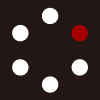
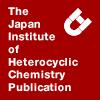
HETEROCYCLES
An International Journal for Reviews and Communications in Heterocyclic ChemistryWeb Edition ISSN: 1881-0942
Published online by The Japan Institute of Heterocyclic Chemistry
e-Journal
Full Text HTML
Received, 9th September, 2014, Accepted, 9th December, 2014, Published online, 7th January, 2015.
DOI: 10.3987/REV-14-806
■ Synthesis, Biological Activities, and Tautomerism of 4-Quinolones and Related Compounds
Yoshihisa Kurasawa* and Kenji Sasaki
Department of Organic Chemistry, School of Pharmacy, Iwaki Meisei University, 5-5-1 Chuodai Iino, Iwaki City, Fukushima 970-8551, Japan
Abstract
This review describes the synthesis, biological activities, and tautomerism of various 4-quinolones together with related compounds including 4-oxopyridazino[3,4-b]quinoxalines (bioisostere of 4-quinolones).CONTENTS
1. Introduction
2. Synthesis and Biological Activities of 3-Substituted 4-Oxopyridazino[3,4-b]quinoxalines as Bioiso-
stere of 4-Quinolones
Section 2-1 to Section 2-9
3. Some Antimalarial Agents Including New Quinolones in 2000-2010
4. Synthesis of Antimalarial 4-Quinolones
Section 4-1 to Section 4-7
5. Diverse 4-Quinolones with Various Biological Activities
Section 5-1 to Section 5-3
6. Tautomerism of Quinolones: Tautomeric Structures between 4-Oxo and 4-Hydroxy Forms in Solution
Section 6-1 to Section 6-9
7. Postscript
1. INTRODUCTION
We have reported the reaction of quinoxaline 1-oxides (Figure 1) providing various condensed quinoxalines,1 which include isoxazolo[2,3-a]quinoxalines,2-6 pyrrolo[1,2-a]quinoxalines,2-6 pyridazino- [3,4-b]quinoxalines,7-9 pyrazolo[3,4-b]quinoxalines,10 1,2-diazepino[3,4-b]quinoxalines,11,12 1,5,6-benz- oxadiazocino[3,4-b]quinoxalines,13 1,2,4-triazolo[4,3,2-o,p][1,3]diazocino[4,5-b]quinoxalines,14 1,3,4- thiadiazino[3,4-b]quinoxalines,15-18 and 1,3,4-oxadiazino[3,4-b]quinoxalines.17,19 In continuation of the above works, we found the conversion of some pyridazino[3,4-b]quinoxalines into the 4-oxopyridazino- [3,4-b]quinoxaline-3-carboxylic acids (1)20 (Scheme 1), which would be regarded as bioisostere of antibacterial 4-quinolones as well as cinoxacin21-23 and pyrido[2,3-b]quinoxaline-3-carboxylic acids24 (Scheme 1). Namely, the structure hybridization of nalidixic acid,25,26 cinoxacin, and pyrido[2,3-b]- quinoxaline-3-carboxylic acids led to the synthesis of compounds 1. However, compounds 1 did not exhibit excellent antibacterial activity so that C3-modified compounds (2-15) (Scheme 2) were elaborated to search for more biologically active compounds.
On the other hand, the modification of the C3-carboxyl into C3-carboxamide group in antibacterial rosoxacin has been known to provide the antiherpetic 4-quinolone-3-carboxamide (Scheme 3).27 Consequently, we synthesized 2- or 3-substituted 4-quinolones aiming at some biological activities such as antimicrobial and/or antimalarial activities (Figure 2). Furthermore, when the N1 of the 4-quinolones has no substituent such as the alkyl or aryl group, there would be the tautomeric equilibria between the 4-oxo and 4-hydroxy forms for 4-quinolones. In such cases, the tautomer assignment of the 4-quinolones is an important matter for the chemists or biologists dealing with sundry quinolone derivatives. This review describes the synthesis, biological activities, and tautomeric equilibria of the above 4-quinolones and related compounds.
2. SYNTHESIS AND BIOLOGICAL ACTIVITIES OF 3-SUBSTITUTED 4-OXOPYRIDAZINO- [3,4-b]QUINOXALINES AS BIOISOSTERE OF 4-QUINOLONES
2-1. Synthesis of 1,4-Dihydro-4-oxopyridazino[3,4-b]quinoxaline-3-carboxylic Acids
The reaction of the 3-chloroquinoxaline 1-oxides (16a,b) with alkylhydrazines gave the 3-(1-alkylhydr- azino)quinoxaline 1-oxides (17a-d), whose 1,3-dipolar cycloaddition reaction with acetylenedicarbox- ylate afforded the 1,5-dihydropyridazino[3,4-b]quinoxaline-3,4-dicarboxylates (18a-d) (Scheme 4) pre- sumably via intermediates (1’-5’) (Scheme 5). 20 The tautomeric structure of compounds 18a-d was as- signed as the 1,5-dihydro form in solution and solid state (Scheme 6),28 while dihydropyridazine29 and dihydrocinnolines30 have been known to exist as the 1,4-dihydro form (Figure 3). The oxidation of 18a-d with nitrous acid provided the 4-hydroxy derivatives (19a-d), whose reaction with DBU or KOH produced the 4-oxopyridazino[3,4-b]quinoxaline-3-carboxylates (20a-d) or 4-oxopyridazino[3,4-b]quino- xaline-3-carboxylic acids 1a-d, respectively. The 3-carboxylic acids 1a-d and 3-carboxylates 20a-d showed weak antimicrobial activities to bacteria [Mycobacterium ranae, Staphylococcus aureus, Staphy-
lococcus epidermis, Klebsiella pneumoniae: minimum inhibitory concentration (MIC) 12.5-25.0 ppm (µg/mL); Bacillus subtilis: (MIC) 2.0-15.6 ppm] and fungi [Candida albicans: (MIC) 12.5-50.0 ppm]. 31 Accordingly, the modification of the C3-substituent was planned for compounds 1, and the (4-oxo- pyridazino[3,4-b]quinoxalin-3-yl)alkylcarboxylates (2, 3) were synthesized in the next section 2-2.
