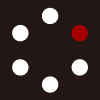
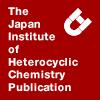
HETEROCYCLES
An International Journal for Reviews and Communications in Heterocyclic ChemistryWeb Edition ISSN: 1881-0942
Published online by The Japan Institute of Heterocyclic Chemistry
e-Journal
Full Text HTML
Received, 18th December, 2012, Accepted, 11th January, 2013, Published online, 15th January, 2013.
DOI: 10.3987/COM-12-12653
■ Phosphonated Bicycles Bearing a N-N Junction
Elise Villemin, Marie-France Herent, and Jacqueline Marchand-Brynaert*
Institute of Condensed Matter and Nanosciences (IMCN), Université Catholique de Louvain, Bâtiment Lavoisier, place Louis Pasteur 1, B-1348 Louvain-la-Neuve , Belgium
Abstract
The Diels-Alder reaction between diethyl 1-phosphono-1,3-butadiene and cyclic azo dienophiles, such as 4-phenyl- and 4-methyl-1,2,4-triazoline-3,5-diones and phthalazine-1,4-dione gave access to phosphonated bicyclic cycloadducts with a nitrogen-nitrogen junction. Various fonctionalizations (dihydroxylation, hydrogenation and phosphonic ester deprotection) have been performed with success. The selective N-N cleavage was not possible for the preparation of large heterocycles. The coordination properties of selected bicycles were tested by ESI-HRMS.INTRODUCTION
Molecules containing an aza-cyclic structure are of interest in medicinal chemistry. The synthesis of aminosugars, with a N-N motif, has been developed in the last decade. M. Bols et al. have demonstrated the biological activities of (-)-1-azafagomine, as a potent competitive inhibitor of almond β-glucosidase, yeast α-glucosidase and isomaltase (Figure 1, structure A).1 Piperazine-3-carboxylic acid2 (Figure 1, structure B) has been used for the preparation of biologically active molecules such as monamycin (antibacterial),3a azinothricin3b (antitumoral), chloptosin3c and lydiamycin3d derivatives. Aminophosphonic acids have been prepared as analogs of aminocarboxylic acids used for serine-enzymes inhibition; due to the tetrahedral geometry of the phosphonate group, it is able to mimic the transition state of nucleophilic addition to a carbonyl function. Another advantage of the phosphonate function is its capacity to modify the hydrosolubility of the drug.4 Such molecules have recently demonstrated a good protective effect against the phytotoxic action of chloroacetanilide herbicide.5 Two different approaches, involving an hetero-Diels-Alder (DA) reaction with dialkyl azodicarboxylates as dienophiles, have been published: either the phosphonate ester group was introduced after the DA reaction with 1-methoxy-l,3-butadiene, by nucleophilic substitution via Lewis acid-catalyzed phosphonylation,6 or the phosphonate ester group was already present before the DA reaction by using diethyl 1-phosphono-1,3-butadiene as diene.7 This second approach, developed in our laboratory, gave better yields of molecule C (Figure 1) in two steps from the phosphonodiene.7
Macrocycles including amine and lactam functions constitute a family of alkaloids derived from spermine and spermidine molecules, endowed with interesting biological activities such as antibiotics and hypertensives (Figure 1, structure D).8 Among the different strategies of total synthesis for making these macrocycles, N-N cleavage of bicycles, followed by a ring enlargement was considered as an alternative route to transamidation strategies.8
4-Phenyl-1,2,4-triazoline-3,5-dione (noted PTAD) is described in the literature as a very reactive dienophile in the DA reaction with carbonated dienes, and as a good enophile in the ene-reaction in the presence of a hydrogen acceptor group.9 PTAD is more reactive in the [4+2] cycloaddition, compared to acyclic azo dienophiles, due to its cyclic structure and its fixed cis configuration facilitating the diene approach. Dienes with particular structures and heterodienes were able to react with PTAD.10 The N-N junction of the resulting bicycles was never cleaved in order to obtain macrocycles. Contrary to the O-N bond (oxazine),11 the cleavage of the N-N bond requires more drastic conditions: the most widespread methodologies for N-N cleavage, with one or both nitrogen atoms involved in an imide/amide function, are the hydrogenolysis in the presence of Raney nickel,12 and the reduction with an alkaline metal such as metallic sodium or lithium.13 No example was found where the N-N junction is part of a bisurea function. The Diels-Alder reaction/N-N reductive cleavage sequence is rarely used, due to the difficulty to perform the second step. The strained bicyclic hydrazines, obtained by [4+2] cycloaddition of cyclopentadiene to acyclic azo compounds,14 and bridgehead bicyclic triazines obtained by intramolecular cycloaddition of N-acylazo compounds,15 are the exceptions where the N-N bond could be easily cleaved.
