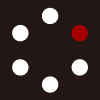
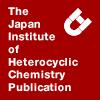
HETEROCYCLES
An International Journal for Reviews and Communications in Heterocyclic ChemistryWeb Edition ISSN: 1881-0942
Published online by The Japan Institute of Heterocyclic Chemistry
e-Journal
Full Text HTML
Received, 31st March, 2015, Accepted, 28th April, 2015, Published online, 14th May, 2015.
DOI: 10.3987/REV-15-820
■ Chemistry of 2,5-Diketopiperazine and Its Bis-Lactim Ether: A Brief Review
Shigeki Sano* and Michiyasu Nakao
Molecular Medicinal Chemistry, Graduate School of Pharmaceutical Sciences, The University of Tokushima, 1-78 Sho-machi, Tokushima 770-8505, Japan
Abstract
2,5-Diketopiperazines have attracted much attention because of their biological activities and molecular structure characteristics. Bis-lactim ethers derived from these 2,5-diketopiperazines include well-known organic molecules such as Schöllkopf chiral auxiliaries, which are used in asymmetric synthesis of α-amino acids. In this article, we briefly discuss the chemistry of both heterocyclic compounds.CONTENTS
1. Introduction
2. 2,5-Diketopiperazine
2-1. Synthesis of 2,5-diketopiperazine
2-2. Conformation of 2,5-diketopiperazine
2-3. Biological activities of 2,5-diketopiperazine
2-4. 2,5-Diketopiperazine as an organocatalyst
3. 3,6-Dialkoxy-2,5-dihydropyrazine
3-1. Synthesis of 3,6-dialkoxy-2,5-dihydropyrazine
3-2. Asymmetric alkylation of 3,6-dialkoxy-2,5-dihydropyrazine
3-3. Asymmetric aldol-type reaction of 3,6-dialkoxy-2,5-dihydropyrazine
4. Conclusion
1. INTRODUCTION
A number of cyclic peptides occur in natural products. Among them, the cyclic dipeptides known as 2,5-diketopiperazines 1 (2,5-DKPs, piperazine-2,5-diones) have recently attracted much attention from the standpoint of biological activities and molecular structure characteristics.1,2 As shown in Scheme 1, 2,5-DKP 1 is easily converted to the corresponding bis-lactim ether 2 (3,6-dialkoxy-2,5-dihydropyrazine) using Meerwein’s salt. The Schöllkopf chiral auxiliaries are a kind of bis-lactim ether 2 that can be flexibly applied to the asymmetric synthesis of α-amino acids.3 The present article provides a brief review of the chemistry of 2,5-DKP 1 and its bis-lactim ether 2, with a focus on their synthesis, reaction, and conformation.
2. 2,5-DIKETOPIPERAZINE
With respect to the chemical structures of diketopiperazine (DKP) molecules, three kinds of regioisomers are possible, 2,3-DKP A, 2,5-DKP B, and 2,6-DKP C, as shown in Scheme 2. Among these three heterocyclic compounds, 2,5-DKP B is perhaps the best known in the field of chemistry.
In 1936, Wrinch proposed a controversial model of protein structure, which was called “the cyclol hypothesis”.4 In this model, a peptide chain such as hexapeptide 3 could be converted by intramolecular cross-linking into the corresponding polycyclic derivative 4, which she called the “cyclol” structure (Scheme 3). The hypothesis proved to be invalid and theoretical calculations have recently confirmed its untenability from a thermodynamic as well as a kinetic point of view.5 But this well-regulated cyclol theory of protein based on 2,5-DKP structures merited particular attention. This was in part because 2,5-DKPs are ubiquitous organic compounds in our everyday lives, being present in various beverages and foods such as coffee,6 cocoa,7 beer,8 sake,9 bread,10 dried squid,11 beef,12 chicken,13 soy sauce,14 and miso paste.14
2-1. Synthesis of 2,5-diketopiperazine
The most extensively utilized strategy for the synthesis of 2,5-DKPs is intramolecular cyclization of the corresponding dipeptide precursors. In 1968, Nitecki and co-workers described intramolecular cyclization of dipeptide methyl esters 8 (R3 = Me) as a simple route to stereochemically pure 2,5-DKPs, as shown in Scheme 4.15 Condensation of N-protected α-amino acid 5 and α-amino acid ester 6 afforded N-protected dipeptides 7. Deprotection of the resulting N-protected dipeptide esters 7 followed by heating gave the corresponding 2,5-DKPs 1. In most situations, 2,5-DKP is insoluble in reaction solvents and it precipitates as it is formed. Thus stereochemically pure 2,5-DKP is easily obtained by a simple work-up, such as filtration and recrystallization of the crude product. However, isolated yields of 2,5-DKPs largely depend on their chemical structure coming from both amino acids as starting materials. Ordinary amino protecting groups such as benzyloxycarbonyl (Cbz),16 tert-butoxycarbonyl (Boc),17 and 9-fluorenylmethoxycarbonyl (Fmoc)18 are available in this synthetic strategy. However, the reaction conditions should be appropriately selected in order to avoid racemization.19 A recent example of 2,5-DKP synthesis includes chemo-enzymatic approach without protection of the amino group.20
Recently, microwave irradiation has been shown to be effective for a one-pot conversion of N-Boc-dipeptide esters 7 (PG = Boc) to 2,5-DKPs 1.21 The mechanistic details of the microwave-assisted deprotection of the N-Boc group are currently unclear, but it is known that a reaction solvent containing water must be utilized. 2,5-DKP is also known to be a troublesome by-product in solid-phase peptide synthesis. In other words, solid-phase synthesis was expected to be more suitable for the preparation of 2,5-DKPs than liquid phase synthesis, especially for the construction of a compound library.22 As a result, dipeptides attached to resins can be effectively converted to 2,5-DKPs by microwave irradiation.23
The Ugi four-component reaction has also afforded the acyclic peptide precursor 12 of N-substituted 2,5-DKP 13.24 Along with three components (aldehyde 9, primary amine 10, and isocyanide 11), N-protected α-amino acid 5 is used as a nucleophile in the Ugi reaction, as shown in Scheme 5. One-pot synthesis of 2,5-DKPs is also achieved using the Ugi reaction under microwave irradiation.25
2-2. Conformation of 2,5-diketopiperazine
2,5-DKPs play an important role in conformationally constrained peptidomimetics, and a 2,5-DKP has recently been reported to function as a reverse-turn inducer in β-hairpin peptidomimetics.26
With regard to conformation, 2,5-DKP rings basically adopt a flat structure in the crystalline state. For example, the planar conformation of the simplest 2,5-DKP composed of two molecules of glycine [cyclo(Gly-Gly)] was first revealed by Corey and co-workers by X-ray crystallographic analysis in 1938.27 The three-dimensional structure of N,N’-diacetyl-cyclo(Gly-Gly) determined by X-ray crystallography was subsequently reported to be a boat conformation.28 Recently, 2,5-DKPs composed of natural L-amino acids were shown to adopt a boat conformation by a quantum chemical study utilizing the DFT B3LYP method.29 As a result, the conformation of cyclo(Gly-Gly) in the crystalline state is somewhat influenced by crystal packing factors. In general, 2,5-DKP rings adopt a boat conformation, which is finely adjusted depending on the substituents at carbon and/or nitrogen atoms.