2-2. Synthesis of (1,4-Dihydro-4-oxopyridazino[3,4-b]quinoxalin-3-yl)acetates and 4-(1,4-Dihydro- 4-oxopyridazino[3,4-b]quinoxalin-3-yl)butanoates
The reaction of the quinoxaline 1-oxides 17a,b with acetone-1,3-dicarboxylate in sulfuric acid/acetic acid gave the (1,5-dihydropyridazino[3,4-b]quinoxalin-3-yl)acetates (21a,b), whose oxidation with nitrous acid or MCPBA afforded the 1,4-dihydro-4-hydroxy derivatives (22a,b), respectively (Scheme 7).32 The oxidation in this step resulted in good yields [compound 22a (80%); compound 21b to compound 2b (82%)] (compound 22b was not isolated). Subsequent reaction of compound 22a with a base provided the (1,4-dihydro-4-oxopyridazino[3,4-b]quinoxalin-3-yl)acetate 2a. In the meantime, the reaction of the quinoxaline 1-oxides 17a,b with cyclohexane-1,3-dione produced the quinoxalino[2,3-c]cinnolines (23a,b), whose oxidation with NBS in water/acetic acid furnished the 4-(1,4-dihydro-4-oxo- pyridazino[3,4-b]quinoxalin-3-yl)butanoic acids (3a,b) (79-90%), respectively. Compounds 3a,b were converted into the ethyl esters (3c,d), respectively. The conversion of the quinoxaline 1-oxides 17a,b into compounds 21a,b and 23a,b would proceed via intermediates (6’ and 7’), and the oxidation of compounds 23a,b formed compounds 3a,b presumably via an intermediate (8’) (Figure 4). Compounds 3c,d exhibited weak antibacterial activity to Bacillus subtilis (MIC, 7.8 ppm), while compounds 2a,b and 3a,b represented no antibacterial activity to the above bacteria.31
2-3. Synthesis of 3-Methylpyridazino[3,4-b]quinoxalin-4(1H)-ones and 3-Ethylpyridazino[3,4-b]- quinoxalin-4(1H)-ones
The reaction of the quinoxaline 1-oxides 17a-d with acetylacetone gave the 4-acetyl-1,5-dihydropyrida-
zino[3,4-b]quinoxalines (24a-d) presumably via intermediates (9’ and 10’) (Scheme 8).28 The oxidation of compounds 24a, 24b,c, or 24d with N-bromosuccinimide/water, sodium bromate, or selenium dioxide afforded the 3-methylpyridazino[3,4-b]quinoxalin-4(1H)-ones (4a-d) presumably via an intermediate (11’).32 On the other hand, the reaction of the quinoxaline 1-oxides 17a-d with propanal provided the hydrazone derivatives (25a-d), whose reaction with 2-chloroacrylonitrile produced the racemic 1,2-diazepino[3,4-b]quinoxalines (26a-d), respectively (Scheme 9), presumably via intermediates (12’- 15’) (Figure 5). 32 The initial 1,3-dipolar cycloaddition reaction giving an intermediate 12’, subsequent ring cleavage of the isoxazolidine ring to an intermediate 13’, and then recyclization would furnish compounds 26a-d. The oxidative ring transformation of compounds 26a-d with selenium dioxide resulted in the formation of the 3-ethylpyridazino[3,4-b]quinoxalin-4(1H)-ones (5a-d), respectively, presumably via intermediates (16’-19’) (Figure 5). Compounds 4a,c showed antibacterial, antifungal, and algicidal activities to various microbials in the concentration of 2-8 ppm (Table 1).33
The tautomeric structure of compounds 26a-d (Scheme 9) and an intermediate 16’ (Figure 5) exhibited above was supported by the NOE spectral data in DMSO-d6 as shown in Figure 6.10,11 Their tautomeric structures were found to depend on the nature of the C5-substituent. Namely, a series of compounds 26
existed as the 2,3-dihydro-4-hydroxy form 26-A or 2,3,4,6-tetrahydro-4-oxo form 26-B when the C5-sub-
stituent was cyano or alkoxyl group, respectively. The crucial NOE was observed in the tautomer 26f-A (NOE between C4-OH and C3-H) or in the tautomer 26g-B (NOE between N6-H and C7-H).
2-4. Synthesis of Pyridazino[3,4-b]quinoxalin-4(1H)-ones
Compounds 1a-d have the β-keto acid moiety (A) as shown in Figure 7, and hence compounds 1a-d are seemed to undergo the decarboxylation easily, producing compounds (6a,b) (Schemes 10 and 11). However, the presence of the α-hydrazono moiety (B) would suppress the decarboxylation by the electron-donating nature of the N1-nitrogen (C). Accordingly, the alternate methods for the synthesis of compounds 6a,b were devised as displayed in Schemes 10 and 11.
The reaction of the quinoxaline 1-oxide 17a with diethyl ethoxymethylenemalonate gave the 1,5-dihy- dropyridazino[3,4-b]quinoxaline-4-carboxylate (27), whose oxidation with nitrous acid provided the 4-hydroxypyridazino[3,4-b]quinoxaline-4-carboxylate (28) (Scheme 10).34 The reaction of compound
28 with potassium hydroxide afforded 7-chloro-1-methylpyridazino[3,4-b]quinoxalin-4(1H)-one (6a). On the other hand, the reaction of the quinoxaline 1-oxide 17a with diethyl ethoxymethylenemalonate in acetic acid produced the pyridazino[3,4-b]quinoxaline-4,4-dicarboxylate (29a) presumably via an inter- mediate (20’). The reaction of compound 29a with a base resulted in hydrolysis and decarboxylation to furnish compound 27. 1-Methylpyridazino[3,4-b]quinoxalin-4(1H)-one (6b) was synthesized by a similar method via the oxidation of the 4-acetyl-1,5-dihydropyridazino[3,4-b]quinoxaline (30) (Scheme 11). Compound 6a showed antifungal activity to Candida albicans, Aspergillus flavus, Aspergillus fumigatus (MIC, 4 ppm), Candida krusei (MIC, 2 ppm), Trichophyton mentagrophytes (MIC, 1 ppm), and Trichophyton rubrum (MIC, 0.5 ppm).33
2-5. Synthesis of 3-Halogenopyridazino[3,4-b]quinoxalin-4(1H)-ones
The reaction of the pyridazino[3,4-b]quinoxaline-4,4-dicarboxylates 29a,b with NCS or NBS gave the 3-halogenopyridazino[3,4-b]quinoxaline-4,4-dicarboxylates (31a-d), whose reaction with hydrazine hydrate provided the 3-halogenopyridazino[3,4-b]quinoxaline-4-carboxylates (32a-d), respectively (Scheme 12).35 The oxidation of compounds 32a-d with nitrous acid afforded the derivatives (33a-d), whose reaction with hydrazine hydrate produced the 4-hydroxy derivatives (34a-d), respectively. The oxidation of compounds 34a-d with NCS or NBS in water furnished the 3-halogenopyridazino- [3,4-b]quinoxalin-4(1H)-ones (7a,b) or (8a,b), respectively. Compound 7a (R1 = Cl) exhibited antibacterial activity to Aureobacididium pullulans, Bacillus subtilis, Cladosporium cladosporioides, Chaetonium globosum, Micrococcus luteus, and Staphylococcus aureus (MIC, 2 ppm) and algicidal activity to Ankistrodesmus falcatus and Selenastrum capricorunutum (MIC, 2 ppm).33 Moreover, com- pounds 7a,b and 8a,b represented antifungal activity to Candida albicans, Candida krusei, Trichophyton mentagrophytes, and Trichophyton rubrum (MIC, 0.5-4 ppm).33
2-6. Synthesis of 3-Trifluoromethylpyridazino[3,4-b]quinoxalin-4(1H)-ones
The reaction of the quinoxaline 1-oxides 17a,b with ethyl 4,4,4-trifluoro-3-oxobutanoate gave the 3-trifluoromethylpyridazino[3,4-b]quinoxaline-4-carboxylates (35a,b), whose oxidation with nitrous acid produced the 4-hydroxy-3-trifluoromethylpyridazino[3,4-b]quinoxaline-4-carboxylates (36a,b), respec- tively (Scheme 13).36 The reaction of compound 36a with a base afforded the 4-hydroxy derivative (37a, 90%), while the reaction of compound 36b with a base produced the 4-hydroxy (37b, 74%) and 4-oxo (9b, 20%) derivatives. The oxidation of compounds 37a,b with sodium bromate furnished compounds 9a,b, respectively. Compound 9a showed antibacterial activity to Bacillus subtilis, Chaetonium globosum, Micrococcus luteus, and Staphylococcus aureus (MIC, 2 ppm) and algicidal activity to Ankistrodesmus falcatus (MIC, 2 ppm).33
2-7. Synthesis of 3-Aminopyridazino[3,4-b]quinoxalin-4(1H)-ones and Related Compounds
The reaction of the 4-oxopyridazino[3,4-b]quinoxaline-3-carboxylate (20e) with an excess of hydrazine hydrate furnished the 4-hydroxypyridazino[3,4-b]quinoxaline-3-carbohydrazide (38) presumably via intermediates (21’-24’) accompanied by reduction and replacement (Scheme 14).37 The reaction of com-
pound 38 with nitrous acid gave the 4-oxopyridazino[3,4-b]quinoxaline-3-carbonylazide (39), which was converted into the 3-aminopyridazino[3,4-b]quinoxalin-4(1H)-one (10a) and N-(pyridazino[3,4-b]- quinoxalin-3-yl)carbamates (10b,c). Compound 38 was also transformed into the hydrazones (40a-d), and the heating of compound 38 in DMSO produced the pyrazolo[3’,4’:5,6]pyridazino[3,4-b]quinoxalin- 3(5H)-one (41) presumably via an intermediate (25’). The in vitro screening data was not obtained for compounds shown in Schemes 14 and 15.