Phthalazine-1,4-dione has also been used in DA reactions as heterodienophile and this motif is present in a few biologically active molecules. A [4+2] cycloaddition was reported with a diene derived from Grundmann’s ketone,16 affording azasteroids with a promising cytotoxicity on HL-60 tumor cells.17 Cycloadduct of (E)-methyl penta-2,4-dienoate gave after hydrolysis of the ester group, a Cilazapril precursor (Figure 1, structure E).18 Catalytic hydrogenation of azabicyclic adducts derived from [4+2] cycloaddition of phthalazine-1,4-dione and cyclo-1,3-hexadiene or cyclo-1,3,5-heptatriene gave compounds with antiarrhythmia properties (Figure 1, structure F).19
The aim of this article is the study of cycloadducts obtained by DA reaction between 1-phosphono-1,3-butadiene (1) and cyclic azodienophiles, such as PTAD (2a) and 4-methyl-1,2,4-triazoline-3,5-dione (2b) and phthalazine-1,4-dione (10),20 their further functionalization and particularly the cleavage of the N-N cyclic junction for the preparation of novel large heterocycles as potential cage-ligands of cations.21 The coordination properties of the bicyclic precursors are also of interest.
RESULTS AND DISCUSSION
During our study of scope and limitations of the DA reaction of diethyl 1-phosphono-1,3-butadiene (1),11 we have observed that the reactivity of this diene is close to the carboxylate analogs. The [4+2] cycloaddition between 1 and 2a,b, under microwave activation, gave access to bicyclic cycloadducts bearing a N-N junction (Scheme 1) similarly to methyl 2,4-pentadienoate1a and its chiral derivative22. It is to note that in our case, no side-products formed from [2+2] cycloaddition were observed. The advancement of the reaction between 2a and the diene 1 was directly observable by the disappearance of the PTAD red color. The cycloadduct 3a,b were isolated in good yields.
The selective deprotection of the phosphonic ester by treatment with trimethylsilyl bromide followed by a methanolysis (McKenna method23) gave the phosphonic acids 4a,b with high yields. The syn-dihydroxylation of the C=C double bond of the cycloadducts 3a,b could be performed by treatment with osmium tetroxide salt and N-methyl morpholine oxide (NMO). The relative stereochemistry of the diols 5a,b was attributed on the basis of typical NMR coupling constants.7 Interestingly, the deprotection method of the phosphonate ester worked well on the functionalized cycloadducts 5a to furnish the corresponding phosphonic acid derivative 6a in moderate yield.
No reaction occurred, or degradation was observed, when using peracids (m-chloroperoxybenzoic acid, acetic peracid) or peroxides (hydrogen peroxide, trimethylsilyl peroxide) as oxidative agents. Small amounts of epoxide 7 were formed with sodium dioxide in ethanol at 0 °C, but the experiment revealed poorly reproducible. The hydrazinolysis of the cycloadducts 3 gave not the expected bicyclic structure 8, but an open structure by addition of hydrazine on one C=O group. Smooth conditions of catalytic hydrogenation allowed the selective reduction of the C=C double bond to afford the cycloadducts 9a,b (Scheme 1).
The direct N-N bond cleavage of cycloadducts 3 has been tested. Conditions required for oxazine bond cleavage (zinc in acetic acid or samarium diiodide)11 failed in our case, and the cycloadducts 3 were retrieved intact. Rare examples of N-N bond cleavage by samarium diiodide have been found in the literature: N-benzoylhydrazines and N-trifluoroacetylhydrazines are cleaved by this reagent.24
Hydrogenation in the presence of catalysts such as palladium or platine on charcoal in neutral or acidic medium and Raney Nickel in ethanol gave the reduced cycloadducts 9 contaminated by various amounts of unidentified products.
Lebrun et al. described N-N bond cleavage of molecules bearing a phenyl substituent in α-position or on a nitrogen atom, using photochemistry.25 The cycloadducts 3 were unreactive under irradiation at 254 nm in i-PrOH or toluene/n-hexane at room temperature. Reduction of 9 with sodium in liquid ammonia gave a lot of unidentified products: the phosphonic ester group and the bicyclic structure were totally destroyed. No reaction was observed when treating the cycloadducts 9 by magnesium in acidic medium.
