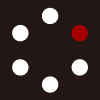
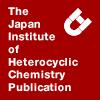
HETEROCYCLES
An International Journal for Reviews and Communications in Heterocyclic ChemistryWeb Edition ISSN: 1881-0942
Published online by The Japan Institute of Heterocyclic Chemistry
e-Journal
Full Text HTML
Received, 14th December, 2012, Accepted, 15th February, 2013, Published online, 26th February, 2013.
DOI: 10.3987/REV-12-761
■ One-Pot Asymmetric 6π-Azaelectrocyclization as a New Strategy for Alkaloid Synthesis
Toyoharu Kobayashi, Taku Sakaguchi, and Shigeo Katsumura*
Department of Chemistry, School of Science and Technology, Kwansei Gakuin University, 2-1 Gakuen, Sanda, Hyogo 669-1337, Japan
Abstract
The one-pot asymmetric 6π-azaelectrocyclization of alkenyl vinyl stannane, ethyl (Z)-2-iodo-4-oxobutenoate, and (–)-7-isopropyl-cis-aminoindanol in the presence of a Pd(0) catalyst stereoselectively produced the tetracyclic aminal compounds, resulting from the four-bond formation by controlling the stereochemistry at the two created asymmetric centers. This asymmetric one-pot protocol was developed based on the studies of the previously established asymmetric azaelectrocyclization, and the produced cyclic aminals can be regarded as synthetic precursors of 2,4-disubstituted chiral piperidines. Furthermore, we also developed a new version of the one-pot asymmetric 6π-azaelectrocyclization using t-butyl (Z)-3-formyl-2-iodopentenoate instead of ethyl (Z)-2-iodo-4-oxobutenoate, which directly afforded the precursors of the 2,4,5-trisubstituted piperidines. The syntheses of the 2,4- and 2,4,5-substituted piperidines were realized by the chemoselective reduction of the conjugated double bond to the ester group in the one-pot azaelectrocyclization products. The synthesis of the 2,4,6-trisubstituted piperidines was achieved by the stereocontrolled alkylation of the aminal moiety in the resulting cyclized products as a key step. The 2,3,4-trisubstituted piperidines were also synthesized utilizing the stereoselective 1,4-addition reaction of the unsaturated ester with a Grignard reagent resulting from the novel neighboring group participation. By applying this protocol, the total syntheses of (–)-dendroprimine, an indolizidine alkaloid, containing the 2,4,6-trisubstituted piperidine motif and (–)-20-epiuleine, a strychnos-type indole alkaloid, containing the 2,3,4-trisubstituted piperidine motif, were achieved.CONTENTS
1. Introduction
2. One-pot Asymmetric 6π-Azaelectrocyclization
2-1. Development of Asymmetric One-pot 6π-Azaelectrocyclization
2-2. Synthesis of Chiral C-2 Aryl 2,4-Disubstituted Tetrahydropyridines
2-3. Analysis of High Stereoselectivity Caused by One-pot Procedure
3. One-pot Asymmetric 6π-Azaelectrocyclization to Produce Chiral 2,4,5-Trisubstituted Piperidines
3-1. Development of One-pot Asymmetric 6π-Azaelectrocyclization to Produce Chiral
2,4,5-Trisubstituted Piperidines
3-2. Synthesis of Chiral 2,4,5-Trisubstituted Tetrahydropyridines
3-3. Improved Synthesis of Chiral 7-Isopropyl-cis-aminoindanol
4. Synthesis of Chiral Polysubstituted Piperidines
4-1. Synthesis of 2,4-Disubstituted and 2,4,5-Trisubstituted Piperidines
4-2. Stereocontrolled Alkylation of the Aminal Moiety to Produce 2,4,6-Trisubstituted
Piperidines
4-3. Novel 1,4-Addition Reaction of the Unsaturated Ester Moiety to Produce 2,3,4-Trisubstituted
Piperidines
5. Synthesis of Naturally-Occurring Alkaloids Possessing a Substituted Piperidine Core
5-1. Synthesis of Indolizidine Alkaloid, (–)-Dendroprimine, with 2,4,6-Trisubstituted Piperidine
Core
5-2. Synthesis of Strychnos-type Indole Alkaloid, (–)-20-Epiuleine, with 2,3,4-Trisubstituted
Piperidine Core
6. Conclusion
1. INTRODUCTION
The establishment of a new synthetic strategy for naturally-occurring products utilizing an efficient reaction is one of the significant tasks for organic chemists. For example, the Diels-Alder reaction has been frequently utilized to construct the cyclohexane core in complex natural compounds. In the last decade, we have focused on the concerted 6π-azaelectrocyclization reaction as a new synthetic strategy for naturally-occurring products possessing the pyridine or piperidine core.1 The 6π-azaelectrocyclization of 1-azatrienes into 1,2-dihydropyridines, which could be regarded as the useful precursor for polysubsituted pyridines and piperidines, is one of the well-known concerted pericyclic reactions.2-5 Although a number of examples of the 6π-azaelectrocyclization has been reported,6,7 the process required a high temperature and a long reaction time, and therefore, the study of the azacyclization reactivity was very limited in the literature as well as applicability of this cyclization to natural product synthesis. The pioneering work by Okamura and co-workers revealed that the s-cis-conformation at the 4,5-position of the azatrienes was very important for the smooth azaelectrocyclization (Scheme 1).5,8
In our studies regarding the enzyme inhibitory mechanism of unsaturated aldehydes,9 we found that the presence of both the C-4 ester functionality and C-6 alkenyl groups in the 1-azatrienes remarkably accelerated the azaelectrocyclization.10 Thus, the azaelectrocyclization of the azatriene prepared from (E)-3-methoxycarbonyl-2,4,6-trienal A and n-propylamine proceeded within 5 minutes at room temperature to produce the corresponding 1,2-dihydropyridine in quantitative yield (Scheme 2, a). This attractive and smooth reaction was applied to the one-pot pyridine synthesis, and the formal biomimetic synthesis of pyridinium bisretinoid, A2-E, was achieved.10-12 Moreover, we also realized the highly stereoselective asymmetric azaelectrocyclization resulting from controlling the torque selectivity of the disrotatory cyclization during the thermal 6π-electrocyclization, which was affected by the chiral nitrogen source under mild conditions.1a-c This asymmetric azaelectrocyclization provided the chiral tetracyclic 2,4-disubstituted 1,2,5,6-tetrahydropyridine, which could be regarded as the useful precursor for chiral polysubstituted piperidines, in high yield with a high diastereoselectivity (Scheme 2, b).
At the same time as our first report on the asymmetric reaction, Hsung and coworkers successfully employed the stereoselective azaelectrocyclization of the conformationally restricted 1-azatrienes under thermodynamically equilibrated conditions, and applied it to a variety of alkaloid syntheses (Scheme 3).13
In order to establish a new strategy for the alkaloid synthesis, we investigated the more simple and highly efficient ‘thermodynamically controlled’ approach to the synthesis of substituted chiral piperidines bearing a variety of substituents at the C-2 position using a one-pot process directly from vinyl stannanes, vinyl halides and chiral amino indanol in the presence of the Pd(0) catalyst.1d This new protocol would be widely applicable to the syntheses of the 2,3,4-,1h 2,4,5-,1f and 2,4,6-trisubstituted piperidine motifs.1e We now summarize our recent progress, especially focusing on the one-pot asymmetric 6π-azaelectro- cyclization and its application to alkaloid syntheses containing the polysubsituted piperidine core.