2-8. Synthesis of 3-Heteroarylpyridazino[3,4-b]quinoxalin-4(1H)-ones
The reaction of the quinoxaline 1-oxide 17a with arylcarbaldehydes provided the hydrazones (42a-c), whose reaction with 2-chloroacrylonitrile afforded the 1,2-diazepino[3,4-b]quinoxaline-5-carbonitriles (43a-c), respectively (Scheme 16).38 The reaction of compounds 43a-c with selenium dioxide/water re-
sulted in the oxidation and ring transformation to produce the 3-hetroarylpyridazino[3,4-b]quino- xalin-4(1H)-ones (11-13), respectively. Compounds 11 and 12 were converted into the 5’-substituted derivatives (14 and 15), respectively. Concerning the formation of compounds 43a-c and 11-13, the reaction would proceed via similar intermediates shown in Figure 5 (section 2-3).
2-9. Synthesis of 7-Methylsulfonylpyridazino[3,4-b]quinoxalin-4(1H)-ones
The reaction of the 7-chloropyridazino[3,4-b]quinoxalin-4(1H)-ones 4a, 6a, and 8a with sodium methylthiolate furnished the 7-methylsulfanyl derivatives (44a-c), whose oxidation with MCPBA gave the 7-methylsulfonylpyridazino[3,4-b]quinoxalin-4(1H)-ones (45a-c), respectively (Scheme 17).39 Com- pounds 44a-c and 45b,c represented in vitro antimicrobial activities to bacteria and algae (Table 2).39 Compounds 44a-c showed antibacterial activity to Micrococcus luteus and Chaetonium globosum (MIC, 2 ppm), and compounds 45b,c exhibited antibacterial activity to Bacillus subtilis and Staphylococcus aureus (MIC, 2-3.9 ppm). In addition, compounds 44a, 45b, and 45c represented algicidal activity to Ankistrodesmus falcatus (MIC, 2 ppm).
The 4H-1,3,4-thiadiazino[5,6-b]quinoxalines were found to exist as the N5-ammonium form in TFA- d1,40 while the 4H-1,3,4-oxadiazino[5,6-b]quinoxalines predominated as the zwitterionic form of the N10-ammonium form in DMSO-d6 (Figure 8).41 On the other hand, compounds 44a,b were found to be
present in the N5-ammonium form in TFA-d1.39 The structures of these ammonium forms were assigned by the comparison of the carbon chemical shifts in CDCl3 with those in TFA-d1.
3. SOME ANTIMALARIAL AGENTS INCLUDING NEW QUINOLONES IN 2000-2010
Quinine and chloroquine have been known as antimalarial agents for the clinical use, and many research groups have synthesized various heterocyclic compounds as candidates of antimalarial agents, which are described in a review by Jain et al.42 In 2000-2010, there have been many reports on the synthesis of the antimalarial agents: for example, pyridinium salt dimers [N,N’-hexamethylenebis(1-aryl-4-carbamoyl- pyridinium bromides), (IC50, 10 nM); 1,1’-(1,12-dodecanediyl)bis(4-butylaminocarbonyl)pyridinium bromides, (ED50, 8.2 mg/kg)],43-45 carbocyclic nucleosides (2-fluoroaristeromycin and 2-fluoronor- aristeromycin, inhibiting Plasmodium falciparum S-adenosyl-L-homocysteine hydrolase),46-49 1,4- disubstituted thiosemicarbazides and 1,3-diarylureas {(4-alkyl-1-[1-(3-chlorobenzyl)-2-pyridone-3-car- bonyl]thiosemicarbazides), 1-aryl-3-(quinolin-6-yl)ureas, 3-(3-amidinophenyl)-1-arylureas, inhibiting the wild-type and resistant mutant Plasmodium falciparum dihydrofolate reductase}50-52 (Figure 9). Some of
new quinolones were also found to possess antimalarial activity,53,54 and the synthetic approaches were carried out to search for a new type of antimalarial 4-quinolones as described below. The literatures of antibacterial new quinolones are not introduced in this review, since there have already been numerous theses, reviews, and monographs on the antibacterial new (and old) quinolones.
4. SYNTHESIS OF ANTIMALARIAL 4-QUINOLONES
4-1. Synthesis of 4-Quinolone-3-carboxylates with Heteroaryl Moiety in N1-Side Chain
The structural conversion of new quinolone antibacterials was carried out as shown in Scheme 18.55 Namely, ordinary new quinolone antibacterials have the N1-(alkyl or aryl) moiety, C3-carboxyl group, 4-oxo group, C6-fluoro group, and C7-basic moiety in the quinoline ring. Although the C7-basic moiety acts an important role for the antibacterial activity, compounds (46-52) produced by shifting the basic moiety to N1-side chain from C7-position of new quinolones were found to show antimalarial activity.
The reaction of the 4-hydroxyquinoline-3-carboxylates (53, 54) with bromoacetate effected the alkylation in the N1 of quinoline to give the (4-quinolon-1-yl)acetates (55, 56), whose reaction with pyridine- carbaldehydes provided the 1-[1-alkoxycarbonyl-2-(2-, 3- and 4-pyridyl)vinyl]-4-quinolone-3-carbox- ylates (46, 47), respectively (Scheme 19). 55 The 1-[1-ethoxycarbonyl-2-(2-furyl, 2-thienyl, and 3- thienyl)vinyl]-4-quinolone-3-carboxylates (52) and 1-[2-(2-, 3- and 4-pyridyl)vinyl]-4-quinolone-3-car- boxylic acids (48-51) were also synthesized from compounds 55, 56 and 46, 47, respectively. The in vitro screening to antimalarial activity exhibited that compound 47a (R1 = H, R2 = Et, R3 = 4-pyridyl) represented the IC50 of 8.2 µM to Plasmodium falciparum and the IC50 of above 24 µM to mouse FM3A cell F28-7 strain (chemotherapeutic coefficient: 2.9). The IC50 of other compounds to Plasmodium falciparum was above 21 µM.
4-2. Synthesis of 4-Quinolones with Pyridyl Moiety in C3-Side Chain
In continuation of the works exhibited in the above section 4-1, the pyridyl moiety was shifted to the C3-side chain, and the 7-chloro atom was excluded. Compounds (57a,b) obtained from the correspond-
ing 4-hydroxyquinoline-3-carboxylates were converted into the 4-quinolone-3-carbohydrazides (58a,b), whose reaction with pyridine-2-, 3-, and 4-carbaldehydes provided the hydrazones (59a-f) (Scheme 20).56 The reaction of compound 58a (R = CF3) with nitrous acid precipitated an azide intermediate (26’), and then heating of the reaction mixture gave the 1,3-bis(4-quinolon-3-yl)urea (60) presumably via intermedi-
ates (27’ and 28’). Compounds 58b and 59a,b (a: R = CF3, 2-pyridyl; b: R = F, 2-pyridyl) represented antimalarial activity to chloroquine-sensitive Plasmodium falciparum (IC50, 8.7, 6.2, and 3.3 µM, respectively).
4-3. Synthesis of 1-(1,2,4-Triazolylmethyl)-4-quinolone-3-carboxylic Acids
The reaction of compound 55 with hydrazine hydrate gave the 1-hydrazinocarbonylmethyl-4-quinolone- 3-carboxylate (61), whose alkaline hydrolysis provided the 1-hydrazinocarbonylmethyl-4-quinolone-3- carboxylic acid (62) (Scheme 21).57 Compound 62 was transformed into the 1-[(4-amino-1,2,4-triazol- 3-yl)methyl]-4-quinolone-3-carboxylic acid (64) via the 1-(1-ethoxyethylidene)hydrazinocarbonylmethyl-
4-quinolone-3-carboxylic acid (63). The reaction of compound 64 with arylaldehydes afforded the 1-[(4-arylmethyleneamino-1,2,4-triazol-3-yl)methyl]-4-quinolone-3-carboxylic acids (65). Although compounds 64 and 65 did not show antimalarial activity, the 4-quinolones having the (thio)semicarbazide moiety at the 3-position of compound 64 exhibited antimalarial activity as described in the next section 4-4.
