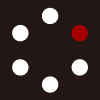
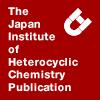
HETEROCYCLES
An International Journal for Reviews and Communications in Heterocyclic ChemistryWeb Edition ISSN: 1881-0942
Published online by The Japan Institute of Heterocyclic Chemistry
e-Journal
Full Text HTML
Received, 6th October, 2014, Accepted, 23rd March, 2015, Published online, 10th April, 2015.
■ Recent Progress in The Synthetic Study of an Antitumor Marine Macrolide Aplyronine A and Related Molecules
Ichiro Hayakawa* and Hideo Kigoshi*
Department of Chemistry, Graduate School of Pure and Applied Sciences, University of Tsukuba, 1-1-1 Ten-nodai, Tsukuba-shi, Ibaraki 305-8571, Japan
Abstract
Aplyronine A is a potent antitumor marine macrolide. The unique molecular structures of aplyronine A and its congeners, in conjunction with their potent biological activities, have made them attractive synthetic targets. This review discusses recent progress in the development of efficient routes to the synthesis of aplyronines and the related molecules.CONTENTS
1. Introduction
2. Total syntheses of aplyronines
2.1. Yamada–Kigoshi’s synthesis of aplyronines A–C
2.2. Kigoshi’s asymmetric Ni/Cr-mediated coupling approach for the second-generation synthesis of aplyronine A
2.3. Paterson’s synthesis of aplyronine C
3. Synthetic approaches toward aplyronines and the related molecules
3.1. Fuchs’s ring-opening reaction of chiral cyclic vinyl sulfones approach
3.2. Kigoshi’s synthesis of an aplyronine A–mycalolide B hybrid molecule
4. Conclusion
1. INTRODUCTION
The search for the seeds of novel types of drugs, such as antitumor agents, from marine animals and plants is ongoing all over the world.1 For instance, Hirata and Uemura discovered halichondrin B, an antitumor polyether macrolide from a marine sponge, in 1985 (Figure 1).2 In 2011, Eisai Co., Ltd., released Halaven® (eribulin mesylate) as a novel anticancer agent against breast cancer, which was inspired from halichondrin B through joint development by Eisai Research Institute and the Kishi group.3 In this way, the development of novel types of drugs based on marine natural products is an important research field.
CONTENTS
1. Introduction
2. Total syntheses of aplyronines
2.1. Yamada–Kigoshi’s synthesis of aplyronines A–C
2.2. Kigoshi’s asymmetric Ni/Cr-mediated coupling approach for the second-generation synthesis of aplyronine A
2.3. Paterson’s synthesis of aplyronine C
3. Synthetic approaches toward aplyronines and the related molecules
3.1. Fuchs’s ring-opening reaction of chiral cyclic vinyl sulfones approach
3.2. Kigoshi’s synthesis of an aplyronine A–mycalolide B hybrid molecule
4. Conclusion
1. INTRODUCTION
The search for the seeds of novel types of drugs, such as antitumor agents, from marine animals and plants is ongoing all over the world.1 For instance, Hirata and Uemura discovered halichondrin B, an antitumor polyether macrolide from a marine sponge, in 1985 (Figure 1).2 In 2011, Eisai Co., Ltd., released Halaven® (eribulin mesylate) as a novel anticancer agent against breast cancer, which was inspired from halichondrin B through joint development by Eisai Research Institute and the Kishi group.3 In this way, the development of novel types of drugs based on marine natural products is an important research field.
The unique molecular structures of aplyronine A (1) and its congeners, in conjunction with their potent biological activities, have made them attractive synthetic targets. Several groups have reported approaches to synthesize aplyronines. In 2009, we reviewed the total synthesis of aplyronine A (1) and the synthetic study of aplyronines.4 In the present review, we outline our synthetic study of aplyronines and introduce synthetic works since 2009.
