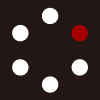
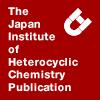
HETEROCYCLES
An International Journal for Reviews and Communications in Heterocyclic ChemistryWeb Edition ISSN: 1881-0942
Published online by The Japan Institute of Heterocyclic Chemistry
e-Journal
Full Text HTML
Received, 22nd July, 2008, Accepted, 5th September, 2008, Published online, 9th September, 2008.
DOI: 10.3987/COM-08-S(F)53
■ Novel Photoinduced Electron Transfer-initiated Cyclization of 1,2,4-Triazole-substituted α-Dehydronaphthylalaninamides in the Presence of Triethylamine
Kei Maekawa, Atsushi Tomod, Tetsutaro Igarashi, and Tadamitsu Sakurai*
Material and Life Chemistry, Faculty of Engineering, Kanagawa University, 3-27-1 Rokkakubashi, Kanagawa-ku, Yokohama 221-8686, Japan
Abstract
The irradiation of the title compounds [(Z)-1] in nitrogen-saturated methanol containing triethylamine (TEA) at room temperature was found to quantitatively afford 2(1H)-benzo[f]quinolinone (2) and 3,4-dihydro-3-(3-methyl-5-substituted 1,2,4-triazol-4-yl)-2(1H)-benzo[f]quinolinone (3) derivatives, which were described as being formed via electron transfer from TEA to the excited-state (E)-1. Analysis of solvent and substituent effects on the composition ratio of 2 to 3 confirmed that this ratio is increased from 0.2 to 9.0 with an increase in the proton-donating ability and polarity of solvent as well as in the electron-withdrawing ability of substituent bonded to the triazole ring.Excited-state chemistry for organic molecules has continued to contribute to the development of convenient methods for synthesizing pharmaceutically useful heterocyclic compounds.1 Particularly, photoinduced electron transfer (PET) reactions have attracted much recent attention owing to the potential that these reactions are able to construct many types of heteroatom-containing ring systems with high efficiencies.1a,c,d Taking into account the fact that intra- and intermolecular PET reactions in amino-substituted stilbene and styrene derivatives result in an efficient conversion of these derivatives into the nitrogen-containing heterocycles,2 we embarked on a systematic study concerning the PET reaction of N-acyl-α-dehydroarylalanines bearing a photoreactive C=C double bond.3 So far we have succeeded in constructing the dihydroquinolinone, isoquinoline and dihydrooxazole rings by controlling these PET reactions. In the course of the study described above, the ring-opening reaction of (Z)-2-methyl-4-(1-naphthylmethylene)-5(4H)-oxazolone with acetohydrazide was found to give (Z)-2-(3,5-dimethyl-1,2,4-triazol-4-yl)-3-(1-naphthyl)-2-propenoic acid.4 This unexpected finding prompted us to investigate the PET reaction of 1,2,4-triazole-substituted propenoic acid derivatives because little is known about the photochemical behavior of these triazoles.5 In order to develop a new mode of PET-initiated cyclization and to shed some light on the cyclization mechanism, we designed and synthesized (Z)-2-(3-methyl-5-substituted 1,2,4-triazol-4-yl)-3-(1-naphthyl)-2-propenamides [(Z)-1a–h; hereafter, referred to as 1,2,4-triazole-substituted α-dehydronaphthylalaninamides] (Chart 1) and examined solvent and substituent effects on the photoreactivity of (Z)-1 in the presence of triethylamine (TEA) and the selectivity of (Z)-1-derived photoproducts.
The starting (Z)-isomers [(Z)-1a–h] were prepared in good yields by the ring-opening reactions of (Z)-4-(1-naphthylmethylene)-2-methyl-5(4H)-oxazolone with equimolar amounts of the corresponding N-substituted hydrazides in acetonitrile, followed by the condensation of the resulting triazole-substituted 2-propenoic acids with primary amines in 1,4-dioxane or dimethyl formamide.6 After a nitrogen-saturated methanol solution of (Z)-1a (4.0×10–3 mol dm–3, 200 mL) containing TEA (0.10 mol dm–3) was irradiated with Pyrex-filtered light (λ>280 nm) from a 400 W high-pressure Hg lamp for 0.5 h at room temperature (conversion, 91%), the reaction mixture obtained was subjected to preparative thin layer or column chromatography over silica gel (eluent: EtOAc, CHCl3 or CHCl3-MeOH). Usual workup allowed us to isolate 1-methyl-2(1H)-benzo[f]quinolinone (2a; isolated yield, 65%), 3,4-dihydro-3-(3,5-dimethyl-1,2,4-triazol-4-yl)-1-methyl-2(1H)-benzo[f]quinolinone (3a; 15%) and 3,5-dimethyl-1,2,4-triazole (4a; 40%).7 Although any attempts were not fruitful to isolate (E)-1a, a 1H NMR spectral analysis of the reaction mixture obtained by the 1 min irradiation of the above methanol solution provided a strong indication of the existence of this (E)-isomer. The same product distribution was observed when methanol solutions of (Z)-1b–h were irradiated under the same conditions as above (Scheme 1).