The preliminary reduction of the imide carbonyl functions seemed necessary to allow the further N-N bond cleavage by hydrogenolysis.26 The cleavage of a N-N bond at the junction of bicyclic structures has been observed on α-aminoammonium ions with lithium aluminium hydride as reductive agent.27 In addition, S. Grabowski et al. proposed the conversion of the urazole ring of a functionalized cycloadduct from PTAD into an azo bridge in order to easily cleave the N-N bond by hydrogenation in the presence of platinium or Raney Nickel.28 In our case, the reduction of the carbonyl groups by the action of hydrides was unfortunately not compatible with the presence of a phosphonic ester function.
All our attempts of N-N bond cleavage from precursors 3 or 9 failed; the access to large heterocycles by the “hetero Diels-Alder cycloaddition/N-N bond reductive cleavage” strategy appeared to be non applicable in the case of the phosphonated compounds.
The same conclusions could be drawn with the cycloadduct 11 resulting from the DA reaction of 1 and phthalazine-1,4-dione (10) prepared in situ by oxidation of phthalhydrazide. The adjustment of the experimental conditions was delicate because the dienophile 10 is thermally unstable and the phosphonodiene 1 is poorly reactive at low temperature. Thus the Clement’s method29 was chosen for the synthesis of 10, i.e. lead tetraacetate at 0-5 °C, instead of the usual treatment by t-butyl hypochlorite at -50 °C,30 and 10 was directly trapped with the diene 1 at 0 °C to afford the cycloadduct 11 in quite modest yield. The reductive cleavage of the N-N bond, without destruction of the molecular skeleton, could not be achieved. However, the selective reduction of the C=C double bond was performed by smooth hydrogenation, giving the cycloadduct 12, and the syn-dihydroxylation of the C=C double bond was obtained by osmylation treatment, furnishing the diol 13 (Scheme 2).
The coordination properties of the phosphonated tricyclic compounds 12 and 13 have been evaluated in solution, using the high resolution mass spectrometry (HRMS) in the electrospray ionisation (ESI) as the analytical tool. These representative ligands (L) were considered for coordination with di- and trivalent metal (M) cations in ethanol. Solutions of ligand (0.5 mM) were treated with 5 equiv. of anhydrous M(II)(ClO4)2 or M(III)(NO3)3 salts, and the mixtures were analyzed by HRMS in the ESI mode, a mild method allowing the transfer of the complexes in the gas phase with the conservation of noncovalent bonds. All of the complexes identified were monocharged and possessed one or two poorly coordinating counteranion(s), that is, one perchlorate anion in the case of a divalent metal and two nitrate anions in the case of a trivalent metal. The complexes stoichiometries for cycloadducts 12 and 13 were [1L:1M] (with or without an additional solvent molecule) and [2L:1M]. The results collected in Tables 1 and 2 give the relative percentages of [1L:1M] and [2L:1M] complexes formed in EtOH, considering the most abundant complex (for each experiment) as 100%. The corresponding atomic compositions and exact masses are given in the Supporting Information. [1L:2M] complexes were never observed in the case of ligand 13: so we conclude, that the diol function has no coordination ability; the carbonyl and phosphoryl functions are the only efficient groups for coordinating metallic cations, such as in ligand 12. A qualitative scale of coordination efficiency could be deduced from the ratios of formed complexes versus the free metal (see Supporting Information): for both ligands 12 and 13, the divalent cations were more easily coordinated than the trivalent ones. The order of coordination was Mg2+ > Co2+ > Ni2+ > Zn2+ > Fe2+ > Ca2+ for 12 and Fe2+ > Co2+ > Ni2+ > Zn2+ > Mg2+ > Ca2+ for 13. As examples the coordination of Fe(II) and Eu(III) cations is illustrated by their respective HRMS spectra given in Supporting Information.
CONCLUSIONS
Hetero-Diels-Alder reaction between diethyl 1-phosphono-1,3-butadiene and cyclic azo dienophiles gave access to novel phosphonated bicycles bearing a N-N junction. In spite of many attempts to cleave the N-N bond, macrocycles have not been obtained. Selective functionalizations, such as deprotection of phosphonic ester group and syn-dihydroxylation, gave functionalized cycloadducts with moderate to good yields. From a HRMS-ESI study, the ligands 12 and 13 presented interesting coordination properties for divalent cations. By comparison with the ligand 3a,20 the observed [1L:1M] and [2L:1M] coordination complexes confirmed, that the phosphoryl and carbonyl functions coordinate well the tested metallic cations. The percentages of [2L:1M] complexes could not be correlated with the ionic radii of the metals, nor with their hardness. A possible coordination mode is depicted in the Figure 2.