2. TOTAL SYNTHESIS OF APLYRONINES
2.1. Yamada–Kigoshi’s synthesis of aplyronines A–C
In 1994 Yamada, Kigoshi, and co-workers reported the first total synthesis of aplyronine A (1).7 This synthesis confirmed the absolute configuration of aplyronine A (1) and determined the absolute configurations of aplyronines B (2) and C (3). Features of this synthesis were 1) construction of four contiguous stereogenic centers by using the Evans aldol reaction12 and the Sharpless asymmetric epoxidation,13 and 2) the coupling reaction of various segments by using the Julia olefination14 as key steps (Figure 3).
The unique molecular structures of aplyronine A (1) and its congeners, in conjunction with their potent biological activities, have made them attractive synthetic targets. Several groups have reported approaches to synthesize aplyronines. In 2009, we reviewed the total synthesis of aplyronine A (1) and the synthetic study of aplyronines.4 In the present review, we outline our synthetic study of aplyronines and introduce synthetic works since 2009.
2. TOTAL SYNTHESIS OF APLYRONINES
2.1. Yamada–Kigoshi’s synthesis of aplyronines A–C
In 1994 Yamada, Kigoshi, and co-workers reported the first total synthesis of aplyronine A (1).7 This synthesis confirmed the absolute configuration of aplyronine A (1) and determined the absolute configurations of aplyronines B (2) and C (3). Features of this synthesis were 1) construction of four contiguous stereogenic centers by using the Evans aldol reaction12 and the Sharpless asymmetric epoxidation,13 and 2) the coupling reaction of various segments by using the Julia olefination14 as key steps (Figure 3).
Coupling reaction of the C21–C27 and C28–C34 segments 12 and 16 by Julia olefination14 afforded olefin 22 in good yield (Scheme 2). Olefin 22 was converted into the C21–C34 segment 23 in five steps. The DMBOM [{(3,4-dimethoxybenzyl)oxy}methyl] ether group15 could be removed under mild conditions at the end game of the total synthesis.7
On the other hand, Julia olefination14 between the C5–C14 segment 21 and the C15–C20 segment 24 gave the desired E-olefin 25 in 44% yield (Scheme 3). The undesired Z-olefin 26 (19%) and tertiary alcohol 27 (25%) were obtained in this coupling conditions. The desired E-olefin 25 was transformed into aldehyde 28 as the C5–C20 segment in four steps.
The Julia olefination14 between the C5–C20 segment 28 and the C21–C34 segment 23 gave a hydroxy sulfone, which was subjected to acetylation and reduction to furnish the coupling compound 29 (Scheme 4). Compound 29 was converted in five steps into seco acid 30, which has two hydroxy groups at C23 and C25 as possible reaction sites. Macrolactonization under the modified Yamaguchi conditions16,17 was carried out to afford the desired 24-membered lactone 31 and the undesired 26-membered lactone 32 in a ratio of ca. 4 : 3. The undesired 26-membered lactone 32 was isomerized to the desired 24-membered lactone 31 by using Ti(Oi-Pr)4.18
The desired lactone 31 was a common intermediate of aplyronines. Thus, the introduction of the N-methylformamide group at C34, the N,N-dimethylalanine ester group at C29, and the N,N,O-trimethylserine ester group (at C7 for aplyronine A (1), at C9 for aplyronine B (2)) afforded aplyronines A (1), B (2), and C (3) (Scheme 5).7 This total synthesis of aplyronine A (1) yielded an amount sufficient for further biological studies, including animal experiments.
2.2. Kigoshi’s asymmetric Ni/Cr-mediated coupling approach for the second-generation synthesis of aplyronine A
In section 2.1, we introduced the first total synthesis of aplyronines by Yamada and Kigoshi.7 However, this synthetic strategy had a problem. Thus, Julia olefination14 to construct a C14 trisubstituted double bond showed poor stereoselectivity: the desired (E)-olefin (44%), the undesired (Z)-olefin (19%), and the C14 tertiary alcohol (25%). Therefore, Kigoshi planned to improve the stereoselective construction of the C14–C15 (E)-trisubstituted double bond by using an asymmetric Ni/Cr-mediated coupling (Nozaki–Hiyama–Takai–Kishi coupling)19 as a key step for the second-generation total synthesis of aplyronine A (1) and the synthesis of related artificial analogues (Scheme 6). This strategy has the benefit of constructing the C14–C15 (E)-trisubstituted double bond and the C13 stereogenic center at once.