On the other hand, 2,5-DKPs bearing a benzyl moiety have been known to adopt a folded conformation, in which the benzyl moiety is folded over the 2,5-DKP ring.30 The folded conformation of these 2,5-DKPs in solution was suggested by the strong shielding effect of the benzyl group observed in 1H-NMR analyses. Based on the unambiguous spectroscopic effect, we proposed a plausible method (the DKP method) for determining the absolute configuration of α-substituted amino acids.31 For example, we developed several non-enzymatic and enzymatic asymmetric syntheses of α-substituted serines.32 Thus, a chiral α-substituted serine 14 of interest was transformed into both of the corresponding diastereomers of 2,5-DKPs 16 by utilizing L- and D-phenylalanine methyl ester hydrochloride [(S)- and (R)-15]. As a result, the absolute configuration of the original α-substituted serine 14 was determined by 1H-NMR analyses of the resulting diastereomers [(6S)- and (6R)-16] by the DKP method as shown in Scheme 6. If the 1H-NMR chemical shift of CH2 of the hydroxymethyl group of (6S)-16 appears at a higher magnetic field than the corresponding protons of (6R)-16 due to the shielding effect of the benzyl group, the absolute configuration of 14 is deduced to be S. In other words, the chemical shift difference in ppm (ΔδSR) of these CH2 protons of (6S)- and (6R)-16 derived from (S)-14 shows a large negative value. In this regard, the ΔδSR value is defined as δ(S) – δ(R), where δ(S) and δ(R) are the chemical shifts of the corresponding protons in (S)- and (R)-16, respectively.
In order to investigate the driving force of the folded conformation of 2,5-DKPs with aromatic side chains, we have synthesized 2,5-DKPs bearing a benzyl moiety with different para-substituents and have demonstrated that these 2,5-DKPs adopt a folded conformation by virtue of the characteristic chemical shift of the cis-substituents (cis to the benzyl moiety) in 1H-NMR spectroscopy.33 In the 1H-NMR spectra of (S)-17a-g, the chemical shifts of HA seem to depend on the nature of the para-substituents of the benzyl groups. In 2,5-DKPs (S)-17f and (S)-17g, which bear the electron-donating para-substituents -OH and -NH2, the chemical shifts of HA were observed at higher magnetic field than that of (S)-17a. In contrast, the chemical shifts of HA of the 2,5-DKPs (S)-17d and (S)-17e, which bear electron-withdrawing para-substituents -Cl and -NO2, were observed at lower magnetic field than that of (S)-17a, as shown in Scheme 7. In other words, the π electron density of a benzene ring of the benzyl moiety seems to correlate with the existence of a charge-transfer interaction (π to σ*C-H) in the 2,5-DKPs (S)-17a-g. In general, a charge-transfer interaction is considered to be an important factor in the CH-π interaction.34 Thus, the probable origin of the folded conformation was inferred to be CH-π interactions based on the observed electronic effects of para-substituents on the benzyl group. On the other hand, the folded conformation of 2,5-DKPs with alkyl side chains has also been predicted by X-ray crystallographic analyses of cyclo(L-Ser-L-Ser)35 and cyclo(L-Met-Gly).36
In general, intermolecular hydrogen bond formation between 2,5-DKP rings (C=O ··· H–N) is responsible for the low solubility of 2,5-DKP in water and various organic solvents.37 For example, atomic force microscopy (AFM) revealed that cyclo(Gly-Gly) had a mesh-like structure that was dependent on a hydrogen bonding-mediated assembly.38 The deconvoluted FT-IR spectra of the amide regions also suggested the presence of parallel and anti-parallel β-sheets conformations in the 2,5-DKP supramolecular structure. In order to overcome the lack of solubility, breaking the intermolecular hydrogen bonds by mono- or di-methylation of nitrogen of the 2,5-DKP ring is a simple and effective strategy.39 We synthesized N-methylated derivatives of various 2,5-DKPs derived from L-phenylalanine and observed an improvement of solubility compared to the precursor 2,5-DKPs. Intriguingly, we also found that 2,5-DKPs bearing a benzyl moiety exhibited a folded or an extended conformation depending on the position of N-methylation (N1 or N4) according to the analysis of 1H-NMR spectra and the single crystal X-ray crystallography.40 For example, the folded conformation was observed in the crystalline structure of N1-methylated DKP (3S,6S)-18a and the intramolecular distance between the benzene ring of the benzyl group and methine hydrogen of the isopropyl group was 2.3769Å. On the other hand, N4-methylated DKP (3S,6S)-18b was found to exist in an extended conformation, as shown in Scheme 8. These conformations were also suggested by the analysis of 1H-NMR spectra in CDCl3. With respect to the conformation of DKP rings, (3S,6S)-18a adopted a pseudo twist-boat conformation. In contrast, a more distorted conformation was found in the DKP ring of (3S,6S)-18b.
2-3. Biological activities of 2,5-diketopiperazine
Most of the natural products containing a 2,5-DKP structure are known to possess interesting pharmacological properties, such as anti-bacterial, anti-viral, anti-fungal, anti-tumor, phytotoxic, and siderophoric activities.41 In order to show the versatility of 2,5-DKP-class natural products as biologically active compounds, a few examples are given here (Scheme 9).
Spirotryprostatin A (19), which has been isolated as a secondary metabolite from the fermentation broth of Aspergillus fumigatus, has been shown to inhibit the progression of cells at the G2/M phase.42,43 The core structure of 19 is a 2,5-DKP unit consisting of two L-proline molecules. Verruculogen (20), first isolated from Penicillium verruculosum in 1972, is a potent inhibitor of calcium-activated potassium channels, as well as a cell cycle inhibitor.44 An eight-membered cyclic peroxide is a characteristic structure of 20. Brevicompanine B (21), isolated from Penicillium brevicompactum and Aspergillus janus, is known to show pronounced plant growth regulatory activity.45 These 2,5-DKPs 19-21 are classified as prenylated indole alkaloids, which are hybrid natural products from prenyl diphosphates and tryptophan or its precursors and widely distributed in filamentous fungi, especially in the genera Penicillium and Aspergillus of ascomycota.46 Phenylahistin (22), which has been isolated from the fermentation broth of Aspergillus ustus NSC-F038, is also a cell cycle inhibitor.47 The fungus produces a racemic mixture of phenylahistin, but it is interesting to note that the (R)-enantiomer of 22 has no anti-microtubule activity. Cairomycin B (23), a bicyclic peptide antibiotic containing L-lysine and L-aspartic acid moieties, was isolated from Streptomyces As-C-19 obtained from the soil of Cairo.48 Cairomycin B (23) is mainly active against Gram-positive bacteria. The antibiotic bicyclomycin (24) was isolated from the fungus, Streptomyces sapporoensis in 1972.49 Bicyclomycin (24) is active against certain Gram-negative bacteria and widely used as a growth-promoting antibiotic for chickens and pigs. Maculosin-1 (25) and maculosin-2 (26) were isolated from liquid cultures of Alternaria alternata and are known as host-specific phytotoxins to spotted knapweed.50 Thaxtomin A (27), produced by Streptomyces scabies, is also a potent phytotoxin and is known as a virulence factor in the common scab potato disease.51 The antibiotic gliotoxin (28) has a sulfur bridge on the 2,5-DKP ring, which is called an epipolythiodioxopiperazine (ETP) ring, and acts as an immunomodulating agent.52 Rhodotorulic acid (29) is a dihydroxamate siderophore isolated from Rhodotorula pilimanae, and the 2,5-DKP ring of 29 is assumed to be biosynthesized starting with L-ornithine.53,54 Siderophores are defined as iron-chelating compounds utilized by bacteria and fungi under iron-limiting conditions.55
We have been interested in the iron-chelating ability of rhodotorulic acid [(3S,6S)-29] and achieved facile syntheses of (3S,6S)-29 and its N,N’-dimethylated derivative (3S,6S)-35 using microwave-assisted cyclization of the corresponding dipeptide precursors as shown in Scheme 10.56 Removal of the Boc group followed by intramolecular cyclization of the dipeptide precursor (S,S)-32 under microwave irradiation with a single-mode microwave reactor (InitiatorTM 60; Biotage AB) at 170 °C in a mixed solvent of water/methanol furnished the 2,5-DKP (3S,6S)-33. Catalytic hydrogenolysis of (3S,6S)-33 and the N,N’-dimethylated product (3S,6S)-34 provided rhodotorulic acid [(3S,6S)-29] and its N,N’-dimethylated derivative (3S,6S)-35. In general, 2,5-DKP derivatives have poor solubility in various solvents due to their intermolecular hydrogen bonding through the amide moiety of the 2,5-DKP ring.37 Therefore, only a few solvents, including water and dimethylsulfoxide (DMSO), have been found to be capable of dissolving (3S,6S)-29. However, (3S,6S)-35 was found to be soluble in water, DMSO, methanol, ethanol, chloroform, ethyl acetate, and so on. This enhanced solubility is likely due to the disappearance of intermolecular hydrogen bonds as a result of N,N’-dimethylation.