EXPERIMENTAL
Materials and Methods: Experiments were performed under an atmosphere of dry argon. The chemicals purchased from Acros Organic, Alfa Aesar and Sigma-Aldrich were of reagent grade and used without purification. TLC analyses were performed on aluminium plates coated with silica gel Merck 60 F-254 and flash column chromatographies over silica gel (230-400 mesh). Visualization of TLC plates was performed under a UV lamp (254 nm) and using p-anisaldehyde or KMnO4. Analytical grade solvents were used for reactions and solvents used for column chromatographies were technical solvents distilled before use. Reactions under microwave heating were conducted in sealed tubes using MicroSYNTH equipment (Milestone Srl). NMR spectra were recorded on a Bruker AVANCE II 300 spectrometer operating at 300 MHz for 1H, 75 MHz for 13C and 121 MHz for 31P, and on a Bruker AVANCE II 500 spectrometer operating at 500 MHz for 1H, 125 MHz for 13C, 202 MHz for 31P. Chemical shifts were reported in ppm from tetramethylsilane as the internal standard (δ = 0.0 ppm) for 1H spectra. The internal standard was the deuterated chloroform for the 13C spectra (δ = 77.16 ppm). 31P downfield shifts (δ) were expressed with a positive sign, using 85% H3PO4 in H2O as external standard. The atoms numbering used for NMR description is given in Schemes 1 and 2; the dienyl protons were assigned as the proton number 1 to 4 because this motif was common for all cycloadducts. Melting points (mp) were determined on a Büchi B-540 apparatus calibrated with caffeine, vanillin and phenacetin. Infrared spectra (IR) were recorded by transmittance with a Shimadzu FTIR-8400S equipment and the absorption bands are reported in cm-1. Products were analyzed as thin films deposited on a Se-Zn crystal by evaporation from CHCl3 solutions. The intensity of peaks was noted by (w), (m) and (s), respectively for weak, medium and strong. Mass spectra (MS) were recorded with a LCQ Finnigan MAT spectrometer and the masses are reported in Dalton. High Resolution Mass Spectrometry analyses (HRMS) were carried out at the University College London.
Procedures for the [4+2] cycloadditions
The protocol and spectroscopic characterization of cycloadduct 3a was described in reference 20.
See the Supporting information (compounds 3b and 11).
See the Supporting information (compounds 9a, 9b and 12).
Typical procedure for the syn-dihydroxylation
A solution of cycloadduct 3 or 11 (1.00 equiv) in acetone (0.5 mL/0.1 mmol) was treated successively by water (0.3 mL/0.1 mmol), NMO (2.60 equiv) and K2OsO4∙2H2O (0.02 equiv) at room temperature. After 24 h, toluene was added and the solution was concentrated under reduced pressure. The resulting dark oil was directly purified by column chromatography on silica gel.
See the Supporting information (compounds 5a, 5b and 13).
Typical procedures for the diethyl phosphonate deprotection
A solution of 3 or 5 (1 equiv), trimethylsilyl bromide (3,1 equiv) and anhydrous CH2Cl2 (0.6 mL/0.1 mmol) was stirred at room temperature under atmosphere of dry argon during for 4 days. The ethyl bromide by-product, the excess of trimethylsilyl bromide and the solvent were evaporated under reduced pressure. The reaction mixture was then hydrolyzed with an excess of MeOH (1.6 mL/0.1 mmol) at room temperature during 1h. The solvent was evaporated under reduced pressure.
See the Supporting information (compounds 4a, 4b and 6a).
