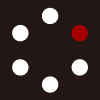
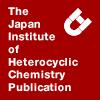
HETEROCYCLES
An International Journal for Reviews and Communications in Heterocyclic ChemistryWeb Edition ISSN: 1881-0942
Published online by The Japan Institute of Heterocyclic Chemistry
e-Journal
Full Text HTML
Received, 30th September, 2008, Accepted, 8th December, 2008, Published online, 12th December, 2008.
DOI: 10.3987/REV-08-SR(D)4
■ Reactions and Uses of Artificial Ketoses
Takashi Yamanoi* and Sho Matsuda
The Noguchi Institute, 1-8-1, Kaga, Itabashi-ku, Tokyo 173-0003, Japan
Abstract
Some artificial ketoses having a naturally occurring aldose backbone can be readily prepared by the addition of RLi or RMgX to aldonolactone derivatives. They are expected to be a novel class of carbohydrate reagents for synthesizing valuable compounds. In order to utilize these ketoses completely, we must elucidate the reaction characteristics influenced by the ketose’s specific structures. In particular, it is important to understand the reaction specificities of the nucleophilic substitutions at the anomeric carbons of these ketoses to produce various ketosides. This review describes the nucleophilic reactions to form the ketosidic linkages from the artificial ketoses, focusing mainly on our recent research results.1. INTRODUCTION
Carbohydrates play important roles in biological processes.1 They are also chemically useful materials.2 Non-natural carbohydrates derived by chemical modifications of natural carbohydrates are frequently utilized as reagents for preparing valuable compounds in synthetic organic chemistry.3
The addition reactions of RLi (or RMgX) to sugarlactone derivatives produced by oxidation of aldoses can readily produce various artificial ketoses whose anomeric carbons are bound to alkyl, alkynyl, alkene, aryl or other groups via a carbon–carbon linkage.4 The ketoses prepared by this method from naturally occurring aldoses posses the ring structures of the starting aldoses. Such ketoses, regarded as aldose analogues, are often used as precursors for preparing C-glycosides by reduction procedures.4 Recent studies show that some useful compounds, such as enzyme inhibitors,5 oligosaccharide mimics6 and spiroketals7 were prepared from these ketoses. These compounds are expected to form a new class of carbohydrate reagents useful for synthesizing more valuable and complicated compounds.
In order to utilize these ketoses completely, we must elucidate the reaction characteristics influenced by the ketose’s specific structures. In particular, it is important to understand the specificities of the reactions to produce various ketosides from these ketoses by nucleophilic substitutions at the anomeric carbons of the ketoses. Since their anomeric carbon atoms are bound directly to functional groups, steric and electron effects of the functional groups easily influence the anomeric carbons. These factors make the reaction behaviour of the nucleophilic substitutions at the anomeric carbons of these ketoses remarkably different from those of aldoses.
We have devoted our considerable effort to investigating nucleophilic reactions at the anomeric carbons of artificial ketoses having a naturally occurring aldohexopyranose backbone.8 As a result, we successfully elucidated their nucleophilic reaction specificities and developed efficient synthetic methods for several types of ketopyranosides. In addition, as shown in Scheme 1, the findings from these studies contributed to the development of novel synthetic approaches for valuable carbohydrate compounds such as exo-glycals,9 trehalose mimics10 and anhydroketopyranoses11 from these ketoses.
This paper summarizes our recent studies on nucleophilic substitutions to form ketopyranosidic linkages from artificial ketoses with a naturally occurring aldohexopyranose backbone as well as reviews the reports from other research groups.
(Nomenclature in this review)
For convenience, the artificial ketoses are defined as follows for easy understanding of the ketose’s ring conformation. The general ketose with a naturally occurring aldohexopyranose backbone is named as ‘1-C-modified hexopyranose’. For example, the ketose with a methyl group at the anomeric carbon and a D-glucopyranose backbone is named as ‘1-C-methyl-D-glucopyranose’. The location number of the anomeric carbon atoms of these ketoses is defined in the same manner as that of the aldose.