2. ONE-POT 6π-AZAELECTROCYCLIZATION
2-1. Development of Asymmetric One-pot 6π-Azaelectrocyclization
As described in the introduction (Scheme 2), we have developed the highly stereoselective asymmetric 6π-azaelectrocyclization of conformationally flexible linear 1-azatrienes using the 7-isopropyl-substituted cis-aminoindanol derivatives. However, to establish the asymmetric 6π-azaelectrocyclization as a new strategy for the alkaloid synthesis, the operation, scale-up and substrate preparation for the azaelectrocyclization of the previous stepwise method needed to be reinvestigated. The stepwise synthesis of tetracyclic 2,4-disubstituted tetrahydropyridine (–)-7, a promising precusor for substituted piperidine derivatives, was obtained in 36% yield in three steps as shown in Scheme 4. The sequence involves the following steps: the Stille coupling of vinyl stannane 2 with vinyl iodide 3, oxidation of the resulting allylic alcohol to the corresponding aldehyde using manganese dioxide, the azatriene formation by the reaction of the produced aldehyde 4 with (–)-7-isopropyl-cis-1-amino-2-indanol (–)-1, and the highly stereoselective 6π-electro-cyclization of the resulting azatriene 5 to produce the corresponding dihydropyridine 6, which was followed by the aminal formation to
give (–)-7. In order to establish this asymmetric cyclization as a practical method for alkaloid synthesis, we decided to investigate the tandem one-pot procedure of the sequence for the preparation of (–)-7 by mixing three components of (E)-vinyl stannane 2, vinyl iodide 8 and (–)-cis-1-amino-2-indanol derivative (–)-1 in the presence of a palladium catalyst. Since such a one-pot protocol significantly reduces the experimental operations including the tedious isolation procedures for each reaction, much effort has been currently devoted to the development of new tandem reactions and the conversion of the existing multi-step synthesis into the one-pot procedures. To date, a number of the powerful one-pot asymmetric reactions has been reported.14
For the first trial, (E)-vinyl stannane 2 and vinyl iodide 8 were subjected to the Stille coupling, and subsequently, cis-aminoindanol (–)-9 (commercially available) was added to the reaction mixture to affect the Schiff base formation followed by 6π-azaelectrocyclization. The reaction, however, between 2 and 8 in the presence of Pd(PPh3)4 in dimethylformamide (DMF) at 80 °C provided a complex mixture. The next trial was then the first aminal formation by the reaction of vinyl iodide 8 with aminoindanol (–)-9 at room temperature, which was followed by the Stille coupling with stannane 2 and the tandem azaelectrocyclization. To our delight, by applying this procedure, the desired tetracyclic compound (–)-10 was obtained in 28% yield with a 3:1 diastereoselectivity at C2 (Table 1, entry 1). Further examination of the solvent, additives, and Pd(0) catalyst led to the following informative observations: (i) DMF was a suitable solvent
(entries 1 and 2); (ii) LiCl as an additive increased the product yields (entries 1, 3 and 4); (iii) the Pd2(dba)3/tri(2-furyl)phosphine system was the optimal Pd(0) catalyst (entries 1, 5 and 6);15,16 and (iv) MS 4A might efficiently trap the water produced during the aminal formation, which would improve the Stille coupling reaction (entries 1, and 7). Finally, the optimized conditions mentioned in Table 1 produced (–)-10 in 82% yield with a 3:1 diastereoselectivity, namely, using the Pd2(dba)3/tri(2-furyl)phosphine catalyst in the presence of LiCl and MS 4A in DMF at 80 °C (entry 7). After establishing the optimal reaction conditions for the one-pot procedure, we then examined the stereoselectivity of the azaelectrocyclization by applying isopropyl substituted aminoindanol (–)-1, which afforded the best selectivity in the stepwise protocol,1b and the corresponding piperidine (–)-7 was obtained in 82% yield as almost a single isomer (> 40:1 by 1H NMR analysis) (entry 8). Note that this one-pot procedure successfully created four new bonds simultaneously and produced better results than the previous stepwise procedure shown in Scheme 4 (82% and > 40:1 for the one-pot protocol vs. 36% and 24:1 for the stepwise method).1b
A possible mechanism for the established one-pot procedure is shown in Scheme 5. First, the formation of the cyclic aminal 12 from the aldehyde 8 and aminoindanol (–)-1 was detected in situ by an 1H NMR analysis. This aminal formation resulted in the protection of the unstable aldehyde moiety in 8, and led to the successful Stille coupling with the vinyl stannane 2. The coupling product 13 was transformed into the 1-azatriene 5 in a reversible process, which spontaneously cyclized into the corresponding dihydropyridine 6. The tautomerization of the reactive enamine moiety in 6 proceeded to produce the corresponding iminium ion, which was immediately trapped by the neighboring hydroxyl group of the cis-aminoindanol, giving rise to the observed product, (–)-7.
2-2. Synthesis of Chiral C-2 Aryl 2,4-Disubstituted Tetrahydropyridines
The developed one-pot asymmetric azaelectrocyclization procedure was applied to the synthesis of 2,4-disubstituted piperidine derivatives having various kinds of aryl substituents at the C-2 position (Table 2). Thus, a small chiral tetrahydropyridine library bearing indolyl (entries 2 and 3), quinolyl (entry 4), pyridyl (entries 5 and 6), and thiophenyl derivatives (entry 7) along with a vinyl derivative (entry 8) was successfully prepared. Note that all the tetracyclic heterocycles could be efficiently obtained with a high diastereo-selectivity ranging from 12:1 to 20:1 with satisfactory yields (67-80%). Next, removal of the indanol moiety of the tetracyclic compound was examined. According to our previously reported method,1d aminal compounds were first reduced by lithium aluminum hydride, and the resulting diols were treated by manganese dioxide as a mild oxidant. However, in some cases, the chemical yield of the oxidation step was very low, and the procedure was sometimes not reproducible. After re-examining the reduction/oxidation conditions, we found that the diisobutylaluminum hydride (DIBAL-H) reduction followed by a lead tetraacetate treatment resulted in the clean removal of the hydroxyindane moiety.
Thus, the cyclic aminal compounds 7 and 21-27 were treated with DIBAL-H at –78 °C to provide the corresponding diols 28-35 in 69-87% yields. They were oxidized with lead tetraacetate17 in the presence of n-propylamine at –50 °C to afford the corresponding aminoalcohols 36-42 in 79-91% yields, except for one case, (–)-40 (29%, entry 5).
The plausible mechanism is described in Scheme 6. The added amine would probably not only catch the liberated acetic acid to keep the reaction media under basic conditions, but also catch the produced dialdehyde 43 resulting from the oxidative cleavage of the aminoindanol moiety by the lead tetraacetate oxidation. It supported the fact that in the absence of n-propylamine in this oxidation, the yield was much lower because of the further reaction of the generated aldehyde 43 with the desired secondary amine 36.
2-3. Analysis of High Stereoselectivity in One-pot Procedure
The one-pot procedure afforded compound (–)-7 with a higher diastereoselectivity (> 40:1) than the stepwise procedure (24:1), and other 2-aryl-substituted derivatives (–)-21-(–)-27 were also obtained with high stereoselectivities. In comparison to the stepwise procedure, which was believed to be a kinetically controlled one because the reaction was completed within 5 minutes at 24 °C in chloroform and the asymmetric cyclization using (–)-1 also proceeded at 24 °C, the one-pot procedure required heating at 80 °C in DMF, and therefore the cyclization step of this three-component one-pot procedure must occur under thermodynamically controlled conditions. The stable conformation of compound 7 was optimized at a B3LYP/6-31G(d) level using Gaussian 0318 and the calculation gave the most stable conformation of 7 as shown in Scheme 7. In this conformer, the substituent at the C-2 position on the tetrahydropyridine ring has the β-pseudo axial orientation, and no severe steric interactions exists. On the other hand, in the case of the minor isomer 44, the substituent at the C-2 position on the tetrahydropyridine ring is located in the α-pseudo equatorial orientation. In this conformer, the steric interaction between the isopropyl substitutent on the indan ring and the phenyl group at the C-2 position of the tetrahydropyridine ring apparently exists. Thus, the major isomer 7 is much more stable than 44, and hence 7 must be preferentially produced in the thermodynamically controlled one-pot procedure.