4-4. Synthesis of 1-(4-Quinolon-3-ylcarbonyl)-4-phenylsemicarbazide and -4-phenylthiosemicarbaz- ide
Compound 61 was converted into the 1-(1-ethoxyethylidene)hydrazinocarbonylmethyl derivative (66), whose reaction with hydrazine hydrate in the presence of DBU furnished the 1-(4-amino-1,2,4-triazol-3-
ylmethyl)-4-quinolone-3-carbohydrazide (67) (Scheme 22).57 The reaction of compound 67 with phenyl isocyanate or phenyl isothiocyanate gave the 1-(4-quinolon-3-ylcarbonyl)-4-phenylsemicarbazide (68a)
or -4-phenylthiosemicarbazide (68b), respectively. Compounds 68a,b represented in vitro antimalarial activity to chloroquine-resistant Plasmodium falciparum (IC50: 68a, 3.89 µM; 68b, 3.91 µM). Diverse 1,4-disubstituted thiosemicarbazides (Figure 9) have been known to show antimalarial activity by inhibiting Plasmodium falciparum dihydrofolate reductase, owing to the thiosemicarbazide moiety (hydrogen bond donor and accepter) and aromatic ring (hydrophobic moiety).50, 51
4-5. Synthesis of 4-Quinolones and 4-Methoxyquinolines Possessing N-Functional Group at 2-Position
The synthesis of the starting materials (71, 72) is shown in Scheme 23.58 The 4-quinolone-2-carboxylate (69) was methylated with methyl iodide/potassium carbonate to provide the 4-methoxy derivative (70). Compounds 69 and 70 were converted into the 2-carbohydrazide derivatives (71) and (72), respectively. The reaction of compound 71 with nitrous acid gave the 4-quinolone-2-carbonylazide (73), whose heating would produce a 4-quinolon-2-yl isocyanate intermediate (29’) (Scheme 24).59 The reaction of an intermediate 29’ with aniline derivatives, arylaminoacetamides, or alcohols formed the 1-aryl-3-(4-quino- lon-2-yl)ureas (74a-e), 1-aryl-3-(4-quinolon-2-yl)imidazolidine-2,4-diones (75a,b), or N-(4-quinolon-2-
yl)carbamates (76a,b), respectively. Compounds 74a-e exhibited antimalarial activity in vitro to chloroquine-resistant Plasmodium falciparum (Gambia Cl D-3, ATCC-50037) (IC50, 0.93-4.00 µM) (Table 3), and these compounds would inhibit Plasmodium falciparum dihydrofolate reductase in a similar manner to that of the 1,3-diarylureas shown in Figure 9 (section 3).
The reaction of the 4-methoxyquinoline-2-carbohydrazide (72) with nitrous acid would produce the 4-methoxyquinoline-2-carbonylazide (78) and then an intermediate (30’), whose reaction with various anilines, arylaminoacetamides, or alcohols gave the 1-aryl-3-(4-methoxyquinolin-2-yl)ureas (79a-d), 1-aryl-3-(4-methoxyquinolin-2-yl)imidazolidine-2,4-diones (80a,b), or N-(4-methoxyquinolin-2-yl)car- bamates (81a,b), respectively (Scheme 25). Compounds 79-81 did not represent antimalarial activity.
4-6. Synthesis of 3-Substituted 2-Methyl-4-quinolones
The 3-alkyl-2-methyl-4-quinolones (82a,b) and other derivatives were synthesized by the reaction of 4-substituted 3-methoxyanilines with 2-substituted ethyl acetoacetates (Scheme 26).60 Compounds 82a,b were most active in the series of compounds 82, showing antimalarial activity to Plasmodium falciparum W2 (EC50: 82a, 12.3 nM; 82b, 6.03 nM) and Plasmodium falciparum TM90-C2B (EC50: 82a, 2.95 nM; 82b, 1.59 nM).
Compound (83) was also converted into the 3-alkenyl-2-methyl-4-quinolones (84a,b) and 3-alkynyl-2- methyl-4-quinolone (85), as shown in Scheme 27.60 Compounds 84a,b exhibited weaker activity than those of compounds 82a,b [W2 (EC50: 84a, 39.4 nM; 84b, 31.5 nM); TM90-C2B (EC50: 84a, 14.8 nM; 84b, 10.6 nM)].
4-7. Synthesis of 2-Aryl-4-quinolone-3-carboxylates
The reaction of the 7-methoxy-4-quinolone-3-carboxylate (86) with phosphoryl chloride gave the 4-chloroquinoline-3-carboxylate (87), whose reaction with MCPBA gave the 1-oxide derivative (88) (Scheme 28).61 The reaction of compound 88 with phosphoryl bromide provided the 2-bromo-4-chloro- quinoline-3-carboxylate (89), whose cross-coupling and then hydrolysis afforded the 2-aryl-4-quinolone- 3-carboxylates (90). One of compounds 90 (Ar = Ph-m-OMe) was most promising analogue, showing antimalarial activity to Plasmodium falciparum chloroquine-resistant strain K1 (EC50: 0.13 µM) and 3D7 (EC50: 0.10 µM). In compounds 90, it was found that (1) the 3-carboxylate derivatives were superior to
the 3-carboxylic acid derivatives, (2) the 7-methoxy derivatives favored over the 5-methoxy derivatives, and (3) the substituent of the 2-aryl group was better in the 3-position than in the 2- or 4-position.
5. DIVERSE 4-QUINOLONES WITH VARIOUS BIOLOGICAL ACTIVITIES
There have been many reports on the naturally occurring 4-quinolones, some of which are shown in Fig-
ure 10. Quinolactacin A2 is the C1’ diastereomer of quinolactacin A1, and quinolactacin A2 has exhibited 14 times higher anti-acetylcholinesterase activity than its diastereomer quinolactacin A1.62,63 The 2-alkyl-4-quinolones (91),64,65 (92),65,66 and (93)67 represented the antibiotic (91), antirheumatic (92), antisparsmodic (92), and antifungal/red pepper growth promoting (93) activities. The 2-alkyl- thio-4-quinolones (94) are artificial compounds and known to exhibit antibacterial activities, especially to MRSA.68
5-1. Synthesis of 2,6-Disubstituted 4-Quinolones with Anticancer Activity
The reaction of the N-arylbenzamides (95a-k) with phosphorus pentachloride gave imidoyl chloride intermediates (31’a-k), whose reaction with sodium diethyl malonate produced dicarboxylate inter- mediates (32’a-k), respectively (Scheme 29).69 The heating of intermediates 32’a-k at 170 °C afforded the 6-substituted 2-arylquinolone-3-carboxylates (96a-k), whose reaction with 10% sodium hydroxide provided the 4-quinolone-3-carboxylic acids (97a-k), respectively. Compounds (98a-k) were also formed together with compounds 96a-k, presumably due to the hydrolysis and decarboxylation during the cyclization of intermediates 32’a-k. One of compounds 97a-k (R1 = OMe, R2 = F) had the highest in vitro cytotoxic activity to A549 lung cancer cells (ED50, 0.19 µg/mL).
5-2. Synthesis of 5,8-Disubstituted 4-Quinolones with Antitumor Activity
The reaction of the substituted anilines (99) with Meldrum’s acid and trimethyl orthoformate gave the 5-arylaminomethylene-2,2-dimethyl-1,3-dioxane-4,6-diones (100), whose heating in diphenyl ether provided the 4-quinolones (101) (Scheme 30).70 One of compounds 101 (5-hydroxy-8-methoxy-4- quinolone) exhibited the potent antitumor activity for HL60 (IC50, 1.7 µM).
5-3. Synthesis of 4-Quinolones and Related Compounds Aiming at Antibacterial and Other Biologi- cal Activities
Since the 4-quinolones with the long chain substituent at the 2-position such as compounds 91-94 (Figure 10) showed various biological activities, the 6-fluoro-4-quinolones (102, 103) and 6-fluoroquinolines (104, 105) having the long chain at the 2-position were synthesized by the reaction of compounds 71 and 72 with methyl isothiocyanate or 1,1,1-trifluoropentane-2,4-dione, respectively (Scheme 31).58 Compounds 103 and 105 represented weak anti-MRSA activity to five kinds of strains (ATCC 33591, 33592, 33593, 13301, 11632) at concentrations of 16-32 ppm, and compound 105 showed weak antifungal activity to five kinds of fungi (Candida albicans, Candida krusei, Aspergillus fumigatus, Tricophyton rubrum, Tricophyton mentagrophytes) at concentrations of 8-16 ppm.58
The reaction of the 2-arylquinolines (106a-d)71 with methyl trifluoromethanesulfonate followed by oxidation with potassium ferricyanide gave the 2-arylquinolone alkaloids (107a-d), respectively (Scheme 32),72 which were synthesized to investigate the antibacterial and other pharmacological activities.