In addition to the extremely high photostability of 2a–4a, the finding that the photoreaction of (Z)-1a in methanol-TEA ([(Z)-1a]= 4.0×10–3 mol dm–3; [TEA]= 0.10 mol dm–3, 10 mL) proceeds cleanly without forming any other products under the parallel irradiation (made using a merry-go-round-type irradiation equipment) allowed us to monitor this reaction by means of 1H NMR spectroscopy (Table 1). The data of the Table demonstrate preferential formation of the benzoquinolinone derivative 2a and, hence, the facile departure of the triazole ring from an (E)-1a anion radical intermediate probably formed via PET.
Because the aminocarbonyl nitrogen in (Z)-1a is not able to cyclize to the products 2a and 3a without its isomerization, the (E)-1a anion radical may serve as a precursor to these two cyclization products. In order to obtain evidence in support of a PET mechanism, the effects of TEA concentration on the fluorescence of (Z)-1a ([(Z)-1a]= 5.0×10–4 mol dm–3; [TEA]= 0–0.10 mol dm–3) and on the (Z)-1a-derived product distribution and composition ([(Z)-1a]= 4.0×10–3 mol dm–3; [TEA]= 0–0.010 mol dm–3; λ>280 nm, parallel irradiation) were examined in nitrogen-saturated methanol. The former effect led us to derive the Stern-Volmer equation: I0/I = 1 + 1.9[TEA], where I and I0 refer to the fluorescence intensities of 1a with and without TEA, respectively. The latter effect permitted the observation that in the absence of TEA the 1 h irradiation affords 2a, 3a and 4a in the composition ratio of about 1:1:1 along with minor amounts of unknown products, showing that the olefinic carbon and triazole nitrogen single bond [C(C=O)–N bond] is susceptible to homolytic cleavage eventually giving 2a–4a as major photoproducts. Additionally, the decrease in TEA concentration (0.10→0.010 mol dm–3) exerted a negligible effect on the composition ratio of 2a to 3a (3.2→3.2 at the 1h irradiation). Thus, the amine concentration effects described above substantiate the almost exclusive participation of a PET mechanism in the TEA concentration range of 0.010–0.10 mol dm–3.
Taking into account the potential that the use of (Z)-1a in which the amide hydrogen labeled with a deuterium atom provides significant information regarding a PET mechanism, after the H-D exchange reaction of (Z)-1a (4.0×10–3 mol dm–3) in MeOD-TEA (0.10 mol dm–3, 10 mL) had been completed (12 h incubation), deuteriated 1a [(Z)-1a-d] was irradiated for 1 h on a merry-go-round-type irradiation equipment (Scheme 2). 1H NMR spectral analysis of 2a-d and 3a-d in DMSO-d6, obtained after usual work-up, clearly showed incorporation of a deuterium atom into the 3-positions on the quinolinone and dihydroquinolinone rings. If we combine this finding with the electron-withdrawing 1,2,4-triazol-4-yl
group5 as well as with the mechanism previously proposed for the PET reaction of N-acyl-α-dehydronaphthylalanines,4 we are led to propose Scheme 3 in which an initially-formed naphthylmethylene anion radical intermediate [(E)-IA] and an anion radical intermediate [(E)-IB] formed by intramolecular ET in (E)-IA, which are in equilibrium with each other, are assumed to play key roles in controlling the composition ratio of 2 to 3. As already discussed in our previous study,3a,b hydrogen shift and back ET in the (E)-IA/TEA cation radical pair generates TEA and the enol-type biradical intermediate II, which undergoes coupling, tautomerization and then hydrogen shift to eventually afford the triazole-substituted dihydrobenzoquinolinone derivative 3. It has also previously been suggested that the naphthylmethylene anion radical formed by ET from TEA to N-benzoyl-α-dehydronaphthylalanine alkyl esters may reduce intramolecularly the N-benzoyl chromophore to greatly enhance the nucleophilicity of the amide carbonyl oxygen.3c This suggestion renders the assumption of the anion radical intermediate (E)-IB reasonable. Homolytic cleavage of the C(C=O)–N bond, hydrogen shift and the subsequent cyclization in (E)-IB give the radical intermediate III, triazolate anion and TEA cation radical. The process that reaches the benzoquinolinone and 1,2,4-triazole derivatives 2 and 4 is completed by back ET from III to the cation radical followed by proton transfer to the triazolate anion. On the other hand, the excited-state (Z)-isomer should also readily accept an electron from TEA forming the anion radical intermediate (Z)-I but there existed negligible amounts of (Z)-I-derived products. It is thus very likely that this anion radical is deactivated to eventually revert to (Z)-1 and/or (E)-1.