We then tried to independently isomerize both products of the cyclization, major 27 and minor 45. Both compounds were isolated by column chromatography and, in particular, 45 was collected from the reaction mixture of many cyclization experiments. On the other hand, 27 was used for the natural dendroprimine synthesis (see chapter 5-1). Compound 45 was dissolved in deuterated DMF in a NMR sample tube, and then the time-course of its isomerization at both 70 °C and 80 °C was monitored by 1H NMR. The results are shown in Scheme 8. Heated at 70 °C, a small amount of 27 was detected with a large amount of 45 by 1H NMR after 90 minutes. Heated at 80 °C, 45 gradually changed and 27 was clearly observed and the ratio of both compounds was 1:1 after 30 minutes. After 120 minutes, compound 27 became the major one with the ratio of > 8:1. On the other hand, although 27 was heated at 80 °C, 45 was not detected at all. These results obviously indicated that the reactions of 6π-azaelectrocyclization followed by enamine-iminium ion tautomerization and then aminal formation were reversible process, and most of the 45 converged to the more stable 27 at 80 °C through the inward rotation of the disrotatory 6π-azaelectrocyclization of the 1-azatirene B generated by the ring opening of 45 (Scheme 8). Thus, it was concluded that the thermodynamically more stable compound 27 was exclusively produced resulting from the reversible reactions of the 6π-azaelectrocyclization, enamine-iminium ion tautomerization and aminal formation during the one-pot procedure at 80 °C. In addition, a rough energy estimation of the stable conformations 27 and 45 by Spartan ’02 (Wave function, Inc., Irvine, CA)19 showed that the conformation of 27 was more stable than that of 45 by 7.8 kcal/mol.
3. ONE-POT ASYMMETRIC 6π-AZAELECTROCYCLIZATION TO PRODUCE CHIRAL 2,4,5-TRISUBSTITUTED PIPERIDINES
3-1. Development of One-pot Asymmetric 6π-Azaelectrocyclization to Produce Chiral 2,4,5-Trisubstituted Piperidines
In order to demonstrate the asymmetric 6π-azaelectrocyclization as a powerful strategy for the polysubstituted piperidine synthesis, we found a new version of the one-pot asymmetric procedure using the tetrasubstituted vinyl iodide 4620 (Table 3) instead of the trisubstituted one 8 (Table 2) to directly produce 2,4,5-trisubstituted tetrahydropyridines.1g The piperidine derivative having this substituent motif was not easily synthesized from the readily prepared 2,4-disubstituted aminal 7 (Scheme 7), although the introduction of an alkyl group to the C-3 and C-6 positions of 7 would be possible.
For the achievement of this new version of the one-pot azacyclization, we had to carefully consider the reaction conditions of each step in the one-pot reaction; one was the aminal formation and the other was the Stille coupling in addition to the electrocyclization step. In Table 3, although the reaction conditions mentioned in entry 1 were successful for the one-pot reaction using trisubstituted olefins 8, the complex mixture was obtained in the case of the tetrasubstituted olefin 46. After a detailed examination of the solvent and the reaction temperature (Table 3), it was found that dioxane was the most suitable solvent and 80 °C was needed for the satisfactory aminal formation to produce 47. In addition, the azaelectrocyclization of the azatriene having a tetrasubstituted olefin and a terminal phenyl group proceeded under refluxing dioxane to give 48. The optimized reaction conditions are shown in entry 6 to produce the desired product 48 in 77% yield as a single isomer.
The relative stereochemistry of the obtained compound 48 was unambiguously determined by an X-ray crystallographic analysis of the corresponding ethyl ester derivative 52 (Scheme 9).21 The X-ray crystallographic figure clearly showed that the newly created asymmetric centers at both the C-2 and C-5 positions had the β-configuration, which made the molecule thermodynamically more stable than that having the α-configuration. It was noteworthy that this one-pot procedure successfully created four new bonds completely controlling the stereochemistry at the three generated asymmetric centers resulting from converging upon the most stable compound under the thermodynamically controlled conditions.
3-2. Synthesis of Chiral 2,4,5-Trisubstituted Tetrahydropyridines
In order to investigate the generality of the new version for the one-pot asymmetric azaelectrocyclization reaction, we tried to apply the established reaction conditions (Table 3) to another system using 2-indolyl vinyl stannane 15 (Scheme 10). When the reaction was attempted under the conditions mentioned in entry 6 of Table 3, the desired 53 was unexpectedly obtained in only 30–50% yield along with the lactam compound 54 as a major byproduct in 20–30% yield. Furthermore, when the aminoindanol with no isopropyl substituent (–)-9 was used, the lactam compound 56 was exclusively obtained in 72% yield. A plausible mechanism for the lactam formation is shown in Scheme 10. The aminoindanol (–)-1 or (–)-9 first reacted with the tetrasubstituted vinyl iodide 46 to produce the imine 57. Prior to the desired Stille coupling with 15, compound 57 would then isomerize to 58 under the reversible process due to the presence of the ethyl group. Next, a very rapid intramolecular aminal formation followed by lactamization would occur to give the stable lactam iodide 59,22 which would undergo the Stille coupling with 15 to give the undesirable conjugated lactam 54 and 56. This speculation obviously indicated that the E, Z isomerization of the imine 58 would be critical for the lactam formation, and strongly suggested the possibility that the higher steric repulsion between the substituent R on the aminoindanol derivative and the ester group of the vinyl iodide moiety in 58 could suppress the E, Z isomerization resulting in the lactam formation.
To prevent the undesired isomerization, we then attempted to use the tetrasubstituted vinyl iodide 6020 possessing a bulky tert-butyl ester group. As expected, the one-pot reaction proceeded quite well and the desired tetracyclic compound 61 was obtained in 77% yield as a single diastereomer with no lactam formation (Table 4, entry 1). The obtained compound 61 was easily converted into the
2,4,5-trisubstituted 2,5-chiral tetrahydropyridine 69 by following the previously established procedure mentioned in Table 2 (Chapter 2-2). Thus, the reduction of 61 with DIBAL-H at –78 °C followed by the oxidative removal of the resulting indanol moiety with lead tetraacetate in CHCl3 in the presence of n-propylamine at –50 °C afforded the desired 69 in 59% yield for two steps. Moreover, as shown in Table 4, the established reaction conditions including the utilization of the tert-butyl ester were successfully applied to the one-pot asymmetric 6π-azaelectrocyclization with various aryl and also alkenyl vinyl stannanes in good yields with excellent stereoselectivities. The obtained tetracyclic aminals (61-68) were transformed into the corresponding tetrahydropyridine derivatives by the established procedure. Thus, a very effective and straightforward synthetic procedure for the preparation of 2,4,5-trisubstituted 2,5-chiral terahydropyridines was established.
3-3 Improved Synthesis of Chiral 7-Isopropyl-cis-Aminoindanol
For the asymmetric 6π-azaelectrocyclization, 7-isopropyl-cis-aminoindanol (–)-1 afforded a high diasteroselectivity. In addition, this chiral nitrogen source was essentially important to obtain the 2,4,5-trisubstituted tetrahydropyridine derivatives such as compounds 69-76 (Table 4) in satisfactory yields in a stereocontrolled manner as described in Chapter 3-2. The first-generation synthesis of 7-isopropyl-cis-aminoindanol is shown in Scheme 11.23 The isopropyl substituted indene was obtained by the Diels–Alder reaction of the 1-substituted diene 78 with cyclopentenone followed by aromatization, reductive removal of the phenolic hydroxyl group and dehydration. The asymmetric dihydroxylation of the resulting indene derivatives 81 followed by the Ritter reaction gave the desired (–)-7-isopropyl-cis-aminoindanol (–)-1. However, the Diels–Alder reaction and the aromatization needed vigorous reaction conditions (200 °C, 72 h and 200 °C, 48 h) and the yields of these reactions were not constant. Therefore, to apply the achieved one-pot asymmetric azaelectrocyclization reaction to the naturally-occurring alkaloid synthesis, it was necessary to improve the synthesis of (–)-7-isopropyl-cis-aminoindanol (–)-1.