The reaction of 4-chloro-N-(2-hydroxyphenyl)anthranilic acid (108) with various acyl chlorides provided
the N-acylated compounds (109), whose treatment with hot acetic anhydride furnished the 6-substituted 2-chlorobenzoxazolo[3,2-a]quinolin-5-ones (110), respectively (Scheme 33). These compounds 110 were synthesized to search for potential quinolone antibacterial agents.73
The reaction of the substituted pyridines (111a-k)74-76 with various bases produced the 1,6-naphth- yridin-4-ones (112a-k), respectively (Scheme 34),77 which were synthesized as candidates of antibacterial quinolone analogues.
The reaction of the quinoline-3-carboxylate 54 with pentaerythritol tribromide gave the 1,1’-(propane-1,- 3-diyl)di(4-quinolone-3-carboxylate) (113) presumably via intermediates (33’ and 34’) (Scheme 35), but the 1-(2,2-bisbromomethyl-3-hydroxypropyl)-4-quinolone-3-carboxylate (Figure 11) was not obtained.78 The bromination of compound 113 gave the 1,1’-(2-bromo-2-bromomethylpropane-1,3-diyl)di(4-quinol-
one-3-carboxylate) (114), whose reaction with sodium acetate furnished the 1,1’-(2-acetoxymethyl- propene-1,3-diyl)di(4-quinolone-3-carboxylate) (Z-115). This conversion mechanism is shown in Figure 11, wherein compound 114 would favor the anti conformation of two quinolone rings. Subsequent hydrolysis of compound Z-115 with sodium hydroxide gave the 1,1’-(2-hydroxymethyl- propene-1,3-diyl)di(4-quinolone-3-carboxylic acid) (E-116), which would be produced via an anion intermediate (35’) (Figure 11). The abstraction of the N1-methylene proton with sodium hydroxide and then the π−π stacking between two quinolone rings might promote the transformation of compound Z-115 into compound E-116. The reaction of compound 114 with imidazole provided the 1,1’-[2-(imidazol-1- ylmethyl)propene-1,3-diyl]di(4-quinolone-3-carboxylate) (E-117), whose hydrolysis with sodium hydroxide also resulted in the E/Z conversion to form the 1,1’-[2-(imidazol-1-ylmethyl)propene-1,3- diyl]di(4-quinolone-3-carboxylic acid) (Z-118). The geometry for (E-116, E-117) and (Z-115, Z-118) was supported by the NOE spectral data. Biological activity is not found in the above 4-quinolone dimers yet.
The reductive ring opening of the 5-(isoxazol-5-yl)-2-(pyrazin-2-yl)pyrimidine (119) with catalytic amount of Mo(CO)6 gave the 2-(pyrazin-2-yl)pyrimidine (120), whose reaction with potassium t-butoxide resulted in the cyclization to produce the 1,3,8-triazanaphthalen-5(8H)-one (121) (Scheme 36).79
6. TAUTOMERISM OF QUINOLONES: TAUTOMERIC STRUCTURES BETWEEN 4-OXO AND 4-HYDROXY FORMS IN SOLUTION
There have been some literatures on the tautomeric structures of the 4-quinolones in solution and solid state, as described below. The tautomerism of the 4-quinolones between the 4-oxo and 4-hydroxy forms depends on the kind of solvents, substituents in the quinoline ring, and/or temperature of the solution, when the N1 of the 4-quinolones is not blocked with the alkyl or other substituent. Several investigations are exhibited below concerning the tautomerism or tautomeric structures of various 4-quinolones in CDCl3, CD3OD, DMSO-d6, TFA-d1, and/or other solvents.
6-1. Quinolones with Enolcarbonyl Function and Related Compounds (in CDCl3, DMSO-d6)
The tautomeric structure of the hydroxyquinolone (122) was assigned as the 4-hydroxy-2-oxo form in CDCl3 from the comparison of the 13C-NMR spectral data among compound 122, 4-methoxy- 1-methyl-2-quinolone (123), and 2-methoxy-1-methyl-4-quinolone (124) (Scheme 37).80,81 The C4 and C4a chemical shifts of compound 122 [δ 161.3 (C4), 116.3 (C4a)] are similar to those of 2-oxo compound
123 [δ 162.5 (C4), 116.3 (C4a)], while the C4 signal of the 1-methyl-4-quinolone 124 (δ 178.3) was observed in a lower magnetic field. The C8a chemical shifts are similar values among compounds 122, 123, and 124 (δ 139.6-140.1).
The tautomeric structures of the 3-substituted 2,4-dihydroxyquinolines (125a-d) depended on the C3-sub- stituents (Scheme 38).80 Namely, the 4-hydroxy-2-oxo form was preferred in DMSO-d6 when the C3-substituent was the methylsulfanyl or cyano group (125a,b), while the 2-hydroxy-4-oxo form was predominant in DMSO-d6 when the C3-substituent was the methylsulfinyl or methylsulfonyl group (125c,d).
6-2. 2-Substituted 4-Quinolones and Related Compounds (in CDCl3, CDCl3-CD3OD, DMSO-d6)
The tautomeric structure of compound 92 was assigned as the 4-oxo form A in CDCl3 from the comparison with the C3-H chemical shift of the 4-methoxyquinoline (126) (Figure 12).82 The 2-methoxy derivative (127) and 2-methylsulfanyl derivatives (128 and 129) were predominant as the 4-oxo form A in CDCl3-CD3OD and as the 4-hydroxy form B in DMSO-d6.83
6-3. 4-Quinolone-2-carboxylate and Related Compounds (in DMSO-d6)
The 4-quinolone-2-carboxylate 69 and 4-quinolone-2-carbohydrazide 71 were found to exist as the 4-oxo form A in DMSO-d6 (Scheme 39).58
The C4 chemical shifts were similar values between the 4-quinolones 69 and 71 (δ 175.7-178.5 in DMSO-d6) and the 1-methyl-4-quinolone 124 (Scheme 37) (δ 178.3 in CDCl3),80,81 other 4-quinolones 93 (Figure 10),67 112 (R = Ph) (Scheme 34)77 (δ 176.6-177.3 in CDCl3). The C4 signals of the 4-methoxyquinolines 70 and 72 (δ 161.8-162.6 in DMSO-d6) appeared in higher magnetic fields than those of the 4-quinolones.
On the other hand, the C3-H chemical shifts were close values between the 4-quinolones 69 and 71 (δ 6.59-6.72 in DMSO-d6) and the 1-methyl-4-quinolones 107 (Scheme 32) (δ 6.29-6.38 in CDCl3),71 other 4-quinolones 101 (Scheme 30) (δ 5.80-6.25 in CDCl3 or DMSO-d6),70 98 (Scheme 29),69 112 (Scheme 34)77 (δ 6.10-6.47 in CDCl3 or DMSO-d6). These C3-H signals of the 4-quinolones (δ 5.80-6.72) were observed in higher magnetic fields than those of the 4-methoxyquinolines 70 and 72 (δ 7.51-7.54 in DMSO-d6), whose values were similar to those of the quinolines 106 (Scheme 32)71 (δ 7.54-7.85 in CDCl3), 5-substituted 6,8-bistrifluoroacetylquinolines (130) (δ 7.23-7.72 in CDCl3 or CD3CN),84 and 8-substituted 5,7-bistrifluoroacetylquinolines (131) (δ 7.48-7.79 in CDCl3)85 (Figure 13).
6-4. 4-Quinolone-2-carboxylate and Related Compounds (in TFA-d1 at Room Temperature)
Compounds 69 and 71 were found to present as the 4-hydroxy form (B-D)+ in TFA-d1, which was supported by the carbon and proton NMR spectral data (Scheme 40).58
The C4, C4a, C8a, and C3-H chemical shifts were similar values between compounds 69, 71 [4-hydroxy form (B-D)+] and the 4-methoxyquinolines [(70, 72)-D]+ in TFA-d1. Furthermore, the deuteronation site was found to be the quinoline N1 by the comparison of the C2, C4, C7, and C8a chemical shifts in DMSO-d6 with those in TFA-d1 (Table 4). Namely, the deuteronation of the quinoline N1 resulted in the
shielding of the C2, C8, and C8a and the deshielding of the C4 and C7, wherein the differences were 6.6 or more in δ values. Similar shielding and deshielding were confirmed in pyridinium salt,86 4H-1,3,4- thiadiazino[5,6-b]quinoxalines (Figure 8),40 and pyridazino[3,4-b]quinoxalin-4(1H)-ones41 (Figure 8).