The C5–C13 segment 38 was prepared from synthetic intermediate 357 in the first-generation total synthesis of aplyronine A (1) in seven steps (Scheme 7). Also, the C14–C19 segment 40 was synthesized from 3920 by using regioselective hydrozirconation with Cp2ZrHCl.21
The C5–C13 segment 38 was prepared from synthetic intermediate 357 in the first-generation total synthesis of aplyronine A (1) in seven steps (Scheme 7). Also, the C14–C19 segment 40 was synthesized from 3920 by using regioselective hydrozirconation with Cp2ZrHCl.21
Coupling compound 42 was converted into the C1–C19 segment 44 by the Kigoshi group’s use of their first-generation synthetic strategy (Scheme 9). The group employed this strategy in the synthesis of aplyronine A–mycalolide B hybrid molecule 76 (see Section 3.2).22
2.3 Paterson’s synthesis of aplyronine C
Paterson and co-workers achieved the total synthesis of aplyronine C (3) in 2013.23 Their strategy involved a key fragment coupling between the C1–C27 segment 53 and the C28–C34 segment 51 using a boron-mediated aldol coupling reaction (Figure 4).24
The C28–C34 segment 51 was prepared with the Mukaiyama Sn(II)-promoted aldol reaction25 as a key step (Scheme 10). Thus, the treatment of ketone 45 and acetaldehyde with Sn(OTf)2 gave syn aldol adduct 46. 1,3-Anti-selective reduction of aldol adduct 46 under the Evans–Tishchenko conditions26 and regioselective acylation afforded ester 47. Ester 47 was converted into aldehyde 48 in four steps. Introduction of N-methylformamide group to 48 gave (Z)-vinyl formamide 50, which was isomerized into the desired (E)-vinyl formamide 51 as the C28–C34 segment. The C1–C27 segment 53 was prepared from Paterson’s previous synthetic intermediate 52 in four steps.27 Aldol coupling reaction between the C1–C27 segment 53 and the C28–C34 segment 51 with c-Hex2BCl/Et3N furnished β-hydroxy ketone 54 as a diastereomeric mixture of alcohols at C27.24 Deoxygenation of the C27 hydroxy group and stereoselective reduction of ketone at C29 with ZnBH4 gave compound 55. To convert compound 55 into aplyronine C (3), they followed Yamada–Kigoshi’s procedure7 to achieve the total synthesis of aplyronine C (3).
3. SYNTHETIC APPROACHES TOWARD APLYRONINES AND THE RELATED MOLECULES
3.1. Fuchs’s ring-opening reaction of chiral cyclic vinyl sulfones approach
In 2011, Fuchs and co-workers reported the synthesis of the C1–C20 and C15–C27 segments 68 and 75 of aplyronine A (1).28 They previously established the stereoselective synthesis of vinyl sulfone 56 as a starting material of the C15–C20 part (Figure 5).29 They also developed a stereoselective method of synthesizing seven-membered vinyl sulfones 59 and 69 involving four contiguous stereogenic centers by using the double Lawton SN2’ addition as a key step.30 These vinyl sulfones 59 and 69 were used as precursors of C5–C11 and C21–C27 segments 62 and 73, respectively.
Synthesis of the C15–C20 segment 58 is shown in Scheme 11. The vinyl sulfone 5629 was converted into aldehyde 58 as the C15–C20 segment in four steps. Synthesis of the C1–C20 segment 68 started from seven-membered vinyl sulfone 5930 (Scheme 11). Thus, oxidation of vinyl sulfone 59 by ozone followed by reduction with BH3·t-BuNH2 gave lactone 60. Protection of the primary hydroxy group of lactone 60 and the subsequent ring-opening reaction afforded amide 61. Reduction of the dimethyl amide group in 61 and iodination of the resulting hydroxy group gave iodide 62. To convert iodide 62 into the C1–C20 segment 68, the Fuchs group followed Paterson’s27 and Yamada–Kigoshi’s7 synthetic strategies for aplyronines.
On the other hand, they synthesized the C15–C27 segment 75 by using Julia–Kocienski olefination31 as a key step (Scheme 12). Thus, they followed Yamada–Kigoshi’s synthetic strategy7 with modification. The seven-membered vinyl sulfone 69,30 which was a diastereomer of vinyl sulfone 59, was converted into lactone 70. Introduction of a thio tetrazole group at the hydroxy group of lactone 70 by Mitsunobu reaction32 and the subsequent ring-opening reaction afforded Weinreb amide 72. This amide was transformed into sulfone 73 as a precursor of Julia–Kocienski olefination.31 Treatment of sulfone 73 with LHMDS in DMF/HMPA followed by the addition of aldehyde 57 furnished coupling compound 74 in 60% yield (83% based on recovered starting material), which was converted into aldehyde 75 as the C15–C27 segment.
3.2. Kigoshi’s synthesis of an aplyronine A–mycalolide B hybrid molecule
The hybrid molecule consisting of the macrolactone part of aplyronine A (1) and the side-chain part of mycalolide B (77)33 was designed by Kigoshi and co-workers (Figure 6). Hybrid molecule 76 is expected to possess stronger actin-depolymerizing activity and cytotoxicity than aplyronine A for three reasons. First, the side-chain moiety in aplyronine A is crucial for both cytotoxicity and actin-depolymerizing activity.9 Second, the synthetic artificial analogue consisting of only the side-chain part of mycalolide B exhibits stronger actin-depolymerizing activity than that of aplyronine A.34 Third, the macrolactone part of aplyronine A significantly emphasizes its cytotoxicity.9 Thus, they synthesized aplyronine A–mycalolide B hybrid molecule 76 to evaluate its biological activity.
The aplyronine A–mycalolide B hybrid molecule 76 was synthesized by a strategy similar to that for the second-generation synthesis of aplyronine A (1) (Section 2.2). They attempted the synthesis of the C20–C34 segment 8122 by an asymmetric Ni/Cr-mediated coupling19 between the C21–C28 and the C29–C34 segments 78 and 79 (Scheme 13). The asymmetric Ni/Cr-mediated coupling of 78 and 79 with our modified chiral sulfonamide ligand 4120 also worked successfully to afford the best yield and stereoselectivity (desired 80 : undesired stereoisomer = 17.6 : 1) among several known sulfonamide ligands. The coupling compound 80 was converted into an aldehyde by a modification of Yamada–Kigoshi’s synthetic method,7 which was subjected to Takai olefination35 and removal of the TBS group to furnish the C20–C34 segment 81.
The C1–C19 segment 4420 and the C20–C34 segment 8122 were connected by using Yamaguchi esterification16 to afford ester 82, which was transformed into aldehyde 83 as a precursor of Ni/Cr-mediated macrocyclization (Scheme 14).36 The Ni/Cr-mediated macrocyclization proceeded smoothly to give 84 (46%) and 85 (49%) even at a higher concentration (c = 10 mM)37 than that of the modified Yamaguchi macrolactonization16,17 in the Yamada–Kigoshi’s total synthesis of aplyronine A (c = 0.39 mM). The undesired C19 epimer 85 could be transformed into the desired isomer 84 by using a sequence of Dess–Martin oxidation and CBS reduction.38
Compound 84 was transformed into hybrid molecule 76 following their first-generation total synthesis of aplyronine A (1)7 and the synthesis of a side-chain analogue of mycalolide B34 (Scheme 15). Thus, they introduced the N-methylformamide group at C34, the O,O-dimethylglyceric ester group at C29, and the N,N,O-trimethylserine ester groups at C7 to furnish aplyronine A–mycalolide B hybrid molecule 76.22
The hybrid molecule 76 showed more potent actin-depolymerizing activity (EC50 = 1.0 μM) than that of aplyronine A (1) (EC50 = 1.4 μM). On the other hand, the cytotoxicity of hybrid molecule 76 (IC50 = 12 nM) proved to be about 1000-fold weaker than that of aplyronine A (1) (IC50 = 0.010 nM).22 These results indicate that there is no direct correlation between actin-depolymerizing activity and cell-growth inhibitory activity, and they are consistent with the finding of tubulin as the second target protein.8–10,34
4. CONCLUSION
In this review, we discussed the development of efficient synthetic routes of aplyronines and related compounds to enable further biological evaluations of these compounds. Because aplyronine A shows potent antitumor activity and a unique mechanism of action, it and its related compounds may serve as lead compounds for novel types of anti-cancer chemotherapeutic agents. The development of a more effective method of synthesizing aplyronine A is anticipated.