For the second-generation synthesis of (–)-1, we planned to utilize the Nozarov reaction to construct the indene core.24 The synthesis of isopropyl indene 81 is shown in scheme 12. Commercially available 2-isopropylphenol (83) was reacted with trifluoromethanesulfonic anhydride to provide the corresponding triflate 84, which was followed by the carbon monoxide insertion with the aid of a palladium catalyst (Pd(OAc)2, dppf) that afforded the 2-isopropylbenzoic acid (85).25 The resulting acid 85 was converted into the Weinreb amide 86, which was transformed into the aryl vinyl ketone 87 by the reaction with vinyl magnesium bromide in 87% yield. With the Nozarov reaction precursor 87 in hand, the cyclization was attempted. In this trial, we encountered the problem of the easier polymerization of the ketone 87 rather than the cyclization to provide the indanone 88. After several trials, we found suitable reaction conditions such that the dropwise addition of a pentane solution of 87 into conc. sulfuric acid at 70 °C followed by the collection of the distilled compound produced the desired indanone 88 in 93% yield without polymerization. Reduction and then dehydration of the indanone 88 provided the indene 81 in 97% yield for 2 steps (Scheme 12).26 It was noteworthy that no purification was necessary in the last three steps.
Thus, we realized the efficient synthesis of 7-isopropyl-cis-aminoindanol (–)-1 by utilizing the Nozarov reaction as the key step. This procedure is actually convenient and helped us to establish the one-pot asymmetric azaelectrocyclization protocol as a new strategy for alkaloid synthesis.
4. SYNTHESIS OF CHIRAL POLYSUBSTITUTED PIPERIDINES
The substituted piperidines can be regarded as one of the core structures of naturally-occurring alkaloids, including the indole alkaloids (Figure 1).27 These functionalized six-member nitrogen heterocycles have drawn a great deal of attention due to their attractive pharmacological activities. The stereocontrolled synthesis of polysubstituted piperidines has therefore been a current topic in the synthetic community.28,29 When enantiomerically-pure polysubstituted piperidines are easily accessible resulting from the successful introduction of the desired alkyl substituents to the desired positions of a chiral piperidine ring, a widely applicable synthetic strategy for alkaloids consisting of a polysubstituted piperidine ring will be envisioned.28c,30
4-1. Synthesis of 2,4-Disubstituted and 2,4,5-Trisubstituted Piperidines
In order to synthesize the 2,4-disubstituted piperidines from the azaelectrocyclization product (–)-7, we examined the stereoselective reduction of the conjugated double bond in (–)-7, which can be readily prepared using the one-pot azaelectrocyclization protocol. Although (–)-7 was decomposed by using Pd/C as a hydrogenation catalyst and both the conjugated double bond and the aminal moiety were reduced by applying PtO2, fortunately, the chemoselective hydrogenation of (–)-7 with W-2 Raney-Nickel successfully produced the desired C-4 β-ester 89 as a single stereoisomer in 87% yield. Meanwhile, when (–)-7 was treated with magnesium in methanol, the C-4 α-isomer 91 was stereoselectively produced as the major product in the ratio of 5:1.31 Since both diastereoisomers 89 and 91 were selectively obtained by choosing the reducing reagent, removal of the indane moiety was next. The obtained saturated
compounds 89 and 91 were treated with DIBAL-H at –78 °C to provide the corresponding diols in 79% and 83% yields, respectively, which were treated with lead tetraacetate in the presence of n-propylamine at –50 °C as mentioned in Chapter 2-2 to afford the desired piperidine compounds 90 and 93 in 75% and 69% yields, respectively (Scheme 13) (see 2-2, Scheme 6). Next, in order to synthesize the 2,4,5-trisubstituted piperidines, the chemoselective hydrogenation of 48 with W-2 Raney-Nickel produced the desired C-4 β-ester 94 as a single stereoisomer in 87% yield. The reduction with DIBAL-H followed by the removal of the hydroxyindane moiety of the C-4 β-ester 94 with lead tetraacetate provided the (2β,4β,5β)-trisubstituted piperidine derivative 95 in 77% yield.32 Thus, the synthetically practical route for producing the chiral 2,4,5-trisubstituted chiral piperidine was established in addition to the preparation of the 2,4-disubstituted one.
4-2. Stereocontrolled Alkylation of the Aminal Moiety to Produce 2,4,6-Trisubstituted Piperidines
We next planned to synthesize the 2,4,6-trisubstituted piperidines by the stereoselective alkylation of the aminal moiety. In order to realize these alkylations by Grignard reagents in a stereoselective manner, the chemoselective reduction of the C-4 ester group in both 89 and 91 was required. Although LAH and DIBAL-H gave the corresponding diols, fortunately, we found that treatment of the aminal compounds 89 and 91 with Red-Al® at room temperature clearly produced the expected corresponding alcohols in a quantitative manner, which were transformed into the corresponding benzyl ethers 96 and 100. The stereoselective alkylation on the aminal moiety of these compounds was then attempted.7b,33 The reaction of the C-4 β-benzyloxymethyl derivative 96 with MeMgI stereoselectively provided the (2β,4β,6α)- trisubstituted piperidine derivative 97 in 38% yield with a 10 : 1 diastereoselectivity after removal of the hydroxyindane moiety by a lead tetracetate treatment (Scheme 14, a). In this case, the addition of n-propylamine was not necessary probably because of the crowded nature of the piperidine nitrogen. When the C-4 α-hydroxymethyl derivative 98 was treated with methylmagnesium iodide (20 equivalents) and CuI (20 equivalents) in ether, the C-6 α-methyl isomer was obtained in 81% yield as almost a single isomer. The hydroxyindane moiety of the methylated compounds was then removed and the (2β,4α,6α)-trisubstituted piperidine derivative 99 was obtained in 64% yield (Scheme 14, b). The high stereoselectivity of the methylation of 98 could be explained by assuming that the alkylation proceeded through the aggregated species of a methylmetal (Mg or Cu) complex coordinating to the C-4 α-hydroxymethyl group in addition to the hydroxyindane moiety of the iminium ion intermediary (Scheme 15, C). On the other hand, the methylation of benzyl ether 100 with MeMgI mainly proceeded from the opposite site of the C-4 benzyloxymethyl group to give the C-6 β-methyl isomer as a major product in the ratio of 3:1, and a Me3Al treatment of 100 in ether provided the same
C-6 β-isomer with the higher selectivity of 5:1 (Scheme 14). Apparently, the steric factor due to the benzyl protecting group overrode the coordination of the Grignard reagent to the oxygen function at the C-4 position (Scheme 15, D). The removal of the hydroxyindane moiety of the resulting C-6 β-methylated compound provided the (2β,4α,6β)-trisubstituted piperidine derivative 101 in 72% yield (Scheme 14, c). The relative configurations of compounds 97, 99 and 101 were determined based on the NOE in the 1H NMR. Thus, we established a method to synthesize the three chiral 2,4,6-trisubstituted piperidine diasteomers 97, 99 and 101 from the common intermediate (–)-7, which was easily obtainable by the one-pot azaelectrocyclization reaction.