6-5. 4-Quinolone-2-carbohydrazide Derivatives (in DMSO-d6)
The 4-quinolone-2-carbohydrazide derivatives 102 (Scheme 31) and (132) showed the tautomeric equilibria between the 4-oxo A and 4-hydroxy B forms in DMSO-d6 (Scheme 41).58
Two kinds of the C3-H signals were observed in compounds 102 and 132 (4-oxo form A: δ 6.69-6.76; 4-hydroxy form B: δ 7.48-7.49), wherein the 4-oxo tautomer A was predominant (68-81%).
6-6. 4-Quinolones and Related Compounds with Nitrogen Function at 2-Position (in DMSO-d6)
The 1-aryl-3-(quinolin-2-yl)ureas 74 and 1-aryl-3-(quinolin-2-yl)imidazolidine-2,4-diones 75 (Scheme 24) were found to exist as the 4-hydroxyquinoline form B in DMSO-d6 (Scheme 42).59 Namely, the C4
and C3-H chemical shifts were similar values between compounds 74,75 [δ 161.8-163.2 (C4), 6.70-6.99 (C3-H)] and the corresponding 4-methoxyquinolines 79,80 [δ 162.6-163.2 (C4), 6.91-7.22 (C3-H)]. However, the N-(quinolin-2-yl)carbamates 76a,b (Scheme 24) showed the tautomeric equilibria between the 4-quinolone A and 4-hydroxyquinoline B forms in DMSO-d6 (Scheme 43).59 Two kinds of the C4 and C3-H signals were confirmed and assigned to the 4-quinolone tautomer A [δ 175.7-176.9 (C4), 5.86-6.68 (C3-H)] and the 4-hydroxyquinoline tautomer B [δ 161.8-162.1 (C4), 7.60-7.66 (C3-H)]. The C4 and C3-H chemical shifts for the 4-hydroxy form B of compounds 76a,b were similar to those of the corresponding 4-methoxyquinolines 81a,b (Scheme 25) [δ 162.3 (C4), 7.65-7.67 (C3-H)] (Scheme 43).
6-7. 4-Quinolones and Related Compounds with Nitrogen Function at 2-Position [in TFA-d1- DMSO-d6 (3:1) at Room Temperature]
The 1-aryl-3-(quinolin-2-yl)ureas 74, 1-aryl-3-(quinolin-2-yl)imidazolidine-2,4-diones 75, and N-(qui- nolin-2-yl)carbamate 76b (Scheme 24) were found to present as the 4-hydroxyquinoline form (B-D)+ in TFA-d1–DMSO-d6 (3:1) (Scheme 44).59,87 That is, the C3-H chemical shifts were similar values between compounds [(74, 75, 76)-(B-D)]+ and the corresponding 4-methoxyquinolines [(79, 80, 81)-D]+ (Table 5). The C4 chemical shifts were also close values between compounds [(74, 75, 76)-(B-D)]+ (δ 171.6-174.9)
and the corresponding 4-methoxyquinolines [(79, 80, 81)-D]+ (δ 172.3-174.4), wherein the C4 chemical shifts underwent the deshielding (about 10-11 in δ values) because of the deuteronation to the quinoline N1, as described in the section 6-4 (Table 4).
On the contrary, the ethyl N-(quinolin-2-yl)carbamate 76a (Scheme 24) exhibited the tautomeric equilibria between the 4-oxo (A-D)+ and 4-hydroxy (B-D)+ forms in TFA-d1–DMSO-d6 (3:1) (Scheme 45).87 Two kinds of the C3-H signals of compound 76a were confirmed and assigned to the 4-quinolone tautomer (A-D)+ (δ 7.52) and the 4-hydroxyquinoline tautomer (B-D)+ (δ 6.55). The chemical shift of the (B-D)+ (δ 6.55) is similar to the values of compounds 76b and 81 in Table 5 (δ 6.32-6.64).
6-8. D-H Exchange for C3-H of 4-Quinolones with Nitrogen Function at 2-Position [in TFA-d1- DMSO-d6 (3:1) at 60 °C]
The D-H exchange of the C3-H was observed for compounds 74-76 with the C2-nitrogen function in TFA-d1–DMSO-d6 (3:1) at 60 °C (Scheme 46), whereas this D-H exchange did not take place in the 4-quinolone-2-carbonyl compounds 69,71 and 4-methoxyquinolines 70,72,79-81 (Scheme 47).87 More-
over, the D-H exchange was clarified to occur via the 4-hydroxy tautomer (B-D)+, but not via the 4-oxo tautomer (A-D)+, from the data on the tautomerism of the ethyl N-(quinolin-2-yl)carbamate 76a (Scheme 46). Namely, the integral intensity for the C3-H signal of the 4-hydroxy tautomer (B-D)+ (76a: δ 6.55) diminished with the laps of time, while the integral intensity for the C3-H signal of the 4-oxo tautomer (A-D)+ (76a: δ 7.52) remained unchanged. The D-H exchange rate of the C3-H was faster in the stronger electron-donating 2-substituent than in the weaker electron-donating 2-substituent, that is, in the order of urea > carbamate > imidazolidinedione. The electron-donating nature of the C2-substituent to the C3 would augment the electron density of the C3, which seemed to be an important factor for the D-H exchange of the C3-H.
6-9. Postulated D-H Exchange Mechanism for C3-H of 4-Quinolones with Nitrogen Function at 2-Position [in TFA-d1-DMSO-d6 (3:1) at 60 °C]
The postulated D-H exchange mechanism of the C3-H in the quinoline ring is shown in Scheme 48, wherein the crucial step would be the hydrogen bonding between the C4-OD group of the quinoline ring and the carbonyl group of deuteriotrifluoroacetic acid.87 Such a convenient mechanism for the cyclic D-H exchange of the C3-H lacks in the 4-methoxyquinolines [(79-81)-D]+ and the 4-oxo tautomer (A-D)+ (Figure 14).
7. POSTSCRIPT
As described above, the synthesis and biological activities of numerous 4-quinolones and related compounds have been reported up to date in the journal, patent, and monograph literatures. Some of these literatures are introduced in this review, whose volume is however inadequate for the quinolone researchers engaged in the development of a new synthetic methodology and/or clinically available medicaments. The computer research on the synthesis and biological activities of 4-quinolones manifests the presence of more than hundred review articles in 2000-2013, and the utilization of the suitable articles is recommended to the quinolone investigators for the aid of various studies. For instance, the following publications have appeared as the review articles except for the category of antibacterial new quinolones: skeletal construction, modification, and biological activities of 4-quinolones,88 synthesis and biological activities of 4-quinolone-metal complexes,89,90 synthesis, transformation, structural properties, and biological activities of 2-aryl-4-quinolones.91 At last, this review describes the tautomeric equilibria (tautomeric structures) between the 4-oxo and 4-hydroxy forms in solution together with their proton and carbon NMR spectral data indicating the crucial and diagnostic chemical shifts. The authors will be delightful if these spectral data are useful and available for the future investigations of the quinolone researchers.