On the other hand, 2,5-DKPs have been used as naturally occurring privileged structures for drug design in medicinal chemistry, and several candidate medicinal agents have been developed by this approach (Scheme 11). In order to develop potent oxytocin (OT) antagonists that are highly selective over the vasopressin receptors, a research group of GlaxoSmithKline has applied the 2,5-DKP structure as a template for a structure-based drug design.57,58 As a result, they developed retosiban (36) as a potential clinical candidate for preterm labor. Retosiban (36) has sub-nanomolar affinity for OT receptors, with more than 1,400-fold selectivity over the related vasopressin receptors. The spiro-2,5-DKP template has also been effectively used in the development of CCR5 antagonists, and aplaviroc (37) was found to be a promising anti-HIV agent for development as a clinical trial candidate by Ono Pharmaceutical.59 In order to generate a natural product-like compound collection, the fusion of 2,5-DKPs with other biologically relevant heterocyclic cores was recently investigated using the strategy of oxidative cleavage and cyclization of linear peptides.60
2-4. 2,5-Diketopiperazine as an organocatalyst
A certain type of organic compounds with low molecular weight are able to catalyze organic reactions, and MacMillan coined the term “organocatalyst” to describe these organic compound in 2000.61 However, organocatalysts have a long history, and we can find pioneering reports of two organocatalysts, O-acetylquinine (38)62 and L-proline (39)63 as shown in Scheme 12.64
Amino acids and short peptides form one of the major families of organocatalysts, and 2,5-DKPs are also included in this family.65 In 1981, Inoue and co-workers reported that asymmetric hydrocyanation of various aromatic aldehydes was efficiently catalyzed by 2,5-DKP (3S,6S)-40 derived from L-phenylalanine and L-histidine (Scheme 13).66 As a possible explanation for this reaction, Schoenebeck and Houk proposed a dual activation mechanism involving a dimer of (3S,6S)-40 as the catalytic species based on computational studies.67
An enantioselective Strecker reaction using 2,5-DKP (3S,6S)-41 as a catalyst was reported by Lipton and co-workers in 1996.68 Stereoselective addition of hydrogen cyanide to a variety of N-substituted imines was catalyzed by (3S,6S)-41 derived from L-phenylalanine and L-norarginine (Scheme 14).69
We have designed and synthesized N4-methylated 2,5-DKP (3S,6S)-42 as a novel organocatalyst and investigated its preliminary application to an asymmetric aldol reaction. As a result, a moderate enantioselectivity (44% ee) in anti-adduct was obtained in the reaction of hydroxyacetone and p-nitorobenzaldehyde as shown in Scheme 15.70 There have been few reports on N1- and/or N4-methylated 2,5-DKPs as organocatalysts in asymmetric reactions. Lipton suggested that N1- or N4-methylated 2,5-DKPs derived from (3S,6S)-40 were more soluble in organic solvents than (3S,6S)-40, but they afforded little or no enantioselectivity in the hydrocyanation described above in Scheme 13.71 It is concluded that both amide bonds of (3S,6S)-40 are critical for enantioselective catalysis in the hydrocyanation.
3. 3,6-DIALKOXY-2,5-DIHYDROPYRAZINE
Both enantiomers of 2-isopropyl-3,6-dimethoxy-2,5-dihydropyrazine [(S)- and (R)-43] are known as the Schöllkopf chiral auxiliaries or the Schöllkopf bis-lactim ethers.3,72,73 Bis-lactim ether 44 with a second stereogenic center is easily obtained via diastereoselective addition of various electrophiles, such as alkyl halides, alkyl sulfonates, acyl chlorides, aldehydes, ketones, thioketones, epoxides, and arynes, to the deprotonated (S)- or (R)-43. Subsequently, cleavage of 44 under aqueous acidic conditions liberates the optically pure α-amino acid methyl ester 45 and (S)- or (R)-valine methyl ester [(S)- or (R)-46] as the chiral auxiliary (Scheme 16).74,75 We successfully synthesized a variety of α-substituted serines utilizing bis-lactim ethers 51 derived from diethyl 2-aminomalonate hydrochloride (47) based on the Schöllkopf synthesis of α-amino acids.76,77 Synthetic methodologies for the preparation of α-substituted serines are mainly described here as follows.
3-1. Synthesis of 3,6-dialkoxy-2,5-dihydropyrazine
The general protocol of bis-lactim ether synthesis involves condensation of two amino acids, cyclization to 2,5-DKP, and bis-lactim ether formation with Meerwein’s salts. Meerwein’s salts, also known as the Merrwein reagents, refer to trimethyl- or triethyloxonium tetrafluoroborate, which can be prepared from boron trifluoride etherate, epichlorohydrin, and the corresponding dialkyl ether.78 Scheme 17 represents the synthesis of bis-lactim ether 51 (R = i-Pr, H) derived from diethyl 2-aminomalonate hydrochloride (47) and N-Cbz-protected amino acid 48 via the formation of 2,5-DKP 50.32 Enantiomerically pure L- or D-valine derivative 48a (R = i-Pr) affords 2,5-DKP 50a as a mixture of two diastereomers without racemization at the C6 position. A racemic mixture of 2,5-DKP 50b is obtained from N-Cbz-protected glycine [48b (R = H)].
3-2. Asymmetric alkylation of 3,6-dialkoxy-2,5-dihydropyrazine
The Schöllkopf synthesis of α-amino acids is versatile and applicable to asymmetric alkylation of chiral bis-lactim ether (2S)-51a for the synthesis of α-alkylated serines in good optical purities.79 Deprotonation of (2S)-51a employing sodium hydride or n-butyllithium afforded the sodium or lithium enolate with planar geometry. Alkylation at the C5 position of the enolate under the direction of an isopropyl group at the C2 position followed by reduction with diisobutylaluminum hydride (DIBAL) afforded (2S,5S)-53 in a diastereoselective manner, as shown in Scheme 18. The absolute configurations of 52 were determined to be 2S and 5R by a 1H-1H NOE experiment (Scheme 19). Interestingly, a considerable upfield shift of C2-H of (2S,5S)-53 (R = Bn) was observed when compared with the chemical shift of (2S,5R)-53 (R = Bn) in the 1H-NMR spectrum. In addition, one of the protons of the methyl group of (2S,5R)-53 (R = Bn) exhibited a significant upfield shift in comparison with the 1H-NMR chemical shift of (2S,5S)-53 (R = Bn). Such a phenomenon seems to be rationalized in terms of the shielding effect of the phenyl ring, which probably adopts a folded conformation with the bis-lactim ether ring as shown in Scheme 20.80 Acidic hydrolysis of (2S,5S)-53 gave the corresponding α-alkylated serines (S)-54 as each enantiomerically pure compound.
In 2010, Akhmedov and co-workers reported that the dihydropirazine ring of syn- and anti-56 derived from tryptophan exists in a boat conformation based on detailed analysis of the NMR spectra of 43 and 56 (Scheme 21).81 In addition, it was concluded that the indole group of 56 adopts a folded conformation in which one diastereotopic face is effectively shielded by the aromatic indole ring in the CDCl3 solution.