4-3 Novel 1,4-Addition Reaction of the Unsaturated Ester Moiety to Produce 2,3,4-Trisubstituted Piperidines
For the synthesis of the 2,3,4-trisubstituted piperidine compounds, the 1,4-addition reaction of the unsaturated ester (–)-7 was attempted. The treatment of (–)-7 with an excess amount of ethylmagnesium bromide at 0 °C spontaneously provided the dialkylated ester 102 as a mixture of stereoisomers, and no 1,4-addition product 103 was detected. We postulated that the 1,4-addition of an ethyl group to the unsaturated ester moiety would occur after alkylation at the aminal moiety proceeded. We then decided to attempt the 1,4-addition reaction with ethylmagnesium bromide for the aminoindanol derivative 104, which would be obtained from the aminal (–)-7 by chemoselective reduction. Fortunately, we found that the treatment of (–)-7 with NaBH4 in the presence of BF3·OEt2 in THF at 0 °C successfully provided the desired alcohol 104 in 90% yield, which was attempted by the 1,4-addition reaction. The reaction with an excess amount of ethylmagnesium bromide in ether at 0 oC for 20 min cleanly proceeded to produce the 1,4-addition product 105 as a mixture of stereoisomers, which could not be separately isolated at this stage. Removal of the indanol moiety of 105 with lead tetraacetate under the established condition produced a mixture of the 2,3,4-trisubstituted piperidine 106 and its stereoisomer in a 2.7 : 1 ratio, which were cleanly separated from each other. The stereochemistry of the major isomer 106 was determined as 2β, 3α, 4β in the piperidine ring based on an analysis of the NMR data. On the contrary, the reaction of the silyl ether 107 produced the tertiary alcohol 108 with ethylmagnesium bromide. Thus, the secondary hydroxyl group of the aminoindanol moiety was essential for the 1,4-addition reaction.
A plausible mechanism for this highly stereoselective 1,4-addition reaction is shown in Scheme 17. It was postulated that the coordination of the Grignard reagent with the OMgBr group prepared from the Grignard reagent and the hydroxyl group in the cis-aminoindanol moiety was generated during the alkylation process in a manner similar to that of the methylation of 98 (Scheme 14 and 15),33d and then the ethyl group attacked from the opposite direction of the phenyl group as shown in structure 110.
In order to investigate the generality of this novel 1,4-addition reaction assisted by the internal hydroxy group, we applied the reaction to a thiophene derivative 112, which was obtained by the chemoselective reduction of 26 (see Table 2), instead of a phenyl derivative 104 (Table 5, entry 1). The reaction under the same conditions gave the corresponding 1,4-addition product 118 through 115 in 75% yield and a rather better stereoselectivity at the C4 ester group. On the contrary, the attempted 1,4-addition reaction of the corresponding methyl ketone derivative 113, which was easily derived from the amide 114, gave the 1,2-addition product 120 along with the 1,4-addition product 119 in 26% and 41% yields, respectively. In the case of the amide 114, the reaction gave a complex mixture and 1,4-addition product was not isolated. These results indicated that the unsaturated ester group was appropriate for the desired 1,4-addition reaction (Table 5).
Thus, the construction of the 2,3,4-trisubstituted piperidine core from the aminal (¬–)-7 was established by the highly stereoselective 1,4-addition reaction with the Grignard reagent utilizing the novel neighboring participation.
5. SYNTHESIS OF NATURALLY-OCCURRING ALKALOIDS POSSESSING A SUBSTITUTED PIPERIDINE CORE
5-1. Synthesis of Indolizidine Alkaloid, (–)-Dendroprimine, with 2,4,6-Trisubstituted Piperidine Core
In order to demonstrate the established protocol for the polysubstituted piperidine synthesis, we examined the asymmetric synthesis of an indolizidine alkaloid, (–)-dendroprimine (121), which was isolated from Dendrobium primulinum Lindl (Orchidaceae) and characterized by Luning and his co-workers in 1965.27a The relative configuration of the stereogenic centers of dendroprimine was determined by the synthesis of the four racemic diastereomers of this indolizidine alkaloid, and the absolute configuration was also reported by the same group in 1972.27b,27c The enantioselective synthesis of (–)-dendroprimine (121) and its two isomers were reported by Gelas-Mialhe in 2004,34 and the synthesis of (–)-5,7-epidendroprimine was reported by two other groups.35 Our synthetic analysis of (–)-dendroprimine (121) is shown in Scheme 18. We envisioned that dendroprimine (121) could be synthesized from the 2,4,6-trisubstituted piperidine E, which was prepared from the one-pot azaelectrocyclization product 27 by applying the established method for the 2,4,6-trisubstituted piperidine synthesis.
According to the established procedure (see Scheme 14), reduction of the double bond conjugated with the ester of 27 with magnesium in methanol selectively provided the C-4 α-isomer 122 with the ratio of 4 : 1. The relative stereochemistry of the major isomer 122 was unambiguously determined by an X-ray crystallographic analysis. Reduction of the ester group of the isolated 122 with Red-Al® followed by catalytic hydrogenation using PtO2 afforded the corresponding alcohol 123 in 87% yield for 2 steps. The reaction with MeMgI and CuI provided compound 124 with the selectivity of 3 : 1, which was isolated by column chromatography. Obviously, the substituents at the C-2 position of the piperidine ring influenced the stereoselectivity of the methylation (compare 99 and 124 in Scheme 14 and Scheme 19). The removal of the indanol moiety in 124 was achieved by catalytic hydrogenation, and the resulting piperidine nitrogen was protected by a Cbz group in 80% yield for two steps. Although the hydrogenolytic removal of the indanol moiety afforded a better yield than the oxidative condition (Pb(OAc)4/n-PrNH2) in compound 124, the procedure was not effective for the C-2 aryl substrate due to the cleavage of the benzylic C-N bond. Interconversion of the hydroxymethyl group of the obtained 125 to the methyl group of 126 was successful using Puglis’s method.36 Thus, compound 125 was treated with CBr4/PPh3 followed by NaBH4 reduction in DMSO to successfully produce the methyl derivative 126 in 72% yield. The terminal TBS ether group was converted into the corresponding methyl ester 127 by the sequence of removing the TBS, Jones oxidation and esterification. The removal of the Cbz group followed by heating the resulting amine in toluene caused smooth cyclization to produce the corresponding lactam derivative
128.37 Finally, the reduction of the lactam carbonyl group of 128 with LiAlH4 under reflux in ether provided the (–)-dendroprimine (121) (Scheme 19).
The spectral data (1H and 13C NMR) of the synthesized (–)-dendroprimine (121) were in good agreement with those published in the literature.27a,34 Moreover, we also achieved the syntheses of three diastereomers, (+)-5-epidendroprimine, (+)-7-epidendroprimine, and (+)-5,7-epidendroprimine, in addition to correcting the stereochemistry of the previously reported (+)-5-epidendroprimine.38 Especially, in the synthesis of (+)-7-epidendroprimine (135), we could realize the complete stereocontrol as shown in Scheme 20.
Thus, the total syntheses of (–)-dendroprimine (121) and its three steroisomers were achieved by the developed synthetic procedure for the 2,4,6-trisubstituted piperidine core.