2. FORMATION OF THE KETOPYRANOSIDIC LINKAGES FROM 1-C-MODIFIED HEXOPYRANOSES
In this section, we summarize published reports on the formation reactions of ketopyranosidic linkages from 1-C-modified hexopyranoses, as shown in Scheme 2. Table 1 lists the ketose structures, nucleophiles and activators used, in addition to the reference numbers.
3. NUCLEOPHLIC SUBSTITUTIONS AT THE ANOMERIC CARBONS OF 1-C-MODIFIED HEXOPYRANOSE AND THEIR APPLICATIONS TO USEFUL COMPOUNDS
We have investigated nucleophilic reactions at the anomeric carbons of 1-C-modified hexopyranoses with various types of nucleophiles. As a result, the nucleophilic reaction specificities and development of efficient synthetic methods for various types of ketopyranosides were clarified.8 In addition, we succeeded in developing novel synthetic approaches to valuable carbohydrate compounds such as exo-glycals,9 trehalose mimics10 and anhydroketopyranoses11 from the 1-C-modified hexopyranoses. This section describes these results.
3.1 Formation of C- and N-ketopyranosidic linkages from 1-C-modified D-glucopyranose
In this section, we describe the C- and N-ketosidations of the 1-C-modified D-glucopyranose derivatives with several nucleophiles.8a,f Scheme 3 shows the utilized glucopyranose derivatives (1a–f) carrying the methyl, ethyl, n-butyl, allyl, benzyl and phenyl groups at the anomeric carbon centres as well as the ketopyranosides synthesized in the study. We used allyltrimethylsilane, trimethylsilyl azide (TMSN3), trimethylsilyl cyanide (TMSCN) and 1-phenyl-1-(trimethylsilyloxy)ethylene as the nucleophiles.
We first investigated C-allylation of 1a with allyltrimethylsilane in the presence of TMSOTf as the activator, as shown in Scheme 4. Although the reaction gave the desired C-allylated glucopyranoside 3a, the benzyl 1-C-methylated O-glucopyranoside 2a was also obtained as a major by-product. This was attributable to the benzyl alcohol produced with the degradation of 1a under the given reaction conditions. It acted as a glycosyl acceptor of 1a and afforded 2a. Then, we modified the reaction conditions such as the solvents, reaction temperatures and amounts of reagents. The reaction conditions using 3 equiv. of allyltrimethylsilane in MeCN at −40 °C in the presence of 20 mol% TMSOTf and the drying agent, CaSO4, successfully increased the yield of 3a up to 88% with almost no production of 2a. The reaction conditions were also effective for the C-allylation of 1b, 1c and 1f having an ethyl, an n-butyl or a phenyl group to afford the C-allylated glucopyranosides 3b, 3c and 3f, respectively, in the good yields of 88-95%. Under the given reaction conditions, however, C-allylation using 1d and 1e yielded the desired products 3d and 3e in only 31% and 18%. The reactions using 1d and 1e still produced the benzyl O-glucopyranosides 4d and 4e, as shown in Scheme 5, as the major by-products in ca. 20% yields, respectively. Apparently, the electronic or steric effect of the functional groups at the anomeric centers of 1d and 1e influenced the leaving ability of the hemiacetal hydroxy group and thereby the productions of 4d and 4e.
All the reactions of 1a–c and 1f with TMSN3, TMSCN and 1-phenyl-1-(trimethylsilyloxy)ethylene under the stated reaction conditions successfully produced the desired glucopyranosides 5a–c and 5f, 6a–c, and 7a–c and 7f in the high yields of 78-99%. In contrast, the yields obtained by ketosidation using 1d and 1e depended on the species of the nucleophiles. The reactions using TMSN3 afforded 5d and 5e both in 80% yield. The reactions using TMSCN gave 6d and 7d and those using 1-phenyl-1-(trimethylsilyloxy)ethylene gave 6e and 7e in moderate yields of 53–73%. The yields of the C- and N-ketosidations using 1d and 1e were lower than those using 1a–c and 1f.