3-3. Asymmetric aldol-type reaction of 3,6-dialkoxy-2,5-dihydropyrazine
Chiral bis-lactim ether 51a is available in a diastereoselective aldol-type reaction with various chiral and achiral aldehydes. It appeared that tin(II)- or magnesium(II)-mediated diastereoselective aldol-type reactions of (2S)-51a with chiral aldehyde (R)-57 proceeded in a dual chiral recognition manner. Aldol reactions of configurationally matched pairs of (2S)-51a and (R)-57 are shown in Scheme 22.82
The reaction in the presence of magnesium bromide and triethylamine afforded (2S,5R,1’S)-59 as the only diastereomeric product. On the other hand, a similar reaction of (2R)-51a with chiral aldehyde (R)-57 employing stannous trifluoromethanesulfonate and N-ethylpiperidine gave a different diastereomer (2R,5S,1’S)-59 as an exclusive product. This stereochemical outcome may be understood in terms of the presumed six-membered transition state A [containing Mg(II)-enolate] or C [containing Sn(II)-enaminate] based on the orbital overlap effect and steric repulsion, as shown in Scheme 23. In the case of transition states B [containing Sn(II)-enaminate] and D [containing Mg(II)-enolate], formation of the mismatched pairs causes the degradation of diastereoselectivity.
Various achiral aldehydes have also been used in magnesium(II)- or tin(II)-mediated diastereoselective aldol-type reactions of chiral bis-lactim ether (2S)-51a.83 In addition, these results were successfully applied to the asymmetric total synthesis of ISP-I (myriocin, thermozymocidin) (63), which is a potent immunosuppressive compound isolated from the culture broth of the fungus Isaria sinclairii (Scheme 24).84 Whereas the bulkiest substituent at the C2 position of chiral aldehyde (R)-57 is a TBDPSO group, a long-chain alkyl group is bulkiest in chiral aldehyde (R)-60. Thus, the magnesium(II)-mediated aldol-type reaction with excellent diastereoselectivity should be understood by the transition state D’ in Scheme 24. In this context, it is interesting to note that fingolimod (GilenyaTM, FTY720), which is a synthetic compound derived from ISP-I (63), was approved as a new treatment for multiple sclerosis (MS) in 2010.85
Tin(II)-mediated enantioselective aldol-type reaction of achiral bis-lactim ether 51b with various achiral aldehydes is also available in the presence of (-)-sparteine (64) as an external chiral ligand. Not only a stoichiometric amount (1.5 mol eq), but also a catalytic amount (0.3 mol eq) of 64 nicely promoted the reaction in an enantioselective manner, yielding bis-lactim ether (2S,1’R)-65 in up to 94% ee (Scheme 25).86 Although the use of stoichiometric amounts of 64 in asymmetric reactions often leads to highly enantioselective transformation, it is noteworthy that there have been far fewer applications of (-)-sparteine (64) as an asymmetric catalyst.87
Finally, some recent papers on organic reactions involving bis-lactim ethers should be mentioned. Synthesis of orthogonally protected bis-α-amino acids,88 3-aza-4-fluoro-L-phenylalanine,89 quaternary aryl amino acid derivative,90 2-aza-4-deoxypodophyllotoxins,91 anthranilimide-based glycogen phosphorylase inhibitors,92 4-fluorohistidine,93 piperidine imino sugars,94 sodium channel blockers,95 histone deacetylase inhibitors,96 and 2-amino-4-phosphonobutanoic acids97 are all notable examples.
4. CONCLUSION
In conclusion, this review has briefly summarized the chemistry of 2,5-diketopiperazine 1 and its bis-lactim ether 2 from the perspectives of synthesis, reaction, and conformation. We have also contributed to this area to some degree. These “classical” heterocycles 1 and 2 have been well-investigated in terms of organic reactions, but have been less well investigated in other regards, particularly their conformation, biological activity, and functions as organocatalysts. In the foreseeable future, as our understanding of the detailed chemistry of 2,5-diketopiperazine 1 and its bis-lactim ether 2 continues to advance, we believe these “classical” heterocycles will again be seen as important “novel” heterocycles with amazing functionality.
ACKNOWLEDGEMENTS
We are very grateful to Professor Yoshimitsu Nagao, Emeritus Professor of Tokushima University, for the helpful input and encouragement. We also thank all of our collaborators and co-workers for their intellectual and experimental contributions.
References
1. P. J. Milne and G. Kilian, “The Properties, Formation, and Biological Activity of 2,5-Diketopiperazines” in Comprehensive Natural Products II: Chemistry and Biology, ed. by L. Mander and H.-W. Liu, Elsevier, Amsterdam, 2010, pp. 657-698.
2. A. D. Borthwick, Chem. Rev., 2012, 112, 3641; CrossRef A. D. Borthwick and N. C. Da Costa, Crit. Rev. Food Sci. Nutr., 2015, in press.
3. U. Schöllkopf, W. Hartwig, and U. Groth, Angew. Chem., Int. Ed. Engl., 1979, 18, 863; CrossRef U. Schöllkopf, W. Hartwig, and U. Groth, Angew. Chem., Int. Ed. Engl., 1980, 19, 212; CrossRef U. Schöllkopf, U. Groth, and C. Deng, Angew. Chem., Int. Ed. Engl., 1981, 20, 798; CrossRef U. Schöllkopf, U. Groth, and W. Hartwig, Ann. Chem., 1981, 2407; U. Schöllkopf, Tetrahedron, 1983, 39, 2085; CrossRef U. Schöllkopf, U. Busse, R. Kilger, and P. Lehr, Synthesis, 1984, 271. CrossRef
4. D. M. Wrinch, Nature, 1936, 137, 411; CrossRef D. M. Wrinch, Science, 1937, 85, 566; CrossRef D. M. Wrinch, Nature, 1937, 139, 972; CrossRef D. M. Wrinch, Proc. Royal Soc. London A, Math. Phys. Sci., 1937, 161, 505; CrossRef D. M. Wrinch, Nature, 1939, 143, 763; CrossRef D. M. Wrinch, Nature, 1940, 145, 669; CrossRef D. M. Wrinch, Nature, 1963, 199, 564. CrossRef
5. I. Alkorta, G. Sánchez-Sanz, C. Trujillo, L. M. Azofra, and J. Elguero, Struct. Chem., 2012, 23, 873. CrossRef
6. M. Ginz and U. H. Engelhardt, J. Agric. Food Chem., 2000, 48, 3528; CrossRef M. Ginz and U. H. Engelhardt, Eur. Food Res. Technol., 2001, 213, 8. CrossRef
7. J. S. Bonvehí and F. V. Coll, Eur. Food Res. Technol., 2000, 210, 189; CrossRef T. Stark and T. Hofmann, J. Agric. Food Chem., 2005, 53, 7222. CrossRef
8. S. Sakamura, K. Furukawa, and T. Kasai, Agric. Biol. Chem., 1978, 42, 607; CrossRef M. Gautschi, J. P. Schmid, T. L. Peppard, T. P. Ryan, R. M. Tuorto, and X. Yang, J. Agric. Food Chem., 1997, 45, 3183. CrossRef
9. K. Takahashi, M. Tadenuma, K. Kitamoto, and S. Sato, Agric. Biol. Chem., 1974, 38, 927. CrossRef