5-2. Synthesis of Strychnos-Type Indole Alkaloid, (–)-20-Epiuleine, with 2,3,4-Trisubstituted Piperidine Core
Uleine (136), 20-epiuleine (137), dasycarpidone (138) and 20-epidasycarpidone (139) are four related Uleine (136), 20-epiuleine (137), dasycarpidone (138) and 20-epidasycarpidone (139) are four related members of the Strychnos-type indole alkaloids, which were principally isolated from Aspidosperma sp. (Figure 2).27f
These indole alkaloids lack the two-carbon chain from tryptophan. Although a number of synthetic efforts for the racemic 20-epiuleine (137) and 20-epidasycarpidone (139) along with uleine (136) and dasycarpidone (138) were reported in the literature,38,39 only a few asymmetric syntheses of these indole alkaloids were reported.1a,1b,40 The structural feature of these alkaloids is the 2-aza-bicyclo[3,3,1]nonane skeleton, which could be constructed from the 3-(2-piperidyl)indole derivative 141 by an acid-catalyzed cyclization followed by dehydration.39 The synthesis of (±)-20-epiuleine (137) reported by Husson is shown in Scheme 21. Therefore, the objective was how to efficiently synthesize the optically homogeneous 2-indolyl-3-ethyl-4-carbonyl piperidine compound such as 141, which was classified as a 2,3,4-trisubstituted piperidine skeleton and was obtained in the dl-form by the 1,4-addition of the unsaturated ketone 140 (Scheme 21).39c,39d
Previously, we reported the formal synthesis of the optically active 20-epiuleine (137) utilizing the step-wise asymmetric 6π-azaelectrocyclization.1a,1b In that formal synthesis, although we synthesized the optically homogeneous 2-indolyl-4-acetyl-1,2,5,6-tetrahydropyridine 140, the transformation to the corresponding chiral 2,3,4-trisubstituted piperidine derivative 141 was not realized. Thus, the procedure for the construction of the chiral 2,3,4-trisubstituted piperidine framework was not yet established. The first synthetic trial of the chiral 2,3,4-trisubstituted piperidine 141 is shown in Scheme 22. The transformation from 21 to the methyl ketone 140 through 142 was accomplished by the sequence of DIBAL-H reduction, lead tetraacetate oxidation in the presence of n-propylamine, reductive N-methylation (HCHO, NaBH3CN in CH3CN), MnO2 oxidation, methylation with MeLi, hydrolysis of the N-tosyl group in the indole ring and then DMP oxidation as previously reported.1a,1b We thus obtained the optically active methyl ketone derivative 140, which was the key synthetic intermediate of (±)-20-epiuleine (137) as reported by Husson’s group. We then attempted the 1,4-addition reaction of (–)-140 under the reaction conditions described in the literature.38c After a detailed investigation of the reaction conditions, we obtained the desired 2,3,4-trisubstituted piperidine compound 141 in 48% yield using excess reagents of ethylmagnesium bromide and copper(I) chloride in the ratio of 2.5:1 in ether. Although we obtained 141, this 1,4-addition reaction was troublesome and sometimes not reproducible. The yield varied from 48% to 13% under the same employed conditions. We then examined a new 1,4-addition reaction method, and successfully developed a novel 1,4-addition reaction assisted by the neighboring hydroxyl group participation as mentioned above (chapter 4-3).
We then applied the newly developed method for the 1,4-addition reaction to the total synthesis of (–)-20-epiuleine (137). Chemoselective reduction of the aminal moiety of 21, which was easily obtained by the one-pot asymmetric azacyclization, with NaBH4 in the presence of BF3·OEt2 as previously mentioned, produced the expected alcohol 143. The reaction of 143 with an excess amount of ethylmagnesium bromide under the established conditions cleanly proceeded to produce the expected 1,4-addition product 144 in 95% yield as a mixture of stereoisomers, which could not be isolated from one another at this stage (Scheme 23). Removal of the indanol moiety in 144 with lead tetraacetate produced the 2,3,4-trisubstituted piperidines 145a and 145b in 24% and 71% yields, respectively, which were cleanly separated from each other. The stereochemistry of 145b was determined as 2β, 3α, 4β in the piperidine ring and that of 145a as 2β, 3α, 4α by the detailed analysis of the NMR data. Although isomerization of the corresponding methyl ketone 141 was successful in the literature,39c,d various attempts of isomerization at the ester group of 145a (t-BuOK in t-BuOH, DBU in DMF and so on) were not successful. Therefore, the obtained 145 was used as a mixture for the next step and transformed into the methyl ketone 141. Thus, the reductive N-methylation of a mixture of 145b and 145a by treatment with a 37% aqueous HCHO solution and sodium cyanoborohydride in acetonitrile produced 146b and 146a as a mixture of the easily separable epimers. Each of them was transformed into the aldehydes 147b and 147a by DIBAL-H reduction followed by Swern oxidation. At this stage, the undesired isomer 147a was epimerized to the desired 147b by a DBU treatment in THF at room temperature. The obtained aldehyde 147b was transformed into the methyl ketone 141 through 148 by methylation with MeLi, Swern oxidation and then hydrolysis of the N-tosyl group of the indole moiety with Cs2CO3 in THF and MeOH. Finally, the treatment of 141 with p-TsOH completed the total synthesis of (–)-20-epiuleine (137) in 25% yield. The spectral data (1H NMR, 13C NMR, IR, HRMS) were in good agreement with those of the natural product. The value of [α]D of the synthesized compound was –30.1 (CHCl3, c 0.9), which was reported for the first time.
6. CONCLUSION
In conclusion, we realized the efficient synthesis of chiral piperidines, which possess three different substituents (2,4,5-, 2,4,6- and 2,3,4-substituted piperidines), by utilizing the highly efficient and stereoselective one-pot azaelectrocyclization protocol. The one-pot reaction proceeded by the simple mixing and heating of three components, i.e., vinyl iodides, cis-aminoindanols and vinyl stannanes, in the presence of a palladium catalyst, with a high stereoselectivity and satisfactory yield. By applying this protocol, the total syntheses of (–)-dendroprimine, an indolizidine alkaloid containing the 2,4,6-trisubstituted piperidine motif, and (–)-20-epiuleine, a strychos-type indole alkaloid containing the 2,3,4-trisubstituted piperidine motif, were achieved. Thus, the one-pot asymmetric 6π-azaelectrocyclization can be regarded as a powerful reaction for the synthetic strategy of alkaloids possessing 2,4,5-, 2,4,6-, and 2,3,4-substituted piperidine cores. Further applications toward related natural alkaloids are currently being pursued in our laboratory.
ACKNOWLEDGEMENT
The authors are grateful to all the co-authors of the references cited in this review. The work cited in this review was financially supported by Grants-in-Aid for Scientific Research on Priority Areas 16073222 from the Ministry of Education, Culture, Sports, Science and Technology, Japan, and JSPS (Research Fellowship for T.K.).
References
1. (a) K. Tanaka and S. Katsumura, J. Am. Chem. Soc., 2002, 124, 9660; CrossRef (b) K. Tanaka, T. Kobayashi, H. Mori, and S. Katsumura, J. Org. Chem., 2004, 69, 5906; CrossRef (c) K. Tanaka and S. Katsumura, J. Synth. Org. Chem. Jpn., 2005, 63, 697; (d) T. Kobayashi, M. Nakashima, T. Hakogi, K. Tanaka, and S. Katsumura, Org. Lett., 2006, 8, 3809; CrossRef (e) T. Kobayashi, F. Hasegawa, K. Tanaka, and S. Katsumura, Org. Lett., 2006, 8, 3813; CrossRef (f) Y. Li, T. Kobayashi, and S. Katsumura, Tetrahedron Lett., 2009, 50, 4482; CrossRef (g) T. Kobayashi, K. Takeuchi, J. Miwa, H. Tsuchikawa, and S. Katsumura, Chem. Commun., 2009, 3363; CrossRef (h) T. Sakaguchi, S. Kobayashi, and S. Katsumura, Org. Biomol. Chem., 2011, 9, 257. CrossRef
2. (a) E. N. Marvell, G. Caple, B. Schatz, and W. Pippin, Tetrahedron, 1973, 29, 3781; CrossRef (b) E. N. Marvell, G. Caple, C. Delphey, J. Platt, N. Polston, and J. Tashiro, Tetrahedron, 1973, 29, 3797. CrossRef
3. C. W. Spangler, T. P. Jondahl, and B. Spangler, J. Org. Chem., 1973, 38, 2478. CrossRef
4. (a) J. A. Palenzuela, H. Y. Elnagar, and W. H. Okamura, J. Am. Chem. Soc., 1989, 111, 1770; CrossRef (b) G.-D. Zhu and W. H. Okamura, Chem. Rev., 1995, 95, 1877; CrossRef (c) W. H. Okamura and A. R. de Lera, In Comprehensive of Organic Synthesis, ed. by B. M. Trost, I. Fleming, and L. A. Paquette, Volume Ed., Pergamon Press: London, 1991; Vol. 5, Chapter 6.2, pp. 699-750.