Our next effort was directed towards improving the yields of the reactions of 1d and 1e with allyltrimethylsilane. Although the use of excess amounts of the reagents increased the yields of 3d and 3e to 53% and 78%, respectively, they were not satisfactory. In addition, the exo-glycal derivative 858 was obtained as a novel by-product in 16% yield by the reaction using 1d. Therefore, we attempted to use the glucopyranosyl acetates 9d and 9e. These acetates were expected to have high leaving abilities of the anomeric acetoxy group and were produced by the reactions of 1d and 1e with acetic anhydride using n-butyllithium in THF. The yield of 3e obtained by the reaction of 9e with allyltrimethylsilane increased up to 80% in the presence of 20 mol% TMSOTf in MeCN/CH2Cl2 (v/v = 1/1) at −78 °C. Under similar conditions, all the reactions of 9d and 9e with allyltrimethylsilane, TMSN3, TMSCN and 1-phenyl-1-(trimethylsilyloxy)ethylene produced the corresponding glucopyranosides 3d and 3e, 5d and 5e, 6d and 6e, and 7d and 7e, respectively, in excellent yields. These results are shown in Table 2.
All the ketopyranosides were produced as single isomers, and the measurement of their NMR spectra indicated the NOE interactions between the proton of H-1’ (or aromatic H) and H-3. The ketosidations proceeded with α-stereoselectivities. The α-side attack was advantageous for direct formation of the chair conformation from oxocarbenium cation intermediates, and forced the original anomeric functional groups of 1a-f to assume an equatorial orientation. These conformational changes would contribute to the stabilization of the formation of the 4C1 conformation, as shown in Scheme 6.
These C- and N-ketosidation methods developed by us are potentially useful for synthesizing the subunits of naturally occurring products and chiral intermediates in organic chemistry.
3.2 Formation of exo-glycals from 1-C-vinyl-hexopyranose derivatives
In this section, we describe our synthetic approach to exo-glycals18,20,68,69 from 1-C-vinyl-hexopyranose derivatives.9 We speculated that the 1-C-vinyl-hexopyranose derivatives were likely to generate the specific glycosyl oxocarbenium cation intermediates stabilized by their resonance forms and that this effect would allow the nucleophiles to attack not only the anomeric carbons as stated above but also the terminal olefinic carbons, as shown in Scheme 7. The latter nucleophilic substitution, i.e. the SN1’-type substitution was expected to produce the exo-glycal structures. All previously published reports, however, involved the SN1-type ketosidation using alcoholic nucleophiles to synthesize 1-C-vinyl-O-hexopyranosides from 1-C-vinyl-hexopyranose derivatives.7b,11a,b,12,17-23 We therefore anticipated that changing the species of the nucleophiles would make it possible to form exo-glycals from the 1-C-vinyl-hexopyranose derivatives via an SN1’-type substitution mechanism.
We investigated the reactions between 10 and several nucleophiles, such as TMSCN, TMSN3 and allyltrimethylsilane, using 20 mol% TMSOTf in MeCN at −40 °C, as shown in Scheme 8. The reaction using TMSCN gave only the ketopyranoside 11 in 88% yield by the SN1-type substitution. When TMSN3 and allyltrimethylsilane were used, the desired (Z)-exo-glycals 12 and 13 were stereoselectively obtained in 64% and 39% yields, respectively. Although the reaction using TMSN3 gave 12 along with the ketopyranoside 14 in 16% yield, the reaction using allyltrimethylsilane produced 13 as a single product. We found that the differences in the species of the nucleophiles markedly influenced the nucleophilic characteristics of the reaction systems. Furthermore, the yield of 13 increased to 58% under reaction conditions using 20 mol% TMSOTf in MeCN/CH2Cl2 (v/v = 1/1) at −78 °C. Table 3 lists these results.