In Table 2 are collected solvent and substituent effects on the conversion (photoreactivity) of the starting alaninamide 1, the selectivity of 2 and 3 and the composition ratio of 2 to 3. Inspection of the solvent effect in protic and aprotic solvents confirmed that while the photoreactivity of 1a is not much affected by either polarity or proton-donating ability, the selectivity of the benzoquinolinone derivative 2 is markedly influenced by these two solvent properties. Intriguingly, upon changing the protic solvent from 2-propanol to methanol containing 50 vol% water, the selectivity of 2 was increased by a factor of 2.3, and then a comparison of this selectivity estimated in methanol (76%) and acetonitrile (29%) of comparable polarity to each other substantiates that as compared to the polarity of solvent, its proton-donating ability plays a more important role in controlling the product selectivity (product composition ratio). These solvent effects on the selectivity can be explained on the basis of the effects on the equilibrium between the anion radical intermediates (E)-IA and (E)-IB shown in Scheme 3: an increase in the proton-donating ability stabilizes the latter intermediate to a more extent than the former through much stronger hydrogen bonds to the triazolyl anion radical in (E)-IB, resulting in a large shift of the above equilibrium to the IB side. The increased solvent polarity is considered to exert the same effect on this equilibrium reaction by accelerating the intramolecular ET to the triazole chromophore within the intermediate (E)-IA. The above-mentioned considerations of solvent effects on the product selectivity, therefore, led us to conclude that the relative composition of (E)-IA and (E)-IB in the equilibrium state determines this selectivity. If our conclusion is valid, the introduction of the substituent R1 enabling the stabilization of an unpaired electron in the triazole ring is predicted to increase the selectivity of 2 by shifting the equilibrium to the IB side. As seen from Table 2, the selectivity is increased in the order of R1= H < Me ≈ PhCH2 < 4-MeOC6H4 < Ph < 4-NCC6Η4, being consistent with our prediction and, hence, providing additional evidence for the equilibrium between the two anion radical intermediates.
While there are several synthetic studies aiming at the construction of a pharmaceutically useful quinolinone ring,8 convenient photochemical routes to quinolinone and its derivatives are scarcely known.9 In addition to 1-methyl-2(1H)-benzo[f]quinolinone (2a), 2(1H)- and 1-benzyl-2(1H)-benzo[f]quinolinones (2g and 2h) were also obtained in 63% and 77% selectivities, respectively (Table 2),
and, hence, it is possible to synthesize photochemically 2(1H)-benzo[f]quinolinone and its related derivatives in high yields by choosing methanol as a solvent and p-cyanophenyl or phenyl group as a substituent bonded to the triazole ring. The use of chromatography over silica gel enables the rapid separation of these derivatives from the reaction mixtures.
ACKNOWLEDGMENTS
This research was partially supported by a “Scientific Frontier Research Project” from the Ministry of Education, Sports, Culture, Science and Technology, Japan.
* This paper is dedicated to Professor Emeritus Keiichiro Fukumoto on the occasion of his 75th birthday.
References
1. a) ‘Synthetic Organic Photochemistry,’ ed. by W. M. Horspool, Plenum, New York, 1984; b) ‘Handbook of Photochemistry and Photobiology,’ Vol. 2, ed. by H. S. Nalwa, American Scientific Publishers, Stevenson Ranch, 2003; c) ‘Synthetic Organic Photochemistry,’ ed. by A. G. Griesbeck and J. Mattay, Marcel Dekker, New York, 2005; d) A. G. Griesbeck, N. Hoffmann, and K. Warzecha, Acc. Chem. Res., 2007, 40, 128. CrossRef
2. F. D. Lewis and G. D. Reddy, J. Am. Chem. Soc., 1989, 111, 6465; CrossRef F. D. Lewis, G. D. Reddy, and D. M. Bassani, J. Am. Chem. Soc., 1993, 115, 6468; CrossRef F. D. Lewis, D. M. Bassani, and G. D. Reddy, J. Org. Chem., 1993, 58, 6390; CrossRef F. D. Lewis, G. D. Reddy, D. M. Bassani, S. Schneider, and M. Gahr, J. Am. Chem. Soc., 1994, 116, 597; CrossRef F. D. Lewis, D. M. Bassani, E. L. Burch, B. E. Cohen, J. A. Engleman, G. D. Reddy, S. Schneider, W. Jaeger, P. Gedeck, and M. Gahr, J. Am. Chem. Soc., 1995, 117, 660. CrossRef
3. K. Maekawa, T. Igarashi, K. Kubo, and T. Sakurai, Tetrahedron, 2001, 57, 5515; CrossRef K. Maekawa, A. Shinozuka, M. Naito, T. Igarashi, and T. Sakurai, Tetrahedron, 2004, 60, 10293; CrossRef K. Maekawa, T. Sasaki, K. Kubo, T. Igarashi, and T. Sakurai, Tetrahedron Lett., 2004, 45, 3663. CrossRef
4. K. Maekawa, Y. Kanno, K. Kubo, T. Igarashi, and T. Sakurai, Heterocycles, 2004, 63, 1273; CrossRef K. Maekawa, A. Tomoda, T. Igarashi, and T. Sakurai, Heterocycles, in press. CrossRef
5. R. A. Abramovitch, J. M. Beckert, H. H. Gibson, Jr., A. Belcher, G. Hundt, T. Sierra, S. Olivella, W. T. Pennington, and A. Sole, J. Org. Chem., 2001, 66, 1242; CrossRef M. J. Paterson, M. A. Robb, L. Blancafort, and A. D. DeBellis, J. Am. Chem. Soc., 2004, 126, 2912. CrossRef
6. Selected data for (Z)-1a: mp 279.0-280.0 °C (EtOAc-EtOH); IR (KBr) ν /cm-1 = 3215, 1668, 1629, 1544; 1H NMR (600 MHz, DMSO-d6) δ = 1.97 (6H, s), 2.79 (3H, d, J = 4.8 Hz), 6.67 (1H, d, J = 6.9 Hz), 7.35 (1H, dd, J = 6.9, 8.2 Hz), 7.62 (1H, dd, J = 7.6, 7.6 Hz), 7.66 (1H, dd, J = 7.6, 8.2 Hz), 7.94 (1H, d, J = 8.2 Hz), 7.99 (1H, d, J = 7.6 Hz), 8.18 (1H, d, J = 8.2 Hz), 8.41 (1H, s), 8.54 (1H, q, J = 4.8 Hz); 13C NMR (150 MHz, DMSO-d6) δ = 10.4 (2C), 26.5, 123.6, 125.5, 125.6, 126.6, 127.4, 127.9, 128.7, 128.8, 130.2, 130.9, 132.8, 133.0, 150.4 (2C), 162.8. Anal. Calcd for C18H18N3O: C, 70.57; H, 5.92; N, 18.29. Found: C, 70.23; H, 5.80; N, 17.96.
7. Selected data for 2a: mp 174.0-174.5 °C (EtOAc-hexane) (lit.,10: 182 °C); IR (KBr) ν /cm-1 = 1647; 1H NMR (600MHz, DMSO-d6) δ = 3.77 (3H, s), 6.77 (1H, d, J = 9.7 Hz), 7.57 (1H, dd, J= 6.9, 8.0 Hz), 7.70 (1H, dd, J= 6.9, 6.9 Hz), 7.80 (1H, d, J= 9.2 Hz), 8.01 (1H, d, J= 6.9 Hz), 8.16 (1H, d, J= 9.2 Hz), 8.57 (1H, d, J= 8.0 Hz), 8.81 (1H, d, J= 9.7 Hz); 13C NMR (125 MHz, DMSO-d6) δ = 29.7, 113.7, 115.4, 120.1, 121.9, 125.2, 128.0, 128.4, 128.5, 129.4, 131.8, 134.4, 139.0, 161.0. Anal. Calcd for C14H11NO: C, 80.36; H, 5.30; N, 6.69. Found: C, 80.49; H, 5.43; N, 6.91.
Selected data for 3a: mp 200.5-201.5 °C (EtOAc-hexane); IR (KBr) ν /cm-1 = 1676; 1H NMR (600 MHz, DMSO-d6) δ = 2.34 (6H, s), 3.47 (3H, s), 3.59 (1H, dd, J = 15.1, 15.8 Hz), 3.95 (1H, dd, J = 6.9, 15.1 Hz), 5.39 (1H, dd, J = 6.9, 15.8 Hz), 7.47 (1H, dd, J = 6.9, 9.0 Hz), 7.54-7.58 (2H, m), 7.94 (1H, d, J = 8.2 Hz), 7.98 (1H, d, J = 9.0 Hz), 8.05 (1H, d, J = 8.2 Hz); 13C NMR (125 MHz, DMSO-d6) δ = 11.3, 26.1, 30.6 (2C), 53.0, 116.0, 117.0, 123.5, 124.7, 126.9, 128.1, 128.2, 129.6, 130.5, 136.7, 151.0 (2C), 165.6. Anal. Calcd for C18H18N4O: C, 70.57; H, 5.92; N, 18.29. Found: C, 70.55; H, 5.86; N, 18.17.
Selected data for 4a: mp 124.0-125.0 °C (EtOH-hexane) (lit.,11: 143 °C); IR (KBr) ν /cm-1= 2926, 1717; 1H NMR (600 MHz, DMSO-d6) δ = 2.21 (6H, s), 13.1 (1H, s); 13C NMR (150 MHz, DMSO-d6) δ = 12.5 (2C), 155.6 (2C). Anal. Calcd (found) for C4H7N3: C, 49.47; H, 7.26; N, 43.27. Found: C, 49.52; H, 7.27; N, 42.93.
8. O. Meth-Cohn, Heterocycles, 1993, 35, 539; CrossRef C. W. Holzapfel and C. Dwyer, Heterocycles, 1998, 48, 215; CrossRef K. Y. Lee and J. N. Kim, Bull. Korean Chem. Soc., 2002, 23, 939; CrossRef B. Joseph, F. Darro, A. Behard, B. Lesur, F. Collignon, C. Decaestecker, A. Frydman, G. Guillaumet, and R. Kiss, J. Med. Chem., 2002, 45, 2543; CrossRef D. V. Kadnikov and R. C. Larock, J. Organomet. Chem., 2003, 687, 425; CrossRef M. Hadjeri, C. Beney, and A. Boumendjel, Curr. Org. Chem., 2003, 7, 679; CrossRef S. K. Chattopadhyay, R. Dey, and S. Biswas, Synthesis, 2005, 403; CrossRef Q. Li, K. W. Woods, W. Wang, N.-H. Lin, A. Claiborne, W.-Z. Gu, J. Cohen, V. S. Stoll, C. Hutchins, D. Frost, S. H. Rosenberg, and H. L. Sham, Bioorg. Med. Chem. Lett., 2005, 15, 2033; CrossRef M. Asahara, M. Ohtsutsumi, M. Tamura, N. Nishiwaki, and M. Ariga, Bull. Chem. Soc. Jpn., 2005, 78, 2235; CrossRef R. Bernini, S. Cacchi, G. Fabrizi, and A. Sferrazza, Heterocycles, 2006, 69, 99; CrossRef R. Bernini, S. Cacchi, I. De Salve, and G. Fabrizi, Synlett., 2006, 2947. CrossRef
9. T. Horaguchi, N. Hosokawa, K. Tanemura, and T. Suzuki, J. Heterocycl. Chem., 2002, 39, 61. CrossRef
10. T. Hase, Acta Chem. Scand., 1964, 18, 1806. CrossRef
11. T. Kauffmann, L. Ban, and D. Kuhlmann, Chem. Ber., 1981, 114, 3684 CrossRef