5. D. F. Maynard and W. H. Okamura, J. Org. Chem., 1995, 60, 1763. CrossRef
6. (a) E. N. Marvell, Thermal Electrocyclic Reactions, Academic Press: New York, 1980; (b) P. Schiess, H. L. Chia, and P. Ringele, Tetrahedron Lett., 1972, 313; CrossRef (c) Y.-S. Cheng, A. T. Lupo Jr., and F. W. Fowler, J. Am. Chem. Soc., 1983, 105, 7696; CrossRef (d) M. J. Wyle and F. W. Fowler, J. Org. Chem., 1984, 49, 4025. CrossRef
7. Recent examples: (a) M. L. Meketa and S. M. Weinreb, Org. Lett., 2006, 8, 1443; CrossRef (b) K. Umetsu and N. Asao, Tetrahedron Lett., 2008, 49, 2722; CrossRef (c) D. Tejedor, G. Mendez-Abt, and F. Garcia-Tellado, Chem. Eur. J., 2010, 16, 428. CrossRef
8. A. R. de Lera, W. Reischl, and W. H. Okamura, J. Am. Chem. Soc., 1989, 111, 4051. CrossRef
9. (a) K. Tanaka, H. Mori, S. Fujii, Y. Itagaki, and S. Katsumura, Tetrahedron Lett., 1998, 39, 1185; CrossRef (b) K. Tanaka, M. Kamatani, H. Mori, S. Fujii, K. Ikeda, M. Hisada, Y. Itagaki, and S. Katsumura, Tetrahedron, 1999, 55, 1657; CrossRef (c) K. Tanaka and S. Katsumura, J. Synth. Org. Chem. Jpn., 1999, 57, 876. CrossRef
10. K. Tanaka, H. Mori, M. Yamamoto, and S. Katsumura, J. Org. Chem., 2001, 66, 3099. CrossRef
11. K. Tanaka and S. Katsumura, Org. Lett., 2000, 2, 373. CrossRef
12. Recent progress in pyridine synthesis: (a) T. Kobayashi, S. Hatano, H. Tsuchikawa, and S. Katsumura, Tetrahedron Lett., 2008, 49, 4349; CrossRef (b) T. Sakaguchi, T. Kobayashi, S. Hatano, H. Tsuchikawa, K. Fukase, K. Tanaka, and S. Katsumura, Chem. Asian J., 2009, 4, 1573. CrossRef
13. (a) R. P. Hsung, L.-L. Wei, H. M. Sklenicka, C. J. Douglas, M. J. McLaughlin, J. A. Mulder, and L. J. Yao, Org. Lett., 1999, 1, 509; CrossRef (b) H. M. Sklenicka, R. P. Hsung, L.-L. Wei, M. J. McLaughlin, A. I. Gerasyuto, and S. J. Degen, Org. Lett., 2000, 2, 1161; CrossRef (c) H. M. Sklenicka, R. P. Hsung, M. J. McLaughlin, L.-L. Wei, A. I. Gerasyuto, and W. B. Brennessel, J. Am. Chem. Soc., 2002, 124, 10435; CrossRef (d) M. J. McLaughlin, R. P. Hsung, K. P. Cole, J. M. Hahn, and J. Wang, Org. Lett., 2002, 4, 2017; CrossRef (e) S. Luo, C. A. Zificsak, and R. P. Hsung, Org. Lett., 2003, 5, 4709. CrossRef
14. See review: (a) A. Dömling and I. Ugi, Angew. Chem. Int. Ed., 2000, 39, 3168; CrossRef (b) D. J. Ramon and M. Yus, Angew. Chem. Int. Ed., 2005, 44, 1602; CrossRef For recent examples see: (c) U. Kusebauch, B. Beck, K. Messer, E. Herdtweck, and A. Dömling, Org. Lett., 2003, 5, 4021; CrossRef (d) L. Banfi, A. Basso, G. Guanti, and R. Riva, Tetrahedron Lett., 2004, 45, 6637; CrossRef (e) M. M. S. Timmer, P. M. D. Risseeuw, D. V. Filippov, J. R. Plaisier, G. A. Marel, H. S. Overkleeft, and J. H. Boom, Tetrahedron: Asymmetry, 2005, 15, 177; CrossRef (f) A. Basso, L. Banfi, R. Riva, and G. Guanti, J. Org. Chem., 2005, 70, 575; CrossRef (g) M. Marigo, T. Schulte, J. Franzen, and A. K. Jørgensen, J. Am. Chem. Soc., 2005, 127, 15710; CrossRef (h) S. Suga, D. Yamada, and J. Yoshida, Chem. Lett., 2010, 39, 404. CrossRef
15. V. Farina, V. Krishnaurthy, and W. J. Scott, The Stille Reaction, John Wiley & Sons, Inc.: New York, 1998.
16. (a) G. Langli, L.-L. Gundersen, and F. Rise, Tetrahedron, 1996, 52, 5625; CrossRef (b) V. Farina and B. Krishnan, J. Am. Chem. Soc., 1991, 113, 9585. CrossRef
17. H. Suginome, H. Umeda, and T. Masamune, Tetrahedron Lett., 1970, 11, 4571. CrossRef
18. M. J. Frisch, G. W. Trucks, H. B. Schlegel, G. E. Scuseria, M. A. Robb, J. R. Cheeseman, V. G. Zakrzewski, J. A. Montgomery, Jr., R. E. Stratmann, J. C. Burant, S. Dapprich, J. M. illam, A. D. Daniels, K. N. Kudin, M. C. Strain, O. Farkas, J. Tomasi, V. Barone, M. Cossi, R. Cammi, B. Mennucci, C. Pomelli, C. Adamo, S. Clifford, J. Ochterski, G. A. Petersson, P. Y. Ayala, Q. Cui, K. Morokuma, D. K. Malick, A. D. Rabuck, K. Raghavachari, J. B. Foresman, J. Cioslowski, J. V. Ortiz, B. B. Stefanov, G. Liu, A. Liashenko, P. Piskorz, I. Komaromi, R. Gomperts, R. L. Martin, D. J. Fox, T. Keith, M. A. Al-Laham, C. Y. Peng, A. Nanayakkara, C. Gonzalez, M. Challacombe, P. M. W. Gill, B. G. Johnson, W. Chen, M. W. Wong, J. L. Andres, M. Head-Gordon, E. S. Replogle, and J. A. Pople, Gaussian 03, Gaussian, Inc.: Pittsburgh, PA, USA, 2003, http://www.gaussian.com/.
19. F. Mohamadi, N. G. J. Richards, W. C. Guida, R. Liskamp, M. Lipton, C. Caufield, G. Chang, T. Hendrickson, and W. C. Still, J. Comput. Chem., 1990, 11, 440. CrossRef
20. Tetrasubstituted vinyl iodides 46 and 60 were prepared as follows.
21. We tried to crystallize both the methyl and ethyl ester derivatives, and happened to obtain a better single crystal of the ethyl ester derivative for X-ray analysis.
22. The formation of the lactam 57 was confirmed through the reaction between aminoindanol (–)-1 or (–)-9 and vinyl iodide 46 in 1,4-dioxane at 80 °C.