Next, we investigated the synthesis of various exo-glycal derivatives from the 1-C-vinyl-hexopyranoses 10, 18, 21 and 22 using several types of trimethylsilyl enol ethers, which were π-electron-related nucleophiles similar to allyltrimethylsilane. For the trimethylsilyl enol ethers, we used 1-phenyl-1-(trimethylsilyloxy)ethylene, 2-methyl-1-trimethylsilyloxy-1-propene, and 3,3-dimethyl-2-trimethylsilyloxy-1-butene. Under the stated reaction conditions, the reactions between 10 and the trimethylsilyl enol ethers afforded the corresponding (Z)-exo-glycals 15–17 by SN1’-type substitution in good yields from 61% to 74% with no production of the corresponding ketopyranosides. The reactions of the 1-C-1-propenyl-glucopyranose 18 with allyltrimethylsilane or 1-phenyl-1-(trimethylsilyloxy)ethylene also produced the corresponding (Z)-exo-glycal 19 or 20 in 68% or 79% yield with a diastereomer ratio of 3.5/1 or 2/1, respectively. The reactions of the 1-C-vinyl-mannopyranose 21 with 1-phenyl-1-(trimethylsilyloxy)ethylene gave the corresponding exo-glycal 23 in a high yield of 94% with a geometric isomer ratio of Z: E = 91: 9. The steric effect of the C-2 benzyloxy group slightly influenced the reaction stereoselectivity. The reactions between the 1-C-vinyl-galactopyranose 22 with allyltrimethylsilane stereoselectively afforded the (Z)-exo-glycal 24 in 76% yield. We observed the NOE interactions between H-2 and H-1’ of the exo-glycals, as shown in Scheme 9, and determined their geometric isomers as the Z forms.
To the best of our knowledge, this is the first synthetic approach to exo-glycals from the 1-C-vinyl-hexopyranoses by the SN1’-type substitution mechanism. This method can provide various types of functionalized exo-glycals useful as synthetic intermediates in carbohydrate chemistry.
3.3 Formation of O-ketopyranosidic linkages from 1-C-modified D-glucopyranoses
1-C-Modified O-glycosides are generally synthesized by glycosidations using 1-methylene sugars (exo-glycals) or 1-C-modified sugars as glycosyl donors. 1-Methylene sugars are convenient glycosyl donors and are utilized in both enzymatic and chemical glycosidations.6a,b,69b,f,g,70 However, there seems to be limitations in the use of exo-glycals because of their synthetic difficulty. In contrast, there is a wide variety of 1-C-modified sugars, and therefore, the O-ketosidation using 1-C-modified sugars can provide various types of O-ketosides. This section describes O-ketosidations using 1-C-modified D-glucopyranose derivatives. Scheme 10 shows the glucopyranose derivatives (1a–c, 1e) carrying the methyl, ethyl, n-butyl and benzyl groups at the anomeric carbon centers, utilized alcohols and produced O-ketopyranosides.
3.3.1 O-Ketosidation using dimethylphosphinothioate
We previously studied glycosidations using 1-O-dimethylphosphinothioyl sugars as the glycosyl donors.71 Our O-ketosidation study using 1-C-modified glucopyranoses started with the dimethylphosphinothioate method.8b,72 We examined the preparation of dimethylphosphinothioates from 1a–c using dimethylphosphinothioyl (Mpt) chloride and n-BuLi in THF at 0 °C, according to our previously reported procedure shown in Scheme 11. Dimethylphosphinothioate 25a was obtained from 1a in a moderate yield of 55%. However, other dimethylphosphinothioates were obtained in very poor yields from 1b and 1c. In all likelihood, the steric hindrances on the anomeric centers of 1b and 1c and the bulkiness of Mpt-Cl prevented the introduction of the Mpt group into 1b and 1c. Compound 25a was not as stable as the aldopyranosyl dimethylphosphinothioates we previously prepared.