ESI-HRMS
ESI-HRMS analyses were performed with a LTQ-Orbitrap XL hybrid mass spectrometer (Thermo Fisher Scientific, Bremen, Germany). Data were acquired in the positive ion mode, using a fullscan MS with a mass range of 200–2000 m/z. The orbitrap operated at 30,000 resolution [FWHM (full width at half maximum) definition]. All experimental data were acquired, along with a daily external calibration prior to the data acquisition. The appropriate tuning of the electrospray ion source was done to ensure the preservation of the complexes formed in the solution, but to avoid the detection of adducts formed during the ESI process. The following electrospray inlet conditions were applied: flow rate, 200 μLmin–1; spray voltage, 5 kV; sheath gas (N2) flow rate, 20 a.u.; auxiliary gas (N2) flow rate, 20 a.u.; capillary temperature, 275 °C; capillary voltage, 45 V; and tube lens, 80 V. The salts, which were purchased from Aldrich, Alpha, or Acros, were of the highest quality available and vacuum dried at room temperature over P2O5 prior to use. Ethanol from Fisher (absolute) was used for the MS experiments. For all experiments, the concentration of the ligand was approximately 0.5 mM with 5 equiv. of cation.
Supplementary Information
Included are characterization of new compounds of compounds 3b, 4a, 4b, 5a, 5b, 6a, 9a, 9b, 11, 12 and 13, spectroscopic analysis of compounds 4a, 6a, 5b, 9a, 9b, and 13, the mass spectra of complexes and tables of exact masses.
References
1. (a) M. Bols, R. G. Hazell, and I. B. Thomsen, Chem. Eur. J., 1997, 3, 940; CrossRef (b) H. H. Jensen, A. Jensen, R. G. Hazell, and M. Bols, J. Chem. Soc., Perkin Trans. 1, 2002, 1190. CrossRef
2. M. Kaname, M. Yamada, S. Yoshifuji, and H. Sashida, Chem. Pharm. Bull., 2009, 57, 49. CrossRef
3. (a) K. Bevan, J. S. Davies, C. H. Hassall, R. B. Morton, and D. A. S. Phillips, J. Chem. Soc., C, 1971, 514; CrossRef (b) K. J. Hale, S. Manaviazar, J. H. George, M. A. Walters, and S. M. Dalby, Org. Lett., 2009, 11, 733; CrossRef (c) K. Umezawa, Y. Ikeda, Y. Uchihata, H. Naganawa, and S. Kondo, J. Org. Chem., 2000, 65, 459; CrossRef (d) W. Li, J. Gan, and D. Ma, Org. Lett., 2009, 11, 5694. CrossRef
4. (a) S. R. LaBrenz, H. Bekele, and J. W. Kelly, Tetrahedron, 1998, 54, 8671; CrossRef (b) M.Bayrakcı, S. Ertul, and M. Yilmaz, J. Chem. Eng. Data, 2012, 57, 233. CrossRef
5. P. J. Diel and L. Maier, Patent Eur. Pat. Appl. EP143078 (1985) (Chem. Abstr., 1985, 103, 15544m).
6. (a) M. Kaname, K. Yoshinaga, Y. Arakawa, and S. Yoshifuji, Chem. Pharm. Bull., 2004, 52, 160; CrossRef (b) M. Kaname, K. Yoshinaga, Y. Arakawa, and S. Yoshifuji, Tetrahedron Lett., 1999, 40, 7993. CrossRef
7. J.-C. Monbaliu and J. Marchand-Brynaert, Synthesis, 2009, 11, 1876. CrossRef
8. (a) H. H. Wasserman, H. Matsuyama, and R. P. Robinson, Tetrahedron, 2002, 58, 7177; CrossRef (b) H. Matsuyama, A. Kurosawa, T. Takei, N. Ohira, M. Yoshida, and M. Iyoda, Chem. Lett., 2000, 1104. CrossRef
9. (a) I. K. Korobitsyna, A. V. Khalikova, L. L. Rodina, and N. P. Shusherina, Chem. Heterocycl. Compd., 1983, 117; CrossRef (b) R. C. Cookson, S. S. H. Giliani, and I. D. R. Stevens, J. Chem. Soc., C, 1967, 1905. CrossRef
10. (a) M. Chiara Aversa, A. Barattucci, P. Bonaccorsi, F. Caruso, and P. Giannetto, ARKIVOC, 2004, i, 79; (b) A. Wasilewska, F. Sączewski, M. Gdaniec, A. Makowska, and P. J. Bednarski, ARKIVOC, 2011, x, 160; (c) U. Pindur, Heterocycles, 1988, 27, 1253; CrossRef (d) F. Palacios, C. Alonso, G. Rubiales, C. Tobillas, and J. M. Ezpeleta, Heterocycles, 2003, 61, 493; CrossRef (e) F. Jensen and C. S. Foote, J. Am. Chem. Soc., 1987, 109, 6376; CrossRef (f) B. T. Gillis and J. D. Hagarty, J. Org. Chem., 1967, 32, 330. CrossRef
11. J.-C. Monbaliu, E. Villemin, B. Elias, and J. Marchand-Brynaert, Targets in Heterocyclic Systems (THS), Reviews and Accounts on Heterocylic Chemistry (editors: O. A. Attanasi and D. Spinelli, Chemical Italian Society, Division of Medicinal Chimistry), 2010, 14, 49.