References
1. Y. Kurasawa, A. Takada, and H. S. Kim, J. Heterocycl. Chem., 1995, 32, 1085. CrossRef
2. H. S. Kim, Y. Kurasawa, and A. Takada, J. Heterocycl. Chem., 1989, 26, 871. CrossRef
3. H. S. Kim, Y. Kurasawa, C. Yoshii, M. Masuyama, A. Takada, and Y. Okamoto, J. Heterocycl. Chem., 1990, 27, 1119. CrossRef
4. H. S. Kim, S. W. Nam, and Y. Kurasawa, J. Korean Chem. Soc., 1990, 34, 469.
5. H. S. Kim, Y. Kurasawa, C. Yoshii, M. Masuyama, A. Takada, and Y. Okamoto, J. Heterocycl. Chem., 1990, 27, 1115. CrossRef
6. Y. Kurasawa, R. Katoh, F. Mori, M. Fukuchi, M. Okamoto, A. Takada, H. S. Kim, and Y. Okamoto, J. Heterocycl. Chem., 1992, 29, 1009. CrossRef
7. H. S. Kim, Y. Kurasawa, and A. Takada, J. Heterocycl. Chem., 1989, 26, 1511. CrossRef
8. H. S. Kim, Y. Kurasawa, C. Yoshii, M. Masuyama, A. Takada, and Y. Okamoto, J. Heterocycl. Chem., 1990, 27, 1111. CrossRef
9. Y. Kurasawa, A. Takada, H. S. Kim, and Y. Okamoto, J. Heterocycl. Chem., 1993, 30, 1659. CrossRef
10. H. S. Kim, Y. Kurasawa, C. Yoshii, M. Masuyama, A. Takada, and Y. Okamoto, J. Heterocycl. Chem., 1990, 27, 819. CrossRef
11. H. S. Kim, Y. Kurasawa, C. Yoshii, M. Masuyama, A. Takada, and Y. Okamoto, J. Heterocycl. Chem., 1990, 27, 2197. CrossRef
12. Y. Kurasawa, T. Kureyama, N. Yoshishiba, R. Katoh, A. Takada, H. S. Kim, and Y. Okamoto, J. Heterocycl. Chem., 1993, 30, 537. CrossRef
13. Y. Kurasawa, N. Yoshishiba, T. Kureyama, T. Okano, A. Takada, H. S. Kim, and Y. Okamoto, J. Heterocycl. Chem., 1992, 29, 1653. CrossRef
14. Y. Kurasawa, M. Sekine, and H. S. Kim, J. Heterocycl. Chem., 1996, 33, 1859. CrossRef
15. H. S. Kim, T. E. Kim, S. T. Kwag, Y. T. Park, Y. S. Hong, Y. Okamoto, and Y. Kurasawa, J. Heterocycl. Chem., 1997, 34, 1539. CrossRef
16. H. S. Kim, E. A. Kim, G. Jeong, Y. T. Park, Y. S. Hong, Y. Okamoto, and Y. Kurasawa, J. Heterocycl. Chem., 1998, 35, 445. CrossRef
17. H. S. Kim, Y. Okamoto, and Y. Kurasawa, J. Heterocycl. Chem., 1997, 34, 1029. CrossRef
18. H. S. Kim, T. E. Kim, S. U. Lee, D. I. Kim, S. W. Han, Y. Okamoto, T. Mitomi, and Y. Kurasawa, J. Heterocycl. Chem., 1998, 35, 1515. CrossRef
19. Y. Kurasawa, T. Mitomi, Y. Okamoto, and H. S. Kim, J. Heterocycl. Chem., 1998, 35, 1333. CrossRef
20. Y. Kurasawa, A. Tsuruoka, N. Rikiishi, N. Fujiwara, Y. Okamoto, and H. S. Kim, J. Heterocycl. Chem., 2000, 37, 791. CrossRef
21. R. Albrecht, Prog. Drug Res., 1977, 21, 46.
22. W. A. White, DOS 2005104; (Chem. Abstr., 1970, 73, 77269).
23. W. A. White, DOS 2065719; (Chem. Abstr., 1975, 83, 58860).
24. M. Pesson, P. D. Lajudie, M. Antoine, S. Chabassier, P. Girard, and C. R. Hebd, Seances Acad. Sci., Ser. C, 1976, 282, 861; (Chem. Abstr., 1976, 85, 63035r).
25. R. Albrecht, Prog. Drug Res., 1977, 21, 62.
26. G. Y. Lesher, E. J. Froelich, M. D. Gruett, J. B. Bailey, and R. P. Brundage, J. Med. Chem., 1962, 5, 1063. CrossRef
27. M. P. Wentland, R. B. Perni, P. H. Dorff, R. P. Brundage, M. J. Castaldi, T. R. Bailey, P. M. Carabateas, E. R. Bacon, D. C. Young, M. G. Woods, D. Rosi, M. L. Drozd, R. K. Kullnig, and F. J. Dutko, J. Med. Chem., 1993, 36, 1580. CrossRef
28. Y. Kurasawa, A. Takano, K. Harada, A. Takada, H. S. Kim, and Y. Okamoto, Khim. Geterotsikl. Soed., 1995, 9, 1245.
29. J. Elguero, C. Maezin, A. R. Katritzky, and P. Linda, Advances in Heterocyclic Chemistry, Sup. 1, The Tautomerism of Heterocycles, ed by A. R. Katritzky and A. J. Boulton, Academic Press, New York, San Francisco, London, 1976, P. 78, and references cited therein.
30. L. Besford, G. Allen, and J. M. Bruce, J. Chem. Soc., 1963, 2867. CrossRef
31. Y. Kurasawa and H. S. Kim, J. Heterocycl. Chem., 2002, 39, 551. CrossRef
32. Y. Kurasawa, S. Ohshima, Y. Kishimoto, M. Ogura, Y. Okamoto, and H. S. Kim, Heterocycles, 2001, 54, 359. CrossRef
33. Y. Kurasawa and H. S. Kim, J. Heterocycl. Chem., 2005, 42, 387. CrossRef
34. Y. Kurasawa, J. Takizawa, Y. Maesaki, A. Kawase, Y. Okamoto, and H. S. Kim, Heterocycles, 2002, 58, 359. CrossRef
35. Y. Kurasawa, W. Satoh, I. Matsuzaki, Y. Maesaki, Y. Okamoto, and H. S. Kim, J. Heterocycl. Chem., 2003, 40, 837. CrossRef
36. Y. Kurasawa, I. Matsuzaki, W. Satoh, Y. Okamoto, and H. S. Kim, Heterocycles, 2002, 56, 291. CrossRef
37. Y. Kurasawa, A. Kawase, J. Takizawa, Y. Maesaki, E. Kaji, Y. Okamoto, and H. S. Kim, J. Heterocycl. Chem., 2005, 42, 551. CrossRef
38. Y. Kurasawa, E. Kaji, Y. Okamoto, and H. S. Kim, J. Heterocycl. Chem., 2005, 42, 249. CrossRef
39. Y. Kurasawa, M. Nakamura, H. Ashida, M. Masuda, E. Kaji, Y. Okamoto, and H. S. Kim, J. Heterocycl. Chem., 20007, 44, 1231.
40. H. S. Kim, Y. Okamoto, and Y. Kurasawa, J. Heterocycl. Chem., 1997, 34, 1029. CrossRef
41. Y. Kurasawa, T. Mitomi, Y. Okamoto, and H. S. Kim, J. Heterocycl. Chem., 1998, 35, 1333. CrossRef
42. K. Kaur, M. Jain, R.-P. Reddy, and R. Jain, Eur. J. Med. Chem., 2010, 45, 3245, and references cited therein. CrossRef
43. K. Fujimoto, D. Morisaki, M. Yoshida, T. Namba, H.-S. Kim, Y. Wataya, H. Kourai, H. Kakuta, and K. Sasaki, Bioorg. Med. Chem. Lett., 2006, 16, 2758. CrossRef
44. K. Motoshima, Y. Hiwasa, M. Yoshikawa, K. Fujimoto, A. Tai, H. Kakuta, and K. Sasaki, Chem. Med. Chem., 2007, 2, 1527. CrossRef
45. M. Yoshikawa, K. Motoshima, K. Fujimoto, A. Tai, H. Kakuta, and K. Sasaki, Bioorg. Med. Chem., 2008, 16, 6027. CrossRef
46. Y. Kitade, H. Kojima, F. Zulfiqar, H.-S. Kim, and Y. Wataya, Bioorg. Med. Chem. Lett., 2003, 13, 3963. CrossRef
47. N. Tanaka, M. Nakanishi, Y. Kusakabe, K. Shiraiwa, S. Yabe, Y. Ito, Y. Kitade, and K.-T. Nakamura, J. Mol. Biol., 2004, 343, 1007. CrossRef
48. T. Ando, M. Iwata, F. Zulfiqar, T. Miyamoto, M. Nakanishi, and Y. Kitade, Bioorg. Med. Chem., 2008, 16, 3809. CrossRef
49. T. Ando, K. Kojima, P. Chahota, A. Kozaki, N.-D. Milind, and Y. Kitade, Bioorg. Med. Chem. Lett., 2008, 18, 2615. CrossRef
50. G. Rastelli, S. Pacchioni, W. Sirawaraporn, R. Sirawaraporn, M.-D. Parenti, and A.-M. Ferrari, J. Med. Chem., 2003, 46, 2834. CrossRef
51. S. Madapa, Z. Tusi, D. Sridhar, A. Kumar, M.-I. Siddiqi, K. Srivastava, A. Rizvi, R. Tripathi, S.-K. Puri, G.-B.-S. Keshava, P.-K. Shukla, and S. Batra, Bioorg. Med. Chem., 2009, 17, 203.