We next investigated the O-ketosidation of 25a with the alcohols 26–28, as shown in Scheme 12. The reactivity of 25a as a glycosyl donor is expected to be high in spite of its anomeric carbon centre’s steric hindrance because the electron donating effect of the anomeric methyl group stabilize the glycosyl cation intermediate. We then used some trityl salts as activators of 25a, which were effective in the glycosidation of the highly reactive 2-deoxy-glycopyranosyl dimethylphosphinothioates. 3β-cholestanol (26) smoothly glycosylated 25a using 10 mol% TrtClO4 in benzene at room temperature to afford 32a in a high yield of 88% with an α-stereoselectivity. The reaction using 5 mol% TrtClO4 slightly decreased the yield of 32a to 71%. The reactions using 10 mol% of TrtSbCl6, TrtBF4 and TrtSnCl5 as other trityl salts afforded 32a in moderate yields from 34% to 43%. Under similar reaction conditions using 10 mol% TrtClO4, we successfully performed glycosidation of 25a with 27 and 28 to produce the O-glucosides 33a and 34a both in 82% yield with an α-stereoselectivity and an α/β ratio of 70/30. These results are indicated in Table 4.
3.3.2 O-Ketosidation using acetates
The acetyl group, less bulky than the Mpt group, could be smoothly introduced into 1a–c and 1e to prepare the corresponding acetates, as mentioned in the Section 3.1. Although the ordinary acetylation of 1a using Ac2O-pyridine could not give 38a at all,73 the use of n-BuLi as a strong base in lieu of pyridine was effective. We acetylated the compounds 1a–c and 1e with n-BuLi and Ac2O at −78–0 °C to produce the acetates 38a–c and 38e in good yields. The one-pot synthesis of 38a–c and 38e was also achieved from the glucono-1,5-lactone derivative 39 by adding RLi (or RMgX) and subsequently Ac2O in THF, as shown in Scheme 13.
We then investigated O-ketosidation using the acetates 38a–c and 38e in the presence of a Lewis acid, as shown in Scheme 14.8b,e First, the reaction of 38a with phenethyl alcohol (29) was examined in detail using 5 mol% of Lewis acids such as Yb(OTf)3, Sc(OTf)3, TMSOTf, TrtClO4 and BF3•OEt2 as the activators in CH2Cl2 at 0 °C. Each Lewis acid activated the glycosidation to give the corresponding glucoside 35a in 67–80% yields with α-stereoselectivities. Yb(OTf)3 and Sc(OTf)3 were especially effective for the activation of 38a. Even the reaction using only 1 mol% of Sc(OTf)3 could afford 35a in a yield of 73% with an α/β ratio of 81/19. We also examined the effect of the solvents using CH2Cl2, PhMe and MeCN. The reaction using PhMe increased the yield of 35a to 89%. However, these solvents did not influence the glycosidation stereoselectivities at all.
Under similar reaction conditions, the reactions of 38a–c with 29 (28) stereoselectively afforded the α-O-glucoside 35a–c (34a–c) and those of 38e with 29 (28) stereoselectively afforded the α-O-glucoside 35e (34e) in yields of 73–89%.74,75 B(OPh)3 (30) worked as the reactive glycosyl acceptors of 38a to stereoselectively afford the phenyl α-O-glucoside 36a in 80% yield.75c Table 5 displays these results.
Inanaga et al. reported that lanthanide triflates could not activate glycosidation using 2,3,4,6-tetra-O-benzyl-D-glucopyranosyl acetate as the glycosyl donor.76 This result greatly differ from our observations that any glycosidation using 38a–c and 38e smoothly proceeded using only 5 mol% Sc(OTf)3. The existence of the alkyl groups on 38a–c and 38e markedly increased their reactivities as glycosyl donors. The species of the alkyl groups at the anomeric carbon centers of 38a–c and 38e had almost no influence on the reactivities and stereoselectivities of the glycosidation. These findings corresponded to the observations about the C- and N-ketosidation mentioned in Section 3.1.
The high α-stereoselectivity of this reaction could be explained by the anomeric effect and by the inhibition of the β-glucoside formation by the 1,3-diaxial interaction. In addition, we examined the possibility that the β-O-glucoside might be isomerized into the corresponding α-O-glucoside in the reaction system. No anomerization was observed in the reaction using the β-glucoside of 34a to α-glucoside in the presence of 10 mol% Sc(OTf)3 in PhMe at room temperature overnight.