12. B. F. Tawil, A. Guggisberg, and M. Hesse, Tetrahedron, 1992, 48, 3775. CrossRef
13. (a) M. Sato, M. Kahn, Z. L. Lin, M. E. Johnson, and T. K. Hayes, Bioorg. Med. Chem. Lett., 1993, 13, 1277; CrossRef (b) H. Matsuyama, N. Itoh, A. Matsumoto, N. Ohira, K. Hara, M. Yoshida, and M. Iyoda, J. Chem. Soc., Perkin Trans. 1, 2001, 2924. CrossRef
14. C. Bournaud, F. Chung, A. Pérez Luna, M. Pasco, G. Errasti, T. Lecourt, and L. Micouin, Synthesis, 2009, 6, 869. CrossRef
15. C. L. Molina, C. P. Chow, and K. J. Shea, J. Org. Chem., 2007, 72, 6816. CrossRef
16. (a) A. Windaus and W. Grundmann, Ann., 1936, 524, 295; (b) H. H. Inhoffen, G. Quinkert, S. Schitz, D. Kampe, and D. F. Domagk, Chem. Ber., 1957, 90, 664. CrossRef
17. C. D. Mayer and F. Bracher, Eur. J. Med. Chem., 2011, 46, 3227. CrossRef
18. S. A. F. Elias, S. K. U. Kunimon Funhimon, S. S. More, E. Tovar, V. H. Dahanukar, S. B. Meruva, R. Akula, A. K. Nuka, J. Puppalla, and S. Gudia, Dr. Reddy’s Laboratories Ltd., 2010, Patent EP2221299 A2, 2010, CAN 153:334058.
19. V. V. Kane and S. D. Levine, Patent US 4250180, 1981, CAN 94:208893.
20. E. Villemin, B. Elias, R. Robiette, K. Robeyns, M.-F. Herent, J.-L. Habib, and J. Marchand-Bryanert, Tetrahedron Lett., 2011, 52, 5140. CrossRef
21. (a) R. Reichenback-Klinke and B. König, J. Chem. Soc., Dalton Trans., 2002, 121; CrossRef (b) R. M. Izatt, R. L. Bruening, B. J. Tarbet, L. D. Griffin, M. L. Bruening, K. E. Krakowiak, and J. S. Bradshaw Pure Appl. Chem., 1990, 62, 1115. CrossRef
22. M. José Alves, F. T. Costa, V. C. M. Duarte, A. Gil Fortes, J. A. Martins, and N. M. Micaelo, J. Org. Chem., 2011, 76, 9584. CrossRef
23. C. E. Mc Kenna, M. T. Higa, N. H. Cheung, and M.-C. McKenna, Tetrahedron Lett., 1977, 18, 155. CrossRef
24. (a) M. J. Burk and J. E. Feaster, J. Am. Chem. Soc., 1992, 114, 6266; CrossRef (b) H. Ding and G. K. Friestad, Org. Lett., 2004, 6, 637. CrossRef
25. S. Lebrun, A. Couture, E. Deniau, and P. Grandclaudon, Synlett, 2009, 16, 2621. CrossRef
26. (a) H. Stetter and H. Spangenberger, Chem. Ber., 1958, 91, 1982; CrossRef (b) H. Stetter and K. Findeisen, Chem. Ber., 1965, 98, 3228. CrossRef
27. R. W. Alder, P. Eastment, R. E Moss, R. B Sessions, and M. A. Stringfellow, Tetrahedron Lett., 1982, 23, 4181. CrossRef
28. S. Grabowski, J. Armbruster, and H. Prinzbach, Tetrahedron Lett., 1997, 38, 5485. CrossRef
29. (a) R. A. Clement, J. Org. Chem., 1960, 25, 1724; CrossRef (b) R. A. Clement, J. Org. Chem., 1962, 27, 1115. CrossRef
30. R. Cookson, S. Gilani, and J. Stevens, Tetrahedron Lett., 1962, 14, 615. CrossRef