52. J. Leban, S. Pegoraro, M. Dormeyer, M. Lanzer, A. Aschenbrenner, and B. Kramer, Bioorg. Med. Chem. Lett., 2004, 14, 1979. CrossRef
53. G. Anquetin, M. Rouquayrol, N. Mahmoudi, M. Santillana-Hayat, R. Gozalbes, J. Greiner, K. Farhati, F. Derouin, R. Guedj, and P. Vierling, Bioorg. Med. Chem. Lett., 2004, 14, 2773. CrossRef
54. G. Anquetin, J. Greiner, N. Mahmoudi, M. Santillana-Hayat, R. Gozalbes, K. Farhati, F. Derouin, A. Aubry, E. Cambau, and P. Vierling, Eur. J. Med. Chem., 2006, 41, 1478. CrossRef
55. Y. Kurasawa, K. Yoshida, N. Yamazaki, E. Kaji, K. Sasaki, Y. Hiwasa, A. Tsukamoto, and H. Ito, J. Heterocycl. Chem., 2010, 47, 657.
56. Y. Kurasawa, K. Yoshida, N. Yamazaki, E. Kaji, K. Sasaki, Y. Zamami, Y. Sakai, T. Fujii, and H. Ito, J. Heterocycl. Chem., 2012, 49, 288. CrossRef
57. Y. Kurasawa, K. Yoshida, N. Yamazaki, K. Sasaki, Y. Zamami, Z. Min, A. Togi, H. Ito, E. Kaji, and H. Fukaya, J. Heterocycl. Chem., 2014, 51, E249. CrossRef
58. Y. Kurasawa, K. Yoshida, N. Yamazaki, K. Sasaki, and Y. Zamami, J. Heterocycl. Chem., 2012, 49, 1323. CrossRef
59. Y. Kurasawa, K. Yoshida, N. Yamazaki, K. Sasaki, Y. Zamami, Z. Min, A. Togi, H. Ito, E. Kaji, and H. Fukaya, J. Heterocycl. Chem., 2014, 51, E241. CrossRef
60. R. M. Cross, A. Monastyrskyi, J. Burrows, D. E. Kyle, and R. Manetsch, J. Med. Chem., 2010, 53, 7076. CrossRef
61. Y. Zhang, W. A. Guiguemde, M. Sigal, F. Zhu, M. C. Connelly, S. Nwaka, and R. K. Guy, Bioorg. Med. Chem., 2010, 18, 2756. CrossRef
62. W.-G. Kim, N.-K. Song, and I.-D. Yoo, J. Antibiot., 2001, 54, 831. CrossRef
63. X. Zhang, W. Jiang, and Z. Sui, J. Org. Chem., 2003, 68, 4523. CrossRef
64. S. J. Wratten, M. S. Wolfe, R. J. Andersen, and D. J. Faulkner, Antimicrob. Agents Chemother.,1977, 11, 411. CrossRef
65. T. G. Back, M. Parvez, and J. E. Wulff, J. Org. Chem., 2003, 68, 2223. CrossRef
66. T. G. Back and J. E. Wulff, J. Chem. Soc., Chem. Commun., 2002, 1710. CrossRef
67. S.-S. Moon, P. M. Kang, K. S. Park, and C. H. Kim, Phytochemistry, 1996, 42, 365. CrossRef
68. K. Narita, Y. Izumi, H. Nishino, T. Yoshida, Y. Takahashi, O. Nagata, and H. Katoh, 121st Conference of the Pharmaceutical Society of Japan, Sapporo, Japan, Abstract-3 No. 29 [PB] I-042 (2001).
69. Y.-Y. Lai, L.-J. Huang, K.-H. Lee, Z. Xiao, K. F. Bastow, T. Yamori, and S.-C. Kuo, Bioorg. Med. Chem., 2005, 13, 265. CrossRef
70. M. W. Chun, K. K. Olmstead, Y. Choi, C. O. Lee, C.-K. Lee, J. H. Kim, and J. Lee, Arc. Pharm. Res., 1998, 21, 445. CrossRef
71. J. Koyama, I. Toyokuni, and K. Tagahara, Chem. Pharm. Bull., 1998, 46, 332. CrossRef
72. J. Koyama, I. Toyokuni, and K. Tagahara, Chem. Pharm. Bull., 1999, 47, 1038. CrossRef
73. S. J. Chung, K. C. Joo, and D. H. Kim, J. Heterocycl. Chem., 1997, 34, 485. CrossRef
74. T. Konakahara and Y. Kurosaki, J. Chem. Res. Synop., 1989, 130.
75. T. Konakahara and K. Sato, Bull. Chem. Soc. Jpn., 1983, 56, 12141. CrossRef
76. T. Konakahara, M. Satoh, T. Haruyama, and K. Sato, Nippon Kagaku Kaishi, 1990, 466. CrossRef
77. S. Suzuki, N. Sakai, R. Iwahara, T. Fujiwaka, M. Satoh, A. Kakehi, and T. Konakahara, J. Org. Chem., 2007, 72, 5878. CrossRef
78. Y. Kurasawa, K. Yoshida, N. Yamazaki, E. Kaji, K. Sasaki, Y. Zamami, T. Fujii, Z. Min, H. Ito, and H. Fukaya, J. Heterocycl. Chem., 2014, 51, 1720. CrossRef
79. N. Sakai, Y. Aoki, T. Sasada, and T. Konakahara, Org. Lett, 2005, 7, 4705. CrossRef
80. J. L. G. Ruano, C. Pedregal, and J. H. Rodriguez, Heterocycles, 1991, 32, 2151. CrossRef
81. G. M. Coppola, A. D. Kahle, and M. J. Shapiro, Org. Magnet. Reson., 1981, 17, 242. CrossRef
82. T. G. Back, M. Parvez, and J. E. Wulff, J. Org. Chem., 2003, 68, 2223. CrossRef
83. C. Wentrup, V. V. R. Rao, W. Frank, B. E. Fulloon, D. W. J. Moloney, and T. Mosandl, J. Org. Chem., 1999, 64, 3608. CrossRef
84. D. Shibata, M. Medebielle, M. Hatakenaka, and E. Okada, Heterocycles, 2012, 84, 1277. CrossRef
85. E. Okada, N. Tsukushi, and N. Shimomura, Synthesis, 2000, 237. CrossRef
86. G. C. Levy and G. L. Nelson, ‘Carbon-13 Nuclear Magnetic Resonance Spectroscopy for Organic Chemists,’ Wiley Interscience, A Division of John Wiley and Sons, Inc., New York, London, Sydney, and Toronto, 1972, P.140, and references cited therein.
87. Y. Kurasawa, K. Yoshida, N. Yamazaki, K. Sasaki, Y. Zamami, Z. Min, A. Togi, H. Ito, E. Kaji, and H. Fukaya, J. Heterocycl. Chem., 2014, 51, 1821. CrossRef
88. A. A. Boteva and O. P. Krasnykh, Chem. Heterocycl. Compd., 2009, 45, 757. CrossRef
89. R. Singh, A. Debnath, D. T. Masram, and D. Rathore, Res. J. Chem. Sci., 2013, 3, 83.
90. A. Rusu, A. Gyeresi, and G. Hancu, Acta Med. Marisiensis, 2011, 57, 149.
91. J. M. Mphahlele, J. Heterocycl. Chem., 2010, 47, 1.