takeya, J. Am. Chem. Soc., 2009, 131, 9967; CrossRef (d) D. Stepanenko, M. Trif, and D. Loss, Inorg. Chim. Acta, 2008, 361, 3740; CrossRef (e) P. Seneor, A. Bernand-Mantel, and F. Petroff, J. Phys.: Condens Matter, 2007, 19, 165222. CrossRef
2. (a) S. Sakaue, A. Fuyuhiro, T. Fukuda, and N. Ishikawa, Chem. Commun., 2012, 48, 5337; CrossRef (b) K. Katoh, T. Kajiwara, M. Nakano, Y. Nakazawa, W. Wernsdorfer, N. Ishikawa, B. K. Breedlove, and M. Yamashita, Chem. Eur. J., 2011, 17, 117; CrossRef (c) K. Katoh, H. Isshiki, T. Komeda, and M. Yamashita, Coord. Chem. Rev., 2011, 255, 2124; CrossRef (d) T. Fukuda, W. Kuroda, and N. Ishikawa, Chem. Commun., 2011, 47, 11686; CrossRef (e) N. Ishikawa, In Functional Phthalocyanine Molecular Materials, ed. by J. Jiang, 2010, Vol. 135, p. 211; CrossRef (f) R. Sessoli and A. K. Powell, Coord. Chem. Rev., 2009, 253, 2328; CrossRef (g) N. Ishikawa, Polyhedron, 2007, 26, 2147; CrossRef (h) N. Ishikawa, M. Sugita, T. Ishikawa, S. Koshihara, and Y. Kaizu, J. Am. Chem. Soc., 2003, 125, 8694. CrossRef
3. D. Tanaka, T. Inose, H. Tanaka, S. Lee, N. Ishikawa, and T. Ogawa, Chem. Commun., 2012, 7796. CrossRef
4. (a) I. Tabushi, N. Koga, and M. Yanagita, Tetrahedron Lett., 1979, 257; CrossRef (b) A. D. Joran, B. A. Leland, P. M. Felker, A. H. Zewail, J. J. Hopfield, and P. B. Dervan, Nature, 1987, 327, 508; CrossRef (c) A. Osuka, K. Maruyama, and S. Hirayama, Tetrahedron, 1989, 45, 4815. CrossRef
5. B. K. Paul and N. Guchhait, Comput. Theor. Chem., 2011, 972, 1. CrossRef
6. (a) M. Y. Okamura, M. L. Paddock, M. S. Graige, and G. Feher, Biochim. Biophys. Acta, 2000, 1458, 148; CrossRef (b) J. Stubbe, D. G. Nocera, C. S. Yee, and M. C. Y. Chang, Chem. Rev., 2003, 103, 2167; CrossRef (c) M. H. V. Huynh and T. J. Meyer, Chem. Rev., 2007, 107, 5004; CrossRef (d) P. A. Karr, M. E. Zandler, M. Beck, J. D. Jaeger, A. L. McCarty, P. M. Smith, and F. D'Souza, J. Mol. Struct.:Theochem, 2006, 765, 91; CrossRef (e) J. J. Warren and J. M. Mayer, J. Am. Chem. Soc., 2011, 133, 8544; CrossRef (f) S. Hammes-Schiffer, J. Phys. Chem. Lett., 2011, 2, 1410. CrossRef
7. M. J. Frisch, G. W. Trucks, H. B. Schlegel, G. E. Scuseria, M. A. Robb, J. R. Cheeseman, G. Scalmani, V. Barone, B. Mennucci, G. A. Petersson, H. Nakatsuji, M. Caricato, X. Li, H. P. Hratchian, A. F. Izmaylov, J. Bloino, G. Zheng, J. L. Sonnenberg, M. Hada, M. Ehara, K. Toyota, R. Fukuda, J. Hasegawa, M. Ishida, T. Nakajima, Y. Honda, O. Kitao, H. Nakai, T. Vreven, J. A. Montgomery, Jr., J. E. Peralta, F. Ogliaro, M. Bearpark, J. J. Heyd, E. Brothers, K. N. Kudin, V. N. Staroverov, R. Kobayashi, J. Normand, K. Raghavachari, A. Rendell, J. C. Burant, S. S. Iyengar, J. Tomasi, M. Cossi, N. Rega, J. M. Millam, M. Klene, J. E. Knox, J. B. Cross, V. Bakken, C. Adamo, J. Jaramillo, R. Gomperts, R. E. Stratmann, O. Yazyev, A. J. Austin, R. Cammi, C. Pomelli, J. W. Ochterski, R. L. Martin, K. Morokuma, V. G. Zakrzewski, G. A. Voth, P. Salvador, J. J. Dannenberg, S. Dapprich, A. D. Daniels, Ö. Farkas, J. B. Foresman, J. V. Ortiz, J. Cioslowski, and D. J. Fox, Gaussian 09, Revision C.1, Gaussian, Inc., Wallingford, CT, 2009.