23. T. Kobayashi, K. Tanaka, J. Miwa, and S. Katsumura, Tetrahedron: Asymmetry, 2004, 15, 185. CrossRef
24. (a) P. G. Gassman, J. A. Ray, P. G. Wenthold, and J. W. Mickelson, J. Org. Chem., 1991, 56, 5143; CrossRef (b) E. D. Thorsett and F. R. Stermiz, Synth. Commun., 1972, 2, 375. CrossRef
25. Compound 85 was also synthesized from the phthalic anhydride and MeMgBr followed by reduction and then hydration, see B. B. Elsner, H. E. Strauss, and E. J. Forbes, J. Chem. Soc., 1957, 578. CrossRef
26. Unpublished data.
27. Dendroprimine: (a) B. Luning and K. Leander, Acta Chem. Scand., 1965, 19, 1607; CrossRef (b) B. Luning and C. Lundin, Acta Chem. Scand., 1967, 21, 2136; CrossRef (c) L. Blomquist, K. Leander, B. Luning, and J. Rosenblom, Acta Chem. Scand., 1972, 26, 3203.; CrossRef Hippodamine: (d) B. Tursch, D. Daloze, J. M. Pasteels, A. Caravador, J. C. Braekman, C. Hootele, and D. Zimmermann, Bull. Soc. Chim. Belg., 1972, 81, 649; (e) B. Tursch, D. Daloze, J. C. Braekman, C. Hootele, A. Caravador, D. Losman, and R. Karlsson, Tetrahedron Lett., 1974, 409; CrossRef Uleine: (f) J. Bosch and J. Bonjoch, Pentacyclic Strychnos Indole Alkaloids. In Studies in Natural Products Chemistry; ed by Atta-ur-Rahman; Elsevier: Amsterdam, 1988; Vol. 1, pp. 31-88; Guettardine: (g) M. H. Brillanceau, S. Kan-Fan, and H.-P. Husson, Tetrahedron Lett., 1984, 26, 2767; CrossRef Protoemetine: (h) A. R. Battersby, G. C. Davidson, and B. J. T. Harper, Chem. Ind., 1957, 983; (i) A. R. Battersby and B. J. T. Harper, J. Chem. Soc., 1959, 1748. CrossRef
28. Reviews of piperidine syntheses was recently reported: (a) M. G. P. Buffat, Tetrahedron, 2004, 60, 1701; CrossRef (b) C. Escolano, M. Amat, and J. Bosch, Chem. Eur. J., 2006, 12, 8189; CrossRef (c) M. Amat, M. Perez, and J. Bosch, Synlett, 2011, 143. CrossRef
29. (a) E. Poupon, D. Francois, N. Kunesch, and H.-P. Husson, J. Org. Chem., 2004, 69, 3836; CrossRef (b) K. M. Goodenough, W. J. Moran, P. Raubo, and P. A. Harrity, J. Org. Chem., 2005, 70, 207; CrossRef (c) J. Monfray, Y. Gelas-Mialhe, J.-C. Gramain, and R. Remuson, Tetrahedron: Asymmetry, 2005, 16, 1025; CrossRef (d) A. P. Dobbs and S. J. Guesne, Synlett, 2005, 2101; CrossRef (e) D. Passarella, A. Barilli, F. Belinghieri, P. Fassi, S. Riva, A. Sacchetti, A. Silvani, and B. Danieli, Tetrahedron: Asymmetry, 2005, 16, 2225; CrossRef (f) J. Gebauer and S. Blechert, Synlett, 2005, 2826; CrossRef (g) R. P. Wung and G. C. S. Fu, J. Am. Chem. Soc., 2005, 127, 12234; CrossRef (h) M. Sales and A. B. Charette, Org. Lett., 2005, 7, 5773; CrossRef (i) L. C. Pattenden, R. A. J. Wybrow, S. A. Smith, and J. P. A. Harrity, Org. Lett., 2006, 8, 3089; CrossRef (j) M. Terada, K. Machioka, and K. Sorimachi, J. Am. Chem. Soc., 2007, 129, 10336; CrossRef (k) N. Sarkar, A. Banerjee, and S. G. Nelson, J. Am. Chem. Soc., 2008, 130, 9222; CrossRef (l) Y. Hayashi, H. Gotoh, R. Masui, and H. Ishikawa, Angew. Chem. Int. Ed., 2008, 47, 4012; CrossRef (m) M. Ahari, A. Perez, C. Menant, J.-L. Vasse, and J. Szymoniak, Org. Lett., 2008, 10, 2473; CrossRef (n) M. A. Fernandez-Ibanez, B. Macia, M. G. Pizzuti, A. J. Minnaard, and B. L. Feringa, Angew. Chem. Int. Ed., 2009, 48, 9339; CrossRef (o) M. Z. Chen and G. C. Micalizio, Org. Lett., 2009, 11, 4982; CrossRef (p) N. P. McLaughlin and P. Evans, J. Org. Chem., 2010, 75, 518; CrossRef (q) S. B. D. Jarvis and A. B. Charette, Org. Lett., 2011, 13, 3830. CrossRef
30. For examples: (a) Y. Hirai, T. Terada, Y. Okaji, T. Yamazaki, and T. Momose, Tetrahedron Lett., 1990, 33, 4755; CrossRef (b) M. Amat, M. Perez, N. Llor, C. Escolano, F. F. Luque, E. Molins, and J. Bosch, J. Org. Chem., 2004, 69, 8681. CrossRef
31. In this reaction, the β-isomer was changed into the corresponding methyl ester.
32. Unpublished data.
33. Reduction and alkylation of aminal. See; (a) M. Yamato, K. Hashigaki, S. Ishikawa, and N. Qais, Tetrahedron Lett., 1988, 29, 6949; CrossRef (b) K. Higashiyama, H. Inoue, and H. Takahashi, Tetrahedron Lett., 1992, 33, 235; CrossRef (c) K. Higashiyama, H. Inoue, and H. Takahashi, Tetraahedron, 1994, 50, 1083; CrossRef (d) K. Higashiyama, K. Nakahata, and H. Takahashi, J. Chem. Soc., Perkin Trans. 1, 1994, 351; CrossRef (e) K. Higashiyama, H. Kyo, and H. Takahashi, Synlett, 1998, 489; CrossRef (f) H.-P. Husson and J. Royer, Chem. Soc. Rev., 1999, 28, 383. CrossRef
34. A. D. S. Bollena, Y. Gelas-Mialhe, J.-C. Gramain, A. Perret, and R. Remuson, J. Nat. Prod., 2004, 67, 1029. CrossRef
35. (a) M. Diederich and U. Nubbrmeyer, Synthesis, 1999, 286; CrossRef (b) R.-T. Hsu, L.-M. Cheng, N.-C. Chang, and H.-M. Tai, J. Org. Chem., 2002, 67, 5044. CrossRef
36. R. O. Hutchins, D. Kandasamy, F. Dux III, C. A. Maryanoff, D. Rotstein, B. Goldsmith, W. Burgoyne, F. Cistone, J. Dalessandro, and J. Puglis, J. Org. Chem., 1978, 43, 2259. CrossRef
37. B. Herradon and F. Sanchez-Sancho, Tetrahedron: Asymmetry, 1998, 9, 1951. CrossRef
38. Synthesis of uleine: (a) A. Jackson, N. D. V. Wilson, A. J. Gaskell, and J. A. Joule, J. Chem. Soc. C, 1969, 2738; CrossRef (b) G. Büchi, S. J. Gould, and F. Näf, J. Am. Chem. Soc., 1971, 93, 2492; CrossRef (c) T. Kametani and T. Suzuki, J. Org. Chem., 1971, 36, 1291. CrossRef
39. Synthesis of 20-epiuleine: (a) L. J. Dolby and H. Biere, J. Org. Chem., 1970, 35, 3843; CrossRef (b) M. Natsume and Y. Kitagawa, Tetrahedron Lett., 1980, 21, 839; CrossRef (c) M. Harris, R. Besselievre, D. S. Grierson, and H.-P. Husson, Tetrahedron Lett., 1981, 22, 331; CrossRef (d) D. S. Grierson, M. Harris, and H.-P. Husson, Tetrahedron, 1983, 39, 3683; CrossRef (e) E. S. Tasber and R. M. Garbaccio, Tetrahedron Lett., 2003, 44, 9185. CrossRef
40. M. Saito, M. Kawamura, K. Hiroya, and K. Ogasawara, Chem. Commun., 1997, 765; CrossRef Enantioselective synthesis of strychnos indole alkaloids and related compounds: J. Bonjoch and D. Sole, Chem. Rev., 2000, 100, 3455. CrossRef