10. L. A. M. Ryan, F. D. Bello, E. K. Arendt, and P. Koehler, J. Agric. Food Chem., 2009, 57, 9563. CrossRef
11. T. Kawai, Y. Ishida, H. Kakiuchi, N. Ikeda, T. Higashida, and S. Nakamura, J. Agric. Food Chem., 1991, 39, 770. CrossRef
12. M. Z. Chen, M. L. Dewis, K. Kraut, D. Merritt, L. Reiber, L. Trinnaman, and N. C. Da Costa, J. Food Sci., 2009, 74, C100. CrossRef
13. Y.-H. Chen, S.-E. Liou, and C.-C. Chen, Eur. Food Res. Technol., 2004, 218, 589; CrossRef N. Tsuruoka, Y. Beppu, H. Koda, N. Doe, H. Watanabe, and K. Abe, PLOS ONE, 2012, 7, e50824. CrossRef
14. T. Hagiwara, T. Yasuno, S. Suzuki, and K. Saito, J. Food Hyg. Soc. Jpn., 1999, 40, 62. CrossRef
15. D. E. Nitecki, B. Halpern, and J. W. Westley, J. Org. Chem., 1968, 33, 864. CrossRef
16. S. Hirano, S. Ichikawa, and A. Matsuda, Bioorg. Med. Chem., 2008, 16, 428. CrossRef
17. K. Suzuki, Y. Sasaki, N. Endo, and Y. Mihara, Chem. Pharm. Bull., 1981, 29, 233; CrossRef K. M. Depew, S. P. Marsden, D. Zatorska, A. Zatorski, W. G. Bornmann, and S. J. Danishefsky, J. Am. Chem. Soc., 1999, 121, 11953; CrossRef M. Marchini, M. Mingozzi, R. Colombo, I. Guzzetti, L. Belvisi, F. Vasile, D. Potenza, U. Piarulli, D. Arosio, and C. Gennari, Chem. Eur. J., 2012, 18, 6195; CrossRef C. Challa, N. Kumar, M. John, and R. S. Lankalapalli, Med. Chem. Res., 2014, 23, 2377. CrossRef
18. F. Airaghi, A. Fiorati, G. Lesma, M. Musolino, A. Sacchetti, and A. Silvani, Beilstein J. Org. Chem., 2013, 9, 147; CrossRef M. B. Martins-Teixeira, V. L. Campo, M. Biondo, R. Sesti-Costa, Z. A. Carneiro, J. S. Silva, and I. Carvalho, Bioorg. Med. Chem., 2013, 21, 1978. CrossRef
19. K. S. Ashton, M. Denti, M. H. Norman, and D. J. St. Jean Jr., Tetrahedron Lett., 2014, 55, 4501. CrossRef
20. P. C. Pereira, I. W. C. E. Arends, and R. A. Sheldon, Tetrahedron: Asymmetry, 2014, 25, 825. CrossRef
21. M. Tullberg, M. Grøtli, and K. Luthman, Tetrahedron, 2006, 62, 7484; CrossRef M. Tullberg, M. Grøtli, and K. Luthman, J. Org. Chem., 2007, 72, 195; CrossRef N. S. Simpkins, I. Pavlakos, M. D. Weller, and L. Male, Org. Biomol. Chem., 2013, 11, 4957. CrossRef
22. L. A. Thompson and J. A. Ellman, Chem. Rev., 1996, 96, 555; CrossRef Y.-W. Pan, C.-W. Guo, H.-Y. Tu, C.-W. Tsai, and W.-C. Cheng, ACS Comb. Sci., 2013, 15, 425. CrossRef
23. J. C. O’Neill and H. E. Blackwell, Comb. Chem. High T. Scr., 2007, 10, 857.
24. C. R. B. Rhoden, D. G. Rivera, O. Kreye, A. K. Bauer, B. Westermann, and L. A. Wessjohann, J. Comb. Chem., 2009, 11, 1078; CrossRef A. Hartung, F. Seufert, C. Berges, V. H, Gessner, and U. Holzgrabe, Molecules, 2012, 17, 14685; CrossRef V. Tyagi, S. Khan, and P. M. S. Chauhan, Synlett, 2013, 24, 1291. CrossRef
25. S. Cho, G. Keum, S. B. Kang, S.-Y. Han, and Y. Kim, Mol. Div., 2003, 6, 283; CrossRef J. P. Bourgault, A. R. Maddirala, and P. R. Andreana, Org. Biomol. Chem., 2014, 12, 8125. CrossRef
26. A. S. M. Ressurreição, R. Delatouche, C. Gennari, and U. Piarulli, Eur. J. Org. Chem., 2011, 217; CrossRef L. Vahdati, R. Fanelli, G. Bernadat, I. Correia, O. Lequin, S. Ongeri, and U. Piarulli, New J. Chem., 2015, 39, 3250. CrossRef
27. R. B. Corey, J. Am. Chem. Soc., 1938, 60, 1598; CrossRef D. L. Dorset, Z. Kristallogr., 2010, 225, 86.
28. A. P. Mendham, J. Spencer, B. Z. Chowdhry, T. J. Dines, M. Mujahid, R. A. Palmer, G. J. Tizzard, and S. J. Coles, J. Chem. Crystallogr., 2011, 41, 1323. CrossRef
29. J. D. Hirst and B. J. Persson, J. Phys. Chem. A, 1998, 102, 7519; CrossRef Y. Zhu, M. Tang, X. Shi, and Y. Zhao, Int. J. Quantum Chem., 2007, 107, 745. CrossRef
30. K. D. Kopple and D. H. Marr, J. Am. Chem. Soc., 1967, 89, 6193; CrossRef K. D. Kopple and M. Ohnishi, J. Am. Chem. Soc., 1969, 91, 962; CrossRef X. Li, K. H. Hopmann, J. Hudecová, J. Isaksson, J. Novotná, W. Stensen, V. Andrushchenko, M. Urbanová, J.-S. Svendsen, P. Bouř, and K. Ruud, J. Phys. Chem. A, 2013, 117, 1721; CrossRef Y. P. Hong, S.-H. Lee, J.-H. Choi, A. Kashima, G. Nakamura, and T. Suzuki, Bull. Korean Chem. Soc., 2014, 35, 2299. CrossRef
31. S. Sano, M. Nakao, M. Takeyasu, S. Kitaike, Y. Yoshioka, and Y. Nagao, Heterocycles, 2009, 79, 781. CrossRef
32. S. Sano, K. Hayashi, T. Miwa, T. Ishii, M. Fujii, H. Miwa, and Y. Nagao, Tetrahedron Lett., 1998, 39, 5571; CrossRef S. Sano, Yakugaku Zasshi, 2000, 120, 28; S. Sano and Y. Nagao, J. Synth. Org. Chem., Jpn., 2000, 58, 756; CrossRef S. Sano, M. Nakao, M. Takeyasu, T. Honjo, and Y. Nagao, Lett. Org. Chem., 2006, 3, 764; CrossRef S. Sano, M. Nakao, M. Takeyasu, C. Yamamoto, S. Kitaike, Y. Yoshioka, and Y. Nagao, Open Org. Chem. J., 2009, 3, 22. CrossRef
33. M. Nakao, Y. Toriuchi, S. Fukayama, and S. Sano, Chem. Lett., 2014, 43, 340. CrossRef
34. M. Nishio, Y. Umezawa, M. Hirota, and Y. Takeuchi, Tetrahedron, 1995, 51, 8665; CrossRef Y. Umezawa, S. Tsuboyama, K. Honda, J. Uzawa, and M. Nishio, Bull. Chem. Soc. Jpn., 1998, 71, 1207; CrossRef Y. Umezawa, S. Tsuboyama, H. Takahashi, J. Uzawa, and M. Nishio, Bioorg. Med. Chem., 1999, 7, 2021; CrossRef Y. Umezawa, S. Tsuboyama, H. Takahashi, J. Uzawa, and M. Nishio, Tetrahedron, 1999, 55, 10047; CrossRef H. Suezawa, T. Hashimoto, K. Tsuchinaga, T. Yoshida, T. Yuzuri, K. Sakakibara, M. Hirota, and M. Nishio, J. Chem. Soc., Perkin Trans. 2, 2000, 1243; CrossRef M. Nishio, CrystEngComm, 2004, 6, 130; CrossRef O. Takahashi, Y. Kohno, and M. Nishio, Chem. Rev., 2010, 110, 6049; CrossRef M. Nishio, Phys. Chem. Chem. Phys., 2011, 13, 13873. CrossRef
35. G. G. Fava, M. F. Belicchi, R. Marchelli, and A. Dossena, Acta Cryst., 1981, B37, 625; CrossRef A. P. Mendham, T. J. Dines, M. J. Snowden, R. Withnall, and B. Z. Chowdhry, J. Raman Spectrosc., 2009, 40, 1508. CrossRef
36. M. Bressan, R. Ettorre, F. Marchiori, and G. Valle, Int. J. Pept. Protein Res., 1982, 19, 402. CrossRef
37. G. T. R. Palmore, T.-J. M. Luo, M. T. McBride-Wieser, E. A. Picciotto, and C. M. Reynoso-Paz Chem. Mater., 1999, 11, 3315; CrossRef G. T. R. Palmore and M. T. McBride, Chem. Commun., 1998, 145; CrossRef S. Palacin, D. N. Chin, E. E. Simanek, J. C. MacDonald, G. M. Whitesides, M. T. McBride, and G. T. R. Palmore, J. Am. Chem. Soc., 1997, 119, 11807. CrossRef
38. K. B. Joshi and S. Verma, Tetrahedron Lett., 2008, 49, 4231. CrossRef
39. F. M. Brunel and A. F. Spatola, J. Peptide Res., 2004, 63, 213. CrossRef
40. M. Nakao, Y. Hiroyama, Y. Toriuchi, and S. Sano, Abstracts of the 40th Congress of Heterocyclic Chemistry, Sendai, Japan, 2010, pp. 257-258.