3.3.3 Dehydrative O-ketosidation
We next examined direct O-ketosidation using the 1-C-modified hexopyranoses 1a–c and 1e without introducing any other leaving group, as shown in Scheme 15, in order to develop a more convenient method for producing O-ketosides.8b,c Dehydrative O-ketosidation of 1a–c and 1e was performed using a Brφnsted acid expected to be resistant to water.
The reaction of 1a with 29 was investigated using 5 mol% of camphorsulfonic acid, CF3CO2H and TfOH in CH2Cl2 at 0 °C in the presence of CaSO4. Only TfOH was effective for the dehydrative O-ketosidation to give 35a in 59% yield with an α-stereoselectivity. The use of MeCN as the solvent slightly increased the yield of 35a to 73%. Similarly, we found C8F17SO3H and Tf2NH acting as the Brφnsted acid analogues of TfOH, and HBF4 to be effective under similar reaction conditions. In particular, Tf2NH gave 35a with a maximum yield of 77%.
Under the given reaction conditions, ketosidation of 1b, 1c and 1e with 29 gave the desired 35b, 35c and 35e, respectively, in yields ranging from 56% to 64% with high α-stereoselectivities. We also found that the difference in the alkyl groups at the anomeric carbon centers of 1a–c and 1e had almost no influence on the dehydrative O-ketosidation reactivity and stereoselectivity. This observation is similar to that of Section 3.3.2. Moreover, 1a and 1e smoothly glycosylated the compounds 27, 28 and 31 in good yields of 78%, 55% and 66%, respectively, with high α-stereoselectivities under the given reaction conditions. Table 6 lists these results.
Our O-ketosidation methods mentioned in Section 3.3 are useful for synthesizing various types of mimics of naturally occurring O-aldopyranosides, which are expected to show biological functions different from those of natural compounds.
3.4 Formation of trehalose mimics by 1-C-methyl-hexopyranosylation
One of the interesting findings on the aforementioned O-ketosidation using 1-C-modified hexopyranoses was that the ketopyranosylation proceeded with high α-stereoselectivity. We expected this feature to be useful for synthesizing mimics of trehalose (α-D-glucopyranosyl α-D-glucopyranoside) as this natural non-reducing disaccharide is composed of two glucose molecules linked together by an α-glucopyranosidic linkage. Trehalose is well known for its various biological activities such as its suppressive effect on the progress of osteoporosis.
We attempted the dehydrative O-ketosidation method to synthesize some non-reducing disaccharides (trehalose mimics) into which 1-C-methyl-D-hexopyranoses were incorporated, as shown in Scheme 16.8b,c,10 First, when we investigated the reaction of 1-C-methyl-D-glucopyranose (1a) with 2,3,4,6-tetra-O-benzyl-D-glucopyranose (40) using 5 mol% Tf2NH in MeCN at 0 °C for 3 h in the presence of CaSO4, the desired non-reducing disaccharide 41 was produced in 47% yield as a single isomer, and both of its glycosidic linkages were α-configured. This interesting observation urged us to make a further investigation into the ketosidation conditions of 1a with 40, including the stereoselectivity of the ketosidation and other effective activators in place of Tf2NH.
When we changed the solvent from MeCN to CH2Cl2, the reaction of 1a with 40 markedly increased the yield of 41 to 87%. This increase was due to the high solubility of 40 in CH2Cl2. We used Lewis acids, Yb(OTf)3, Sc(OTf)3 and Bi(OTf)3, which were expected to be resistant to water. The reactions using Sc(OTf)3 and Bi(OTf)3 gave 41 in high yields of 80% and 89%, respectively. Bi(OTf)3 was an efficient activator for this reaction,77,78 while BiCl3 did not work at all.