ACKNOWLEDGEMENTS
We are grateful for the financial supports from the MEXT/JSPS, the Uehara Memorial Foundation (H. K.), the Suntory Institute for Bioorganic Research (I. H.), the Meiji Seika (I. H.), and the Naito Foundation (I. H.).
References
1. T. F. Molinski, D. S. Dalisay, S. L. Lievens, and J. P. Saludes, Nat. Rev. Drug Discov., 2009, 8, 69. CrossRef
2. D. Uemura, K. Takahashi, T. Yamamoto, C. Katayama, J. Tanaka, Y. Okumura, and Y. Hirata, J. Am. Chem. Soc., 1985, 107, 4796. CrossRef
3. Review: T. K. Huyck, W. Gradishar, F. Manuguid, and P. Kirkpatrick, Nat. Rev. Drug Discov., 2011, 10, 173; CrossRef Structure–activity relationship study of halichondrin B: W. Zheng, B. M. Seletsky, M. H. Palme, P. J. Lydon, L. A. Singer, C. E. Chase, C. A. Lemelin, Y. Shen, H. Davis, L. Tremblay, M. J. Towle, K. A. Salvato, B. F. Wels, K. K. Aalfs, Y. Kishi, B. A. Littlefield, and M. J. Yu, Bioorg. Med. Chem. Lett., 2004, 14, 5551; CrossRef Synthesis of Halaven®: D-S. Kim, C-G. Dong, J. T. Kim, H. Guo, J. Huang, P. S. Tiseni, and Y. Kishi, J. Am. Chem. Soc., 2009, 131, 15636; CrossRef C-G. Dong, J. A. Henderson, Y. Kaburagi, T. Sasaki, D-S. Kim, J. T. Kim, D. Urabe, H. Guo, and Y. Kishi, J. Am. Chem. Soc., 2009, 131, 15642. CrossRef
4. Review: K. Yamada, M. Ojika, H. Kigoshi, and K. Suenaga, Nat. Prod. Rep., 2009, 26, 27. CrossRef
5. K. Yamada, M. Ojika, T. Ishigaki, Y. Yoshida, H. Ekimoto, and M. Arakawa, J. Am. Chem. Soc., 1993, 115, 11020; CrossRef M. Ojika, H. Kigoshi, T. Ishigaki, and K. Yamada, Tetrahedron Lett., 1993, 34, 8501; CrossRef M. Ojika, H. Kigoshi, T. Ishigaki, M. Nisiwaki, I. Tsukada, K. Mizuta, and K. Yamada, Tetrahedron Lett., 1993, 34, 8505; CrossRef M. Ojika, H. Kigoshi, T. Ishigaki, I. Tsukada, T. Tsuboi, T. Ogawa, and K. Yamada, J. Am. Chem. Soc., 1994, 116, 7441; CrossRef M. Ojika, H. Kigoshi, Y. Yoshida, T. Ishigaki, M. Nisiwaki, I. Tsukada, M. Arakawa, H. Ekimoto, and K. Yamada, Tetrahedron, 2007, 63, 3138. CrossRef
6. M. Ojika, H. Kigoshi, K. Suenaga, Y. Imamura, K. Yoshikawa, T. Ishigaki, A. Sakakura, T. Mutou, and K. Yamada, Tetrahedron, 2012, 68, 982. CrossRef
7. H. Kigoshi, M. Ojika, K. Suenaga, T. Mutou, J. Hirano, A. Sakakura, T. Ogawa, M. Nisiwaki, and K. Yamada, Tetrahedron Lett., 1994, 35, 1247; CrossRef H. Kigoshi, M. Ojika, T. Ishigaki, K. Suenaga, T. Mutou, A. Sakakura, T. Ogawa, and K. Yamada, J. Am. Chem. Soc., 1994, 116, 7443; CrossRef K. Suenaga, T. Ishigaki, A. Sakakura, H. Kigoshi, and K. Yamada, Tetrahedron Lett., 1995, 36, 5053. CrossRef
8. Review: M. Kita and H. Kigoshi, Nat. Prod. Rep., 2015, 32, in press. doi:10.1039/c4np00129j; CrossRef S. Saito, S. Watabe, H. Ozaki, H. Kigoshi, K. Yamada, N. Fusetani, and H. Karaki, J. Biochem., 1996, 120, 552; CrossRef T. Kuroda, K. Suenaga, A. Sakakura, T. Handa, K. Okamoto, and H. Kigoshi, Bioconjugate Chem., 2006, 17, 524; CrossRef K. Hirata, S. Muraoka, K. Suenaga, T. Kuroda, K. Kato, H. Tanaka, M. Yamamoto, M. Takata, K. Yamada, and H. Kigoshi, J. Mol. Biol., 2006, 356, 945; CrossRef M. Kita, Y. Hirayama, M. Sugiyama, and H. Kigoshi, Angew. Chem. Int. Ed., 2011, 50, 9871; CrossRef M. Kita, K. Yoneda, Y. Hirayama, K. Yamagishi, Y. Saito, Y. Sugiyama, Y. Miwa, O. Ohno, M. Morita, K. Suenaga, and H. Kigoshi, ChemBioChem, 2012, 13, 1754; CrossRef M. Kita, Y. Hirayama, K. Yamagishi, K. Yoneda, R. Fujisawa, and H. Kigoshi, J. Am. Chem. Soc., 2012, 134, 20314; CrossRef O. Ohno, M. Morita, K. Kitamura, T. Teruya, K. Yoneda, M. Kita, H. Kigoshi, and K. Suenaga, Bioorg. Med. Chem. Lett., 2013, 23, 1467. CrossRef
9. H. Kigoshi, K. Suenaga, T. Mutou, T. Ishigaki, T. Atsumi, H. Ishiwata, A. Sakakura, T. Ogawa, M. Ojika, and K. Yamada, J. Org. Chem., 1996, 61, 5326; CrossRef K. Suenaga, N. Kamei, Y. Okugawa, M. Takagi, A. Akao, H. Kigoshi, and K. Yamada, Bioorg. Med. Chem. Lett., 1997, 7, 269; CrossRef H. Kigoshi, K. Suenaga, M. Takagi, A. Akao, K. Kanematsu, N. Kamei, Y. Okugawa, and K. Yamada, Tetrahedron, 2002, 58, 1075. CrossRef
10. M. Kita, Y. Hirayama, K. Yoneda, K. Yamagishi, T. Chinen, T. Usui, E. Sumiya, M. Uesugi, and H. Kigoshi, J. Am. Chem. Soc., 2013, 135, 18089. CrossRef
11. C. Dumontet and M. A. Jordan, Nat. Rev. Drug Discov., 2010, 9, 790. CrossRef
12. D. A. Evans, J. Bartroli, and T. L. Shih, J. Am. Chem. Soc., 1981, 103, 2127. CrossRef
13. T. Katsuki and K. B. Sharpless, J. Am. Chem. Soc., 1980, 102, 5974. CrossRef
14. M. Julia and J.-M. Paris, Tetrahedron Lett., 1973, 14, 4833. CrossRef
15. W.-H. Gündel and W. Kramer, Chem. Ber., 1978, 111, 2594. CrossRef
16. J. Inanaga, K. Hirata, H. Saeki, T. Katsuki, and M. Yamaguchi, Bull. Chem. Soc. Jpn., 1979, 52, 1989. CrossRef
17. M. Hikota, Y. Sakurai, K. Horita, and O. Yonemitsu, Tetrahedron Lett., 1990, 31, 6367. CrossRef
18. D. Seebach, E. Hungerbühler, R. Naef, P. Schnurrenberger, B. Weidmann, and M. Zügger, Synthesis, 1982, 138. CrossRef
19. H. Guo, C.-G. Dong, D.-S. Kim, D. Urabe, J. Wang, J. T. Kim, X. Liu, T. Sasaki, and Y. Kishi, J. Am. Chem. Soc., 2009, 131, 15387; CrossRef X. Liu, J. A. Henderson, T. Sasaki, and Y. Kishi, J. Am. Chem. Soc., 2009, 131, 16678; CrossRef X. Liu, X. Li, Y. Chen, Y. Hu, and Y. Kishi, J. Am. Chem. Soc., 2012, 134, 6136. and references cited therein. CrossRef