41. M. B. Martins and I. Carvalho, Tetrahedron, 2007, 63, 9923; CrossRef Y. Wang, P. Wang, H. Ma, and W. Zhu, Expert. Opin. Ther. Pat., 2013, 23, 1415; CrossRef M. M. M. Santos, Tetrahedron, 2014, 70, 9735. CrossRef
42. T. Lindel, “Short Syntheses of the Spirotryprostatins” in Organic Synthesis Highlights V, ed. by H.-G. Schmalz and T. Wirth, Wiley-VCH Verlag GmbH, Weinheim, Germany, 2003, pp. 360-367. CrossRef
43. S. D. Edmondson and S. J. Danishefsky, Angew. Chem. Int. Ed., 1998, 37, 1138; CrossRef S. Edmondson, S. J. Danishefsky, L. Sepp-Lorenzino, and N. Rosen, J. Am. Chem. Soc., 1999, 121, 2147; CrossRef T. Onishi, P. R. Sebahar, and R. M. Williams, Org. Lett., 2003, 5, 3135; CrossRef T. Onishi, P. R. Sebahar, and R. M. Williams, Tetrahedron, 2004, 60, 9503; CrossRef K. Kitahara, J. Shimokawa, and T. Fukuyama, Chem. Sci., 2014, 5, 904. CrossRef
44. R. J. Cole, J. W. Kirksey, J. H. Moore, B. R. Blankenship, U. L. Diener, and N. D. Davis, Appl. Microbiol., 1972, 24, 248; J. Fayos, D. Lokensgard, J. Clardy, R. J. Cole, and J. W. Kirksey, J. Am. Chem. Soc., 1974, 96, 6785; CrossRef L. Hotujac, R. H. Muftić, and N. Filipović, Pharmacology, 1976, 14, 297; CrossRef H.-G. Knaus, O. B. McManus, S. H. Lee, W. A. Schmalhofer, M. Garcia-Calvo, L. M. H. Helms, M. Sanchez, K. Giangiacomo, and J. P. Reuben, Biochemisry, 1994, 33, 5819; CrossRef K. Khoufache, O. Puel, N. Loiseau, M. Delaforge, D. Rivollet, A. Coste, C. Cordonnier, E. Escudier, F. Botterel, and S. Bretagne, BMC Microbiol., 2007, 7, 5; CrossRef D. Kulke, G. von Samson-Himmelstjerna, S. M. Miltsch, A. J. Wolstenholme, A. R. Jex, R. B. Gasser, C. Ballesteros, T. G. Geary, J. Keiser, S. Townson, A. Harder, and J. Krücken, PLOS Negl. Trop. Dis., 2014, 8, e3401. CrossRef
45. M. Kusano, G. Sotoma, H. Koshino, J. Uzawa, M. Chijimatsu, S. Fujioka, T. Kawano, and Y. Kimura, J. Chem. Soc., Perkin Trans. 1, 1998, 2823; CrossRef K. Matsumura and T. Kitahara, Heterocycles, 2001, 54, 727; CrossRef K. Sprogøe, S. Manniche, T. O. Larsen, and C. Christophersen, Tetrahedron, 2005, 61, 8718; CrossRef J. Ruchti and E. M. Carreira, J. Am. Chem. Soc., 2014, 136, 16756. CrossRef
46. S.-M. Li, Nat. Prod. Rep., 2010, 27, 57. CrossRef
47. K. Kanoh, S. Kohno, T. Asari, T. Harada, J. Katada, M. Muramatsu, H. Kawashima, H. Sekiya, and I. Uno, Bioorg. Med. Chem. Lett., 1997, 7, 2847; CrossRef K. Kanoh, S. Kohno, J. Katada, J. Takahashi, and I. Uno, J. Antibiot., 1999, 52, 134; CrossRef K. Kanoh, S. Kohno, J. Katada, Y. Hayashi, M. Muramatsu, and I. Uno, Biosci. Biotechnol. Biochem., 1999, 63, 1130; CrossRef Y. Hayashi, S. Orikasa, K. Tanaka, K. Kanoh, and Y. Kiso, J. Org. Chem., 2000, 65, 8402; CrossRef L. Perrin, C. Aninat, V. Hamon, Y. Hayashi, C. Abadie, B. Heyd, F. André, and M. Delaforge, Drug Metab. Dispos., 2008, 36, 2381; CrossRef Y. Hayashi, Y. Yamazaki-Nakamura, and F. Yakushiji, Chem. Pharm. Bull., 2013, 61, 889. CrossRef
48. I. R. Shimi, N. Abedallah, and S. Fathy, Antimicrob. Agents Chemother., 1977, 11, 373; CrossRef I. R. Shimi, S. Shoukry, and S. Fathey, Int. J. Biochem., 1979, 10, 511; CrossRef A. Kumar, M. Singh, and V. S. Chauhan, Indian J. Chem. Sect. B, 1986, 25, 230; I. L. Rodionov, L. N. Rodionova, L. K. Baidakova, A. M. Romashko, T. A. Balashova, and V. T. Ivanov, Tetrahedron, 2002, 58, 8515. CrossRef
49. T. Miyoshi, N. Miyairi, H. Aoki, M. Kohsaka, H. Sakai, and H. Imanaka, J. Antibiot., 1972, 25, 569; CrossRef T. Kamiya, S. Maeno, M. Hashimoto, and Y. Mine, J. Antibiot., 1972, 25, 576; CrossRef M. Nishida, Y. Mine, T. Matsubara, S. Goto, and S. Kuwahara, J. Antibiot., 1972, 25, 582; CrossRef R. M. Williams, R. W. Armstrong, and J. S. Dung, J. Am. Chem. Soc., 1984, 106, 5748; CrossRef R. M. Williams and C. A. Durham, Chem. Rev., 1988, 88, 511; CrossRef A. Santillán, Jr., X. Zhang, J. Hardesty, W. R. Widger, and H. Kohn, J. Med. Chem., 1998, 41, 1185; CrossRef H. Kohn and W. Widger, Curr. Drug Targets Infect. Disord., 2005, 5, 273; CrossRef M. Malik, L. Li, X. Zhao, R. J. Kerns, J. M. Berger, and K. Drlica, J. Antimicrob. Chemother., 2014, 69, 3227. CrossRef
50. A. C. Stierle, J. H. Cardellina, II, and G. A. Strobel, Proc. Natl. Acad. Sci. USA, 1988, 85, 8008; CrossRef S. H Park, A. Stierle, and G. A. Strobel, Phytochemistry, 1993, 35, 101; CrossRef M. M. Bobylev, L. I. Bobyleva, and G. A. Strobel, J. Agric. Food Chem., 1996, 44, 3960; CrossRef S. C. D. N. Lopes, A. Fedorov, and M. A. R. B. Castanho, Steroids, 2004, 69, 825; CrossRef K. Nakagawa, K. Takada, and N. Imamura, Biosci. Biotechnol. Biochem., 2013, 77, 1449. CrossRef
