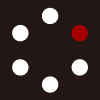
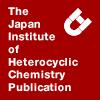
HETEROCYCLES
An International Journal for Reviews and Communications in Heterocyclic ChemistryWeb Edition ISSN: 1881-0942
Published online by The Japan Institute of Heterocyclic Chemistry
e-Journal
Full Text HTML
Received, 30th July, 2008, Accepted, 12th September, 2008, Published online, 18th September, 2008.
DOI: 10.3987/REV-08-SR(F)4
■ Production of Unnatural Bioactive Glycosides Using Plant Secondary Product Glycosyltransferases
Sayaka Masada and Hajime Mizukami*
Graduate School of Pharmaceutical Sciences, Nagoya City University, 3-1 Tanabe-dori, Mizuho-ku, Nagoya 467-8603, Japan
Abstract
Plant glycosyltransferases mediate transfer of a glycosyl residue from activated nucleotide sugars to lipophilic small molecules, thus affecting the solubility, stability and pharmacological activities of the compounds. Biotechnological application of the plant glycosyltransferases in glycoside synthesis has attracted attention recently. This review describes the in vivo and in vitro glycosylation of natural organic compounds using the glycosyltransferases, focusing on our current investigation for enzymatic synthesis of curcumin glycosides.NTRODUCTION
Higher plants accumulate a wide array of structurally diversified secondary metabolites, many of which have been used as pharmaceuticals, pigments and flavors. The structural diversity arises not only from the variation in basic structures but also from the modifications of core structures by methylation, acylation, hydroxylation, glycosylation, etc.
The glycosylation reactions are catalyzed by so called plant secondary product glycosyltransferases (PSPGs), which transfer sugar moieties from UDP-activated sugar donors to low molecular weight acceptors. PSPGs can recognize a wide range of acceptor substrates, not only endogenous metabolic intermediates but also xenobiotics, and can add a broad range of different carbohydrate moieties by employing all forms of sugars, independently (monoglycosides), in parallel or in chains (di-, tri-glycosides). This gives rise to a broad spectrum of glycosidic structures for any given aglycone. For example, out of a total of 5,000 different flavonoid aglycone, 300 different glycosides of one single quercetin, have already been identified.1 Glycosylation of low molecular weight compounds has a wide range of effects on their physicochemical and physiological properties, including increased water solubility, improved chemical stability, reduced chemical toxicity and altered biological activity.2 Chemical synthesis of glycosides is usually difficult because it involves multiple blocking/deblocking steps before any product can be obtained. Enzymatic glycosylation offers several advantages over chemical methods, including (1) avoiding use of harsh conditions and toxic catalysts, (2) providing strict control of regio- and stereo-selectivity and (3) high efficiency.3 Thus, PSPGs have been attracting considerable interest as general biocatalysts in glycoside synthesis.4
Curcumin (diferuloylmethane, 1) is a water-insoluble yellow pigment of turmeric (dried rhizome of Curcuma longa, Zingiberaceae). It has been used primarily as a food colorant, but is potentially a neutraceutical because of its interesting pharmaceutical properties, including anti-oxidative, anti-inflammatory, anti-angiogenesis, anti-leishmanial and anti-tumor activities, and its potential as a therapeutic agent in Alzheimer’s disease, Parkinson disease, cardiovascular disease, pulmonary disease, and arthritis.5 However, its low water solubility has been limiting further pharmacological exploration and practical application.
Here we review our current research on the enzymatic synthesis of curcumin glycosides using Catharanthus roseus cell suspension cultures as well as recombinant PSPGs whose corresponding cDNA was isolated from cultured C. roseus cells.
1. Production of unnatural curcumin glycosides by cultured plant cells
1.1 Screening for the curcumin glycosylation ability in various plant cell cultures
Glycosylation of various organic molecules by cultured plant cells has been investigated as one of the targets in biotechonological application of plant cell culture systems. It has been reported that various aromatic compounds supplied to cell suspension cultures can be efficiently converted to their glycosides, including hydroquinone,6 vanillin,7 capsaicin,8 and isoflavones9 on species-dependent manner.
We screened suspension cultures of 10 different plant species for their ability to glycosylate curcumin; Bupleurum falcatum, Catharanthus roseus, Daucus carota, Duboisia hybrid, Gardenia jasminoides, Glehnia littoralis, Ipomoea batatas, Lithospermum erythrorhizon, Nicotiana tabacum, and Papaver somniferum. TLC analysis of the extracts from the cells and medium indicated that only C. roseus cells were capable of converting exogenously supplied curcumin to novel products with higher polarities, which were subjected to further isolation and structure identification.
1.2 Identification of curcumin glucosides produced in C. roseus cell suspension cultures
At least five potentially glycosylated products were detected when the cell extract of C. roseus cells supplied with curcumin was analyzed by HPLC. These products were isolated by preparative HPLC and their chemical structures were elucidated based on the MS and NMR spectra as curcumin 4’-O-β-D-glucopyranoside (2), curcumin 4’-O-β-D-gentiobioside (3), curcumin 4’,4”-O-β-D-diglucoside (4), curcumin 4’-O-β-D-gentiobiosyl 4”-O-β-D-glucopyranoside (5), and curcumin 4’,4”-O-β-D-digentiobioside (6).10 None of these glucosides has been found in nature so far.
There have been many reports on glucosylation of exogeneously supplied phenolic compounds in cultured plant cells since Pilgrim first described glucosylation of some simple phenols, including hydroquinone, in callus cultures of three plant species.11 In most cases exogenous compounds with two hydroxyl groups were shown to be converted to their monoglucosides: hydroquinone to arbutin,12 salicyl alcohol to salicin and isosalicin,13 esculetin to esculin,14 and salicylic acid to salicylic acid O-glucoside.15 Futhermore, camphor was shown to be transformed to its monoglucosides at 3-, 5-, 6- or 8-position by Eucalyptus perriniana cultured cells after the introduction of hydroxyl groups into these positions but no diglucoside was formed. In contrast to these results, C. roseus cells in culture converted curcumin to various glycosides not only by introducing individual glucose residues onto its two phenolic hydroxyl groups but also by forming β-1,6-glycosidic linkages, leading to the gentiobioside derivatives.
1.3 Water solubility and antioxidative activity of curcumin glucosides
The water solubility of curcumin glycosides was estimated and compared with that of 1 (Table 1). Although the water solubility of 2 was increased only 230-fold, the solubility was increased about 2 million-fold and about 20 million-fold in the case of 3 and 6, respectively, compared with the solubility of 1. The result indicated that conjugation of at least two glucose residues to curcumin enhanced the water solubility dramatically. As for solubilization of curcumin, two different approaches, conjugation with cyclodextrins17 and nano-particle formation18 have been reported so far. Compared to these methods, the increase in solubility by the glucosyl conjugation was found to be quite drastic.
The antioxidative activity of curcumin glycosides was determined by the DPPH radical scavenging method (Table 1). The antioxidative activity of 2 and 3 was half of that of 1, and the activity was lost in the case of 4, 5 and 6 because of their lack of a phenolic hydroxyl group.
1.4 Optimization of glucosylation activity of C. roseus cell cultures
To optimize the curcumin glycosides productivity of C. roseus cell cultures, we evaluated the effect of culture stage of the cells, medium sucrose concentration, and elicitor addition on glucosylation activity of the cells. The glycoside yield reached 32% when 15 μmol curcumin was added to the 30 mL cell suspension in the presence of 250 µM methyl jasmonate and 8% sucrose on day 4 of the culture.10 However, the yield is still low, and additional extraction and isolation steps are necessary because the cells accumulate glycosides in their vacuoles, and it is, therefore, desirable to establish in vitro systems for enzymatic production of curcumin glycosides using recombinant enzymes.
2. Enzymatic synthesis of curcumin glucosides with recombinant glucosyltransferases
2.1 Molecular cloning and characterization of a curcumin glucosyltransferase from C. roseus.
PSPGs comprise a super family of plant genes similar in magnitude to the O-methyltransferase19 and cytochrome P45020 gene families. These enzyme classes are involved in modification of secondary metabolites, thus contributing to the diversity of plant chemistry. PSPGs are defined by the presence of a 44 amino acid C-terminal signature motif designated as the PSPG–box.21 Within the PSPG-box, a peptide sequence of HCGWNS has been detected in 95% of all the PSPGs that catalyze inversion of the anomeric bond from the α-linkage found in UDP-sugar to the β-configuration found in the resulting glycosides.22 Furthermore, investigation on the 3D-structures of isoflavnoid 3’-O-glucosyltransferase and (iso)flavnoid 3,7-O-glucosyltransferase from Medicago truncatula,23 anthocyanidin 3-O-glucosyltransferase from Vitis vinifera,24 and bifunctional N- and O-glucosyltransferase from Arabidopsis thaliana25 by X-ray crystallography revealed the role of specific conserved amino acids residues in the PSPG-box as the donor-sugar binding pocket.
We designed a degenerate primer based on the amino acid sequence F(L/V)(T/S)HCGWN in this motif and isolated two cDNAs (UCaUtharanthus roseus UUUDP-glucose UgUlucosylUtUransferase, CaUGT1 and CaUGT2) from a cDNA library of cultured C. roseus cells, using a homology-based PCR cloning strategy.26 To clarify the enzymatic properties of CaUGT1 and CaUGT2, both cDNAs were expressed in Escherichia coli as His6-tag fusion proteins and the purified enzyme was used for enzyme activity assays using either curcumin (1) or curcumin monoglucoside (2) as an acceptor substrate in the presence of UDP-glucose. As shown in Figure 2, CaUGT2 converted both 1 and 2 to 2 and curcumin diglucoside (4), respectively.
The time course pattern of the glucosylation reaction with CaUGT2 indicated that 1 was first converted to 2 which was then further glucosylated to 4 by the single enzyme of the recombinant CaUGT2. In contrast, no products were detected when the recombinant CaUGT1 was incubated with either 1 or 2. These results clearly indicate that CaUGT2 is defined as UDP-glucose: curcumin glucosyltransferase (UCGT).
2.2 Enzymatic vs. chemical synthesis of curcumin glucosides
There have been some reports describing chemical synthesis of curcumin glucosides. Direct glucosylation of 1 by acetobromoglucose followed by deacetylation gave 2 and 4 at yields of 8% and 3%, respectively.27 A total synthesis approach starting from vanillin gave 2 and 4 with overall yields of 14% and 20%, respectively.28
In sharp contrast to these chemical syntheses, the glucosylation yield reached up to 89% (2, 36%; 4, 53%) after 48h incubation in a total volume of 500 mL containing 0.85 mmole 1, 2.5 mmole UDP-glucose, and crude enzyme preparation containing 500 mg bacterial protein. Furthermore, the enzymatic method is simple and less laborious compared with the chemical methods because it requires neither blocking/deblocking steps nor intermediates purification at each reaction step.
2.3. One-pot two-enzyme system for efficient chemoenzymatic synthesis of curcumin glucosides
Although enzymatic synthesis of curcumin glucosides using the recombinant CaUGT2 is efficient, the process still requires that a sugar donor such as UDP-glucose, which is usually expensive and difficult to obtain in large quantities, be supplied in the reaction mix. In addition, UDP, one of the products of the enzymatic glucosylation reaction, is a potent inhibitor of PSPGs and the reaction rate gradually declines as UDP accumulates in the reaction mixture.
To alleviate these problems, enzymatic methods that allow in situ regeneration of UDP-sugars are quite attractive because this strategy not only reduces the cost of supplying the UDP-sugar substrate, but also avoids product inhibition by UDP.29 Therefore, we established such an in situ system for regeneration of UDP-glucose catalyzed by a recombinant Arabidopsis sucrose synthase (AtSUS1) combined with in vitro glucosylation of curcumin by the recombinant CaUGT2.30 In this one-pot two-enzyme system glucosyl transfer from UDP-glucose to curcumin by the CaUGT2 is coupled with removal of UDP and regeneration of UDP-glucose by the recombinant AtSUS1 as shown in Figure 3.
Sucrose synthase (SUS; E.C. 2.4.2.13.) is capable of catalyzing both synthesis and cleavage of sucrose. Although the reaction is reversible, its biological function has been considered to be synthesis of nucleotide diphosphate derivatives of glucose in the presence of higher concentrations of sucrose.31 We cloned the coding region of AtSUS1 cDNA from A. thaliana by RT-PCR based on the genomic sequence, and obtained the recombinant AtSUS1 protein as a His6-fusion protein. When we incubated 1 with UDP-glucose and recombinant CaUGT2, 2 initially accumulated followed by slower accumulation of 4. After 2 hr incubation, about 20% of the initial curcumin was converted to a mixture of the two glucosides (Figure 4A). In sharp contrast to this pattern, when AtSUS1 and sucrose were included in the glycosylation reaction mixture, 4 was rapidly accumulated, and after 60 min incubation, neither 1 nor 2 could be detected in the reaction mixture (Figure 4B).
The final amount of glucosides formed in a reaction mixture including 0.2 mM UDP-glucose plus AtSUS1 and sucrose was similar to that produced at 2 mM UDP-glucose without AtSUS1. In effect, addition of recombinant AtSUS1 to the reaction mixture allowed the initial concentration of UDP-glucose substrate to be reduced to one-tenth of that required in the absence of AtSUS1. Furthermore, from AtSUS1-supplemented incubations supplied with 0.2 mM UDP-glucose, 0.7 mM 2 and 0.8 mM 4 were produced. Since 4 contains two molecules of glucose, the above result indicates that 0.23 mM glucose had been converted into glucosides from an initial supply of 0.2 mM UDP-glucose, confirming that UDP-glucose was actively being regenerated for utilization as a glucose donor. We finally indicated that the present one-pot two-enzyme system linking UDP-glucose regeneration with PSPGs is generally applicable to enzymatic glucosylation of lipophilic small molecules by showing drastic increase in apigenin 7-O-glucoside synthesis from apigenin with Scuterallia baicalensis flavonoid 7-O-glucosyltransferase (F7GT)32 when AtSUS1 and sucrose were included in the reaction mixture.
3. Future approaches
3.1 Isolation of the second glucosyltransferase
Curcumin gentiobioside (3) is a potentially useful compound because its water solubility is dramatically increased while retaining the antioxidative activity, compared with curcumin (1). For enzymatic synthesis of 3 from 1, besides CaUGT2 the second enzyme, UDP-glucose: curcumin glucoside 1→6 glucosyltransferase (UCGGT), catalyzing glucosylation of the 6”-hydroxy group of the glucose moiety of curcumin monoglucoside (2) is necessary (Figure 5). In general, many reports have described cloning and molecular characterization of PSPGs transferring a sugar moiety to free hydroxyl or carboxyl groups of aglycones whereas cDNA isolation of PSPGs attaching additional sugar groups to an existing sugar residue of the particular glycosides have been quite limited and is, therefore, challenging.
Since neither CaUGT1 nor CaUGT2 exhibited the UCGGT activity and we could not obtain a cDNA clone encoding UCGGT by the homology-based approach, we are now trying to isolate the cDNA based on the partial amino acid sequence of the UCGGT. Towards this end we are so far successful to purify the UCGGT to apparent homogeneity from C. roseus cell suspention cultures.33
3.2 Establishing the in vivo glucosylation system
Although the one-pot two-enzyme system using PSPGs plus AtSUS1 is effective for enzymatic glucosylation of small molecules, it still requires UDP-glucose as a sugar donor and costly for large scale synthesis of glucosides. Microorganisms are well known to be simple and efficient biocatalysts and have a set biosynthetic enzymes leading to endogeneous UDP-sugar production. In fact, E. coli transformed with an Arabidopsis glycosyltransferase was used for regioselective glucosylation of quecetin.34 Thus, the recombinant E. coli cells simultaneously expressing CaUGT2 and ATSUS1 for production of 2 and 4, or CaUGT2, UCGGT cDNA (though not isolated yet) and AtSUS1 for production of 3 can be promising biocatalysts in synthesis of curcumin glucosides.
This paper is dedicated to Professor Emeritus Keiihiro Fukumoto on the occasion of his 75th birthday.
References
1. L. Packer, ‘Flavonoids and other polyphenols’, Academic Press, Inc., Tokyo, 2001.
2. P. Jones and T. Vogt, Planta, 2001, 213, 164. CrossRef
3. V. Kren and J. Thiem, Chem. Soc. Rev., 1997, 26, 463. CrossRef
4. E. K. Lim, Chem. Eur. J., 2005, 11, 5486. CrossRef
5. A. Goel, A. B. Kunnumakkara, and B. B. Aqqarwal, Biochem. Pharmacol., 2008, 75, 787. CrossRef
6. S. Inomata, M. Yokoyama, S. Seto, and M. Yanagi, Appl. Microbiol. Biotechnol., 1991, 36, 315. CrossRef
7. T. Kometani, H. Tnimoto, T. Nishimura, and S. Okada, Biosci. Biotechnol. Biochem., 1993, 57, 1290.
8. T. Kometani, H. Tnimoto, T. Nishimura, I. Kanbara, and S. Okada, Biosci. Biotechnol. Biochem., 1993, 57, 2192; CrossRef K. Shimoda, S. Kwon, A. Utsuki, S. Ohiwa, H. Katsuragi, N. Yonemoto, H. Hamada, and H. Hamada, Phytochemistry, 2007, 68, 1391. CrossRef
9. D. Li, J. H. Park, J. T. Park, C. S. Park, and K. H. Park, J. Agric. Food Chem., 2004, 52, 2561; CrossRef D. Li, S. A. Roh, J. H. Shim, B. Mikami, M. Y. Baik, C. S. Park, and K. H. Park, J. Agric. Food Chem., 2005, 53, 6516. CrossRef
10. Y. Kaminaga, A. Nagatsu, T. Akiyama, N. Sugimoto, T. Yamazaki, T. Maitani, and H. Mizukami, FEBS Lett., 2003, 555, 311. CrossRef
11. H. Pligrim, Pharmazie, 1970, 25, 568.
12. M. Tabata, F. Ikeda, N. Hiraoka, and M. Konoshima, Phytochemistry, 1976, 12, 1225. CrossRef
13. H. Mizukami, T. Terao, H. Miura, and H. Ohashi, Phytochemistry, 1983, 22, 679. CrossRef
14. M. Tabata, Y. Umetani, K. Shima, and S. Tanaka, Plant Cell Tissue Organ Culture, 1984, 3, 3. CrossRef
15. Y. Umetani, E. Kodakari, T. Yamamura, S. Tanaka, and M. Tabata, Plant Cell Rep., 1994, 35, 941.
16. Y. Orihara, T. Noguchi, and T. Furuya, Phytochemistry, 1994, 35, 941. CrossRef
17. H. H. Tønnessen, M. MÁsson, and T. Loftsson, Int. J. Pharm., 2002, 244, 127.
18. S. Bisht, G. Feldmann, S. Soni, R. Ravi, C. Karikar, A. Maitra, and A. Maitra, J. Nanobiotechnol., 2007, 5, 3. CrossRef
19. R. K. Ibrahim, Trends Plant Sci., 1997, 2, 249. CrossRef
20. C. Chapple, Annu. Rev. Plant Physiol. Plant Mol. Biol., 1998, 49, 311. CrossRef
21. J. Hughes and M. A. Hughes, DNA Seq., 1994, 5,41. CrossRef
22. D. Kapitonov and R. K. Yu, Glycobiology, 1999, 9, 961; CrossRef T. Vogt and P. Jones, Trends Plant Sci., 2000, 5,380. CrossRef
23. H. Shao, X. He, L. Achnine, J. W. Blount, R. A. Dixon, and X. Wang, Plant Cell, 2005, 17,3141; CrossRef X. Z. He, X. Wang, and R. A. Dixon, J. Biol. Chem., 2006, 281, 34441; CrossRef L. Li, L. V. Modolo, L. L. Escamilla-Trevino, L. Achnine, R. A. Dixon, and X. Wang, J. Mol. Biol., 2007, 370, 951. CrossRef
24. W. Offen, C. Martinez-Fleites, M. Yang, E. K. Lim, B. G. Davis, C. A. Tarling, C. M. Ford, D. J. Bowles, and G. J. Davies, EMBO J., 2006, 25, 1396. CrossRef
25. M. Brazier-Hicks, W. A. Offen, M. C. Gershater, T. J. Revett, E. K. Lim, D. J. Bowles, G. J. Davies, and R. Edwards, PNAS, 2007, 104, 51. CrossRef
26. Y. Kaminaga, F. P. Sahin, and H. Mizukami, FEBS Lett., 2004, 567, 197. CrossRef
27. M. Hergenhahn, German patent application, 2002, DE 2337.
28. K. Mohri, Y. Watanabe, Y. Yoshida, M. Satoh, K. Isobe, N. Suqimoto, and Y. Tsuda, Chem. Pharm. Bull., 2003, 51, 1268. CrossRef
29. Y. Ichikawa, R. Wang, and C. H. Wong, Meth. Enzymol., 1994, 247, 107. CrossRef
30. S. Masada, Y. Kawase, M. Nagatoshi, Y. Oguchi, K. Terasaka, and H. Mizukami, FEBS Lett., 2007, 581, 2562. CrossRef
31. B. B. Buchanan, W. J. Gruissem, and R. L. Jones, ‘Biochemistry & Molecular Biology of Plants’, American Society of Plant Physiologists, Rockville, 2000, pp. 646-648.
32. M. Hirotani, R. Kuroda, H. Suzuki, and T. Yoshikawa, Planta, 2000, 210, 1006.
33. Y. Oguchi, S. Masada, T. Kondo, K. Terasaka, and H. Mizukami, Plant Cell Physiol., 2007, 48, 1635. CrossRef
34. E. K. Lim, D. A. Ashford, B. Hou, R. G. Jackson, and D. J. Bowles, Biotechnol. Bioeng., 2004, 87, 623 CrossRef