20. K. Kobayashi, Y. Fujii, I. Hayakawa, and H. Kigoshi, Org. Lett., 2011, 13, 900. CrossRef
21. J. Schwartz and J. A. Labinger, Angew. Chem., Int. Ed. Engl., 1976, 15, 333. CrossRef
22. K. Kobayashi, Y. Fujii, Y. Hirayama, S. Kobayashi, I. Hayakawa, and H. Kigoshi, Org. Lett., 2012, 14, 1290. CrossRef
23. I. Paterson, S. J. Fink, L. Y. W. Lee, S. J. Atkinson, and S. B. Blakey, Org. Lett., 2013, 15, 3118. CrossRef
24. I. Paterson, K. Ashton, R. Britton, G. Cecere, G. Chouraqui, G. J. Florence, H. Knust, and J. Stafford, Chem. Asian J., 2008, 3, 367. CrossRef
25. T. Mukaiyama, R. W. Stevens, and N. Iwasawa, Chem. Lett., 1982, 11, 353. CrossRef
26. D. A. Evans and A. H. Hoveyda, J. Am. Chem. Soc., 1990, 112, 6447. CrossRef
27. I. Paterson, M. D. Woodrow, and C. J. Cowden, Tetrahedron Lett., 1998, 39, 6041. CrossRef
28. W. P. Hong, M. N. Noshi, A. El-Awa, and P. L. Fuchs, Org. Lett., 2011, 13, 6342. CrossRef
29. M. N. Noshi, A. El-Awa, E. Torres, and P. L. Fuchs, J. Am. Chem. Soc., 2007, 129, 11242. CrossRef
30. A. El-Awa and P. L. Fuchs, Org. Lett., 2006, 8, 2905. CrossRef
31. J. B. Baudin, G. Hareau, S. A. Julia, and O. Ruel, Tetrahedron Lett., 1991, 32, 1175; CrossRef P, R. Blakemore, W. J. Cole, P. J. Kocienski, and A. Morley, Synlett, 1998, 26.
32. O. Mitsunobu, Synthesis, 1981, 1. CrossRef
33. N. Fusetani, K. Yasumuro, S. Matsunaga, and K. Hashimoto, Tetrahedron Lett., 1989, 30, 2809; CrossRef S. Matsunaga, P. Liu, C. A. Celatka, J. S. Panek, and N. Fusetani, J. Am. Chem. Soc., 1999, 121, 5605. CrossRef
34. K. Suenaga, S. Miya, T. Kuroda, T. Handa, K. Kanematsu, A. Sakakura, and H. Kigoshi, Tetrahedron Lett., 2004, 45, 5383; CrossRef K. Suenaga, T. Kimura, T. Kuroda, K. Matsui, S. Miya, S. Kuribayashi, A. Sakakura, and H. Kigoshi, Tetrahedron, 2006, 62, 8278. CrossRef
35. K. Takai, K. Nitta, and K. Utimoto, J. Am. Chem. Soc., 1986, 108, 7408. CrossRef
36. H. Jin, J. Uenishi, W. J. Christ, and Y. Kishi, J. Am. Chem. Soc., 1986, 108, 5644; CrossRef K. Takai, M. Tagashira, T. Kuroda, K. Oshima, K. Utimoto, and H. Nozaki, J. Am. Chem. Soc., 1986, 108, 6048. CrossRef
37. Namba and Kishi reported a catalytic Ni/Cr-mediated macrocyclizaton without the use of high-dilution techniques: K. Namba and Y. Kishi, J. Am. Chem. Soc., 2005, 127, 15382. CrossRef
38. E. J. Corey, R. K. Bakshi, and S. Shibata, J. Am. Chem. Soc., 1987, 109, 5551 CrossRef