51. R. R. King, C. H. Lawrence, M. C. Clark, and L. A. Calhoun, J. Chem. Soc., Chem. Commun., 1989, 849; CrossRef R. R. King and C. H. Lawrence, J. Agric. Food Chem., 2001, 49, 2298; CrossRef R. R. King and L. A. Calhoun, Phytochemistry, 2009, 70, 833; CrossRef H. Zhang, X. Ning, H. Hang, X. Ru, H. Li, Y. Li, L. Wang, X. Zhang, S. Yu, Y. Qiao, X. Wang, and P. G. Wang, Org. Lett., 2013, 15, 5670; CrossRef J. P. Bourgault, A. R. Maddirala, and P. R. Andreana, Org. Biomol. Chem., 2014, 12, 8125. CrossRef
52. J. W. Mason and J. G. Kidd, J. Immunol., 1951, 66, 99; T. Fukuyama and Y. Kishi, J. Am. Chem. Soc., 1976, 98, 6723; CrossRef P. Waring, R. D. Eichner, and A. Müllbacher, Med. Res. Rev., 1988, 8, 499; CrossRef M. S. Shathele, Asian J. Anim. Sci., 2011, 5, 285; A. Geissler, F. Haun, D. O. Frank, K. Wieland, M. M. Simon, M. Idzko, R. J. Davis, U. Maurer, and C. Borner, Cell Death Differ., 2013, 20, 1317; CrossRef P. Waring and C. L. L. Chai, Aust. J. Chem., 2015, 68, 178.
53. M. I. Sanz Ferramola, D. Benuzzi, V. Calvente, J. Calvo, G. Sansone, S. Cerutti, and J. Raba, “The use of siderophores for improving the control of postharvest diseases in stored fruits and vegetables” in Microbial Pathogens and Strategies for Combating Them: Science, Technology and Education, ed. by A. Méndez-Vilas, Formatex Res. Center, 2013, Vol. 2, pp. 1385-1394.
54. C. L. Atkin and J. B. Neilands, Biochemistry, 1968, 7, 3734; CrossRef R. W. Grady, C. M. Peterson, R. L. Jones, J. H. Graziano, K. K. Bhargava, V. A. Berdoukas, G. Kokkini, D. Loukopoulos, and A. Cerami, J. Pharmacol. Exp. Ther., 1979, 209, 342; G. Müller, B. F. Matzanke, and K. N. Raymond, J. Bacteriol., 1984, 160, 313; G. Müller, S. J. Barclay, and K. N. Raymond, J. Biol. Chem., 1985, 260, 13916; D. Andersen, J. C. Renshaw, and M. G. Wiebe, Mycol. Res., 2003, 107, 949; CrossRef J. M. Scervino, I. Sampedro, M. A. Ponce, M. A. Rodriguez, J. A. Ocampo, and A. Godeas, Soil Biol. Biochem., 2008, 40, 2474; CrossRef T. Funahashi, T. Tanabe, K. Mihara, K. Miyamoto, H. Tsujibo, and S. Yamamoto, Biol. Pharm. Bull., 2012, 35, 753; CrossRef L. Rajpert, A. Skłodowska, and R. Matlakowska, Chemosphere, 2013, 91, 1257. CrossRef
55. R. Saha, N. Saha, R. S. Donofrio, and L. L. Bestervelt, J. Basic Microbiol., 2013, 53, 303. CrossRef
56. M. Nakao, S. Fukayama, S. Kitaike, and S. Sano, Heterocycles, 2015, 90, 1309. CrossRef
57. A. D. Borthwick and J. Liddle, “Retosiban and Epelsiban: Potent and Selective Orally Available Oxytocin Antagonists” in Methods and Principles in Medicinal Chemistry: Protein-Protein Interactions in Drug Discovery, ed. by A. Dömling, Wiley-VCH Verlag GmbH & Co. KGaA, Weinheim, Germany, 2013, Vol. 56, pp. 225-256.
58. A. D. Borthwick and J. Liddle, Med. Res. Rev., 2011, 31, 576. CrossRef
59. P. Yeni, A. LaMarca, D. Berger, P. Cimoch, A. Lazzarin, P. Salvato, F. M. Smaill, E. Teofilo, S. J. Madison, W. G. Nichols, K. K. Adkison, T. Bonny, J. Millard, D. McCarty, and the EPIC (CCR100136) study team, HIV Medicine, 2009, 10, 116; CrossRef R. Nishizawa, T. Nishiyama, K. Hisaichi, K. Hirai, H. Habashita, Y. Takaoka, H. Tada, K. Sagawa, S. Shibayama, K. Maeda, H. Mitsuya, H. Nakai, D. Fukushima, and M. Toda, Bioorg. Med. Chem., 2010, 18, 5208; CrossRef J. Gavrilyuk, H. Ban, H. Uehara, S. J. Sirk, K. Saye-Francisco, A. Cuevas, E. Zablowsky, A. Oza, M. S. Seaman, D. R. Burton, and C. F. Barbas III, J. Virol., 2013, 87, 4985. CrossRef
60. R. Petersen, S. T. Le Quement, and T. E. Nielsen, Angew. Chem. Int. Ed., 2014, 53, 11778. CrossRef
61. K. A. Ahrendt, C. J. Borths, and D. W. C. MacMillan, J. Am. Chem. Soc., 2000, 122, 4243; CrossRef G. Lelais and D. W. C. MacMillan, Aldrichimica Acta, 2006, 39, 79; T. D. Beeson, A. Mastracchio, J.-B. Hong, K. Ashton, and D. W. C. MacMillan, Science, 2007, 316, 582. CrossRef
62. H. Pracejus, Liebigs Ann. Chem., 1960, 634, 9; CrossRef H. Pracejus, J. Mätje, J. Prakt. Chem., 1964, 24, 195. CrossRef
63. U. Eder, G. Sauer, and R. Wiechert, Angew. Chem., Int. Ed. Engl., 1971, 10, 496; CrossRef Z. G. Hajos and D. R. Parrish, J. Org. Chem., 1974, 39, 1615. CrossRef
64. P. I. Dalko and L. Moisan, Angew. Chem. Int. Ed., 2001, 40, 3726; CrossRef P. I. Dalko and L. Moisan, Angew. Chem. Int. Ed., 2004, 43, 5138; CrossRef J. Alemán and S. Cabrera, Chem. Soc. Rev., 2013, 42, 774. CrossRef
65. E. R. Jarvo and S. J. Miller, Tetrahedron, 2002, 58, 2481. CrossRef
66. K. Tanaka, A. Mori, and S. Inoue, J. Org. Chem., 1990, 55, 181. CrossRef
67. F. Schoenebeck and K. N. Houk, J. Org. Chem., 2009, 74, 1464. CrossRef
68. M. S. Iyer, K. M. Gigstad, N. D. Namdev, and M. Lipton, J. Am. Chem. Soc., 1996, 118, 4910; CrossRef M. S. Iyer, K. M. Gigstad, N. D. Namdev, and M. Lipton, Amino Acids, 1996, 11, 259. CrossRef
69. X.-H. Cai and B. Xie, ARKIVOC, 2014, i, 205. CrossRef
70. R. Wada, M. Nakao, and S, Sano, Abstracts of the 52nd Annual Meeting of the Chugoku-Shikoku Branch of the Pharmaceutical Society of Japan, Matsuyama, Japan, 2013, pp. 132.