The syntheses of several non-reducing disaccharides by 1-C-methyl-hexopyranosylation were examined using the 1-C-methyl-hexopyranoses 1a or 42a and the aldopyranoses 40 and 43–45 by employing both Tf2NH and Bi(OTf)3 as the activators. We obtained the corresponding non-reducing disaccharides 41 and 46–49 in good yields. Compounds 41, 46 and 49 were obtained as single isomers. In addition, compound 47 was formed in the isomer ratios of 78/22 and 58/42, and compound 48 was formed in isomer ratios of 87/13 and 76/24. Table 7 displays these results. The anomeric configurations of all the formed ketopyranosidic linkages of 46–49 were α, which were determined by the observation of the NOE interactions between the methyl group and the H-2 of the 1-C-methyl-D-hexopyranosyl rings. The measurement of the coupling constants of H-1 determined the aldopyranosidic linkages of 47 and 48.
In order to investigate the relation between the α/β-anomer ratios of the acceptors and those of the aldopyranosidic linkages of the products, we measured the α/β-anomer ratios of the aldohexopyranoses 40 and 43–45 in CH2Cl2 solvent based on the NMR spectra. Compounds 40 and 43–45 were dissolved in CD2Cl2, and the NMR spectra measured about 30 min later showed that 40 and 43 contained ca. 15–20% of the β-anomers and that 44 and 45 contained ca. 40% of the β-anomers. In spite of the presence of the β-anomers, the ketopyranosylations of 40 and 43 did not form products with β-aldopyranosidic linkages at all. This suggested that the α-anomers of 40 and 43 predominantly worked as reactive acceptors. The ketopyranosylations of 44 and 45 formed small amounts of 47 and 48 with β-aldopyranosidic linkages. This showed that their β-anomers partly participated in the ketopyranosylations, although the α-anomers were preferentially used as reactive acceptors.
To the best of our knowledge, this study is the first example of the incorporation of a 1-C-methyl-D-hexopyranose into a non-reducing disaccharide.79 These trehalose mimics are expected to exhibit novel biological activities.
3.5 Formation of anhydroketopyranoses from 1-C-modified hexopyranoses
Several anhydroketopyranoses having 6,8-dioxabicyclo[3.2.1]octane structures are found in natural products such as Sedum spectabile,80 Smallanthus sonchifolius,81 and Coriaria japonica A.82 Coriariin found in C. japonica A has an anhydro-D-altro-heptulose bound to a tannin molecule via an ester linkage, as shown in Scheme 17. Its analogues possess biologically important antitumour and antivirus activities. In addition, the 6,8-dioxabicyclo[3.2.1]octane structural anhydroketopyranoses are useful chiral building blocks in synthetic organic chemistry. This section 3.5 describes the general reaction characteristics of the formation of anhydroketopyranoses by intramolecular O-ketosylation, i.e. ‘anhydroketopyranosylation’ of 1-C-modified hexopyranoses and a synthetic approach to an anhydro-D-altro-heptulose derivative found in C. japonica A.
3.5.1 Formation of anhydroketopyranoses by the intramolecular O-ketosidation
6,8-Dioxabicyclo[3.2.1]octane structural anhydroketopyranoses can be regarded as intramolecular O-ketopyranosides. The 1-C-modified hexopyranoses are expected to be useful precursors for providing various types of anhydroketopyranoses having 6,8-dioxabicyclo[3.2.1]octane structures. Therefore, we investigated the formation of these anhydroketopyranoses by direct anhydroketopyranosylation of 1-C-modified hexopyranoses.8d
Several 1-C-modified D-hexopyranoses, 50a, 50c–f and 51a carrying the methyl, allyl, n-butyl, phenyl and benzyl groups at the anomeric carbon centers and having a hydroxyl group at the C-6 position were utilized. We investigated the anhydroketopyranosylation of 50a to afford the corresponding anhydroketopyranose 52a using Brønsted acids as activators, as shown in Scheme 18, according to a concept similar to that mentioned in Section 3.3.3. Table 8 summarizes these results. Each reaction using 20 mol% of camphorsulfonic acid, Tf2NH and TfOH as the Brønsted acid in MeCN at 0 °C gave the desired 52a in 59%, 82% and 84% yields, respectively. Tf2NH and TfOH were very efficient for this anhydroketopyranosylation. We then varied the amount of TfOH. The reactions using only 1 mol% or 0.5 mol% of TfOH proceeded to give 51a in 86% and 68% yields, respectively. A maximum yield of 93% was attained in the reaction using 5 mol% of TfOH. Thus, the reactivity of 50a was remarkably high, a fact corresponding to the ‘intermolecular’ O-ketosidation mentioned in Section 3.3.3. The reaction from 2,3,4-tri-O-benzyl-D-glucopyranose (53) to 1,6-anhydro-2,3,4-tri-O-benzyl-β-D-glucopyranose (54) under similar reaction conditions scarcely proceeded. We considered that the presence of the anomeric methyl group of 50a would promote not only the generation of the oxocarbenium cation intermediate from 50a but also the conformation flip of the pyranosyl ring from 4C1 into 1C4 because of its equatorial orientation in the anhydroketopyranose form.