71. J. C. Thoen and M. A. Lipton, Tetrahedron: Asymmetry, 1997, 8, 3947. CrossRef
72. W. Hartwig and J. Mittendorf, “(2S)-(+)-2,5-Dihydro-2-isopropyl-3,6-dimethoxypyrazine” in Handbook of Reagents for Organic Synthesis: Chiral Reagents for Asymmetric Synthesis, ed. by L. A. Paquette, John Wiley & Sons, Chichester, 2003, pp. 219-221.
73. S. D. Bull, S. G. Davies, and W. O. Moss, Tetrahedron: Asymmetry, 1998, 9, 321; CrossRef A.-C. Carlsson, F. Jam, M. Tullberg, Å. Pilotti, P. Ioannidis, K. Luthman, and M. Grøtli, Tetrahedron Lett., 2006, 47, 5199. CrossRef
74. R. M. Williams, “Asymmetric Derivatization of Glycine: Glycine and Related Enolates – Bis-Lactim Ethers” in Synthesis of Optically Active α-Amino Acids, Pergamon Press, Oxford, 1989, pp. 1-33.
75. U. Schöllkopf, Pure Appl. Chem., 1983, 55, 1799; CrossRef U. Schöllkopf, Top. Current Chem., 1983, 109, 65. CrossRef
76. T. Wirth, “New Strategies to α-Alkylated α-Amino Acids” in Organic Synthesis Highlights IV, ed. by H.-G. Schmalz, Wiley-VCH Verlag GmbH, Weinheim, Germany, 2000, pp. 26-33. CrossRef
77. T. Wirth, Angew. Chem., Int. Ed. Engl., 1997, 36, 225. CrossRef
78. H. Meerwein, Org. Synth., 1973, Coll. Vol. 5, 1080; H. Meerwein, Org. Synth., 1973, Coll. Vol. 5, 1096; S. Pichlmair, Synlett, 2004, 195. CrossRef
79. S. Sano, M. Takebayashi, T. Miwa, T. Ishii, and Y. Nagao, Y. Tetrahedron: Asymmetry, 1998, 9, 3611. CrossRef
80. M. Bolte, B. Benecke, and E. Egert, Acta Cryst., 1999, C55, 968.
81. N. G. Akhmedov, C. A. Dacko, A. Güven, and B. C. G. Söderberg, Magn. Reson. Chem., 2010, 48, 134. CrossRef
82. S. Sano, X.-K. Liu, M. Takebayashi, Y. Kobayashi, K. Tabata, M. Shiro, and Y. Nagao, Tetrahedron Lett., 1995, 36, 4101. CrossRef
83. S. Sano, T. Miwa, X.-K. Liu, T. Ishii, T. Takehisa, M. Shiro, and Y. Nagao, Tetrahedron: Asymmetry, 1998, 9, 3615. CrossRef
84. S. Sano, Y. Kobayashi, T. Kondo, M. Takebayashi, S. Maruyama, T. Fujita, and Y. Nagao, Tetrahedron Lett., 1995, 36, 2097. CrossRef
85. T. Fujita, N. Matsumoto, S. Uchida, T. Kohno, T. Shimizu, R. Hirose, K. Yanada, W. Kurio, and K. Watabe, Bioorg. Med. Chem. Lett., 2000, 10, 337; CrossRef C. R. Strader, C. J. Pearce, and N. H. Oberlies, J. Nat. Prod., 2011, 74, 900; CrossRef M. Kipp and S. Amor, Mult. Scler. J., 2012, 18, 258. CrossRef
86. S. Sano, T. Ishii, T. Miwa, and Y. Nagao, Tetrahedron Lett., 1999, 40, 3013. CrossRef
87. O. Chuzel and O. Riant, Top. Organomet. Chem., 2005, 15, 59. CrossRef
88. P.-T. Chen, C.-K. Lin, C.-J. Tsai, D.-Y. Huang, F.-Y. Nien, W.-W. Lin, and W.-C. Cheng, Chem. Asian J., 2015, 10, 474. CrossRef
89. M. Adachi, M. Nakajima, and M. Isobe, Biosci. Biotechnol. Biochem., 2015, in press.
90. E. P. Jones, P. Jones, and A. G. M. Barrett, Org. Lett., 2011, 13, 1012; CrossRef E. P. Jones, P. Jones, A. J. P. White, and A. G. M. Barrett, Beilstein J. Org. Chem., 2011, 7, 1570. CrossRef
91. Y. Usami, M. Arimoto, K. Kobayashi, M. Honjou, M. Yamanaka, M. Miyao, H. Ichikawa, K. F. Bastow, and K.-H. Lee, Heterocycles, 2009, 78, 2041. CrossRef
92. S. M. Sparks, P. Banker, D. M. Bickett, D. C. Clancy, S. H. Dickerson, D. M. Garrido, P. L. Golden, A. J. Peat, L. R. Sheckler, F. X. Tavares, S. A. Thomson, and J. E. Weiel, Bioorg. Med. Chem. Lett., 2009, 19, 981. CrossRef
93. J. Hajduch, J. C. Cramer, and K. L. Kirk, J. Fluorine Chem., 2008, 129, 807. CrossRef
94. M. Ruiz, T. M. Ruanova, O. Blanco, F. Núñez, C. Pato, and V. Ojea, J. Org. Chem., 2008, 73, 2240; CrossRef O. Blanco, C. Pato, M. Ruiz, and V. Ojea, Org. Biomol. Chem., 2008, 6, 3967. CrossRef
95. S. B. Hoyt, C. London, D. Gorin, M. J. Wyvratt, M. H. Fisher, C. Abbadie, J. P. Felix, M. L. Garcia, X. Li, K. A. Lyons, E. McGowan, D. E. MacIntyre, W. J. Martin, B. T. Priest, A. Ritter, M. M. Smith, V. A. Warren, B. S. Williams, G. J. Kaczorowski, and W. H. Parsons, Bioorg. Med. Chem. Lett., 2007, 17, 4630; CrossRef C. London, S. B. Hoyt, W. H. Parsons, B. S. Williams, V. A. Warren, R. Tschirret-Guth, M. M. Smith, B. T. Priest, E. McGowan, W. J. Martin, K. A. Lyons, X. Li, B. V. Karanam, N. Jochnowitz, M. L. Garcia, J. P. Felix, B. Dean, C. Abbadie, G. J. Kaczorowski, and J. L. Duffy, Bioorg. Med. Chem. Lett., 2008, 18, 1696. CrossRef
96. P. Jones, S. Altamura, P. K. Chakravarty, O. Cecchetti, R. De Francesco, P. Gallinari, R. Ingenito, P. T. Meinke, A. Petrocchi, M. Rowley, R. Scarpelli, S. Serafini, and C. Steinkühler, Bioorg. Med. Chem. Lett., 2006, 16, 5948. CrossRef
97. M. C. Fernández, A. Díaz, J. J. Guillín, O. Blanco, M. Ruiz, and V. Ojea, J. Org. Chem., 2006, 71, 6958. CrossRef