We then demonstrated the synthesis of various types of anhydroketopyranoses (52c–f) by anhydroketopyranosylation of 50c–f. The reactions using 50c and 50d in MeCN at 0 °C for 2 h in the presence of 5 mol% TfOH afforded the desired anhydroketopyranoses 52c and 52d in the excellent yields of 91% and 95%, respectively. The 1C4 conformational 52e and 52f were similarly obtained from 50e and 50f in 70% and 78% yields, respectively. Interestingly, in these two reactions, the twist-boat conformational isomers 52e’ and 52f’ were also formed in 26% and 14% yields. Therefore, the total yields of these glycosidations were 96% and 92%, respectively. These results showed that the difference in the 1-C-functional groups of 1a–e had almost no influence on the anhydroketopyranosylation yields, although the benzyl and phenyl groups of 50e and 50f influenced the ring conformations of the anhydroketopyranoses.
Anhydroketopyranosylation of 51a under same reaction conditions smoothly proceeded to afford 55a in a high yield of 93%. Furthermore, the reaction using 6-O-tert-butyldimethylsilyl-1-C-methyl-D-glucopyranose (56a) also afforded 52a in 80% yield. In this reaction, TfOH not only promoted the anhydroketopyranosylation but also removed the TBS group of 56a, which is a great advantage of this anhydroketopyranosylation. In none of the above reactions, we observed the production of oligosaccharides by intermolecular O-ketosidation.
3.5.2 Synthesis of an anhydro-D-altro-heptulose found in C. japonica A
We applied the aforementioned anhydroketopyranosylation to the synthesis of an anhydro-D-altro-heptulose83 found in C. japonica A.11a,b Our synthetic target was the partially benzylated anhydro-D-altro-heptulose derivative 57, as shown in Scheme 19. Compound 57 is a useful unit for synthesizing coriariin because it has a free hydroxyl group that functions as a binding site with a tannin molecule, and its other hydroxyl functions are protected by benzyl groups. Our synthetic approach to 57 starts from a D-mannopyranose derivative 58,84 and consists of the following key reaction steps: (i) steric inversion at the C-3 position from a D-mannopyranose derivative to a D-altropyranose derivative, (ii) introduction of a vinyl group to the C-1 position of the altropyranose derivative to produce a 1-C-vinylated D-altropyranose derivative, (iii) anhydroketopyranosylation of the 1-C-vinylated D-altropyranose derivative to form the anhydroketopyranose structure and (iv) conversion of the vinyl group of the anhydroketopyranose to a hydroxymethyl group to produce the desired compound 57.
We introduced a TBS group into the C-6 position of 58 using TBSCl-imidazole in CH2Cl2. Introduction of a Tf group to the C-3 position was performed using Tf2O in pyridine. Steric inversion at C-3 of 60 to 61 was successfully achieved in 62% yield using AcOCs in the presence of 18-crown-6 in PhMe at 30 °C for 24 h under ultrasonic conditions. After the conversion of the acetyl group of 61 to a benzyl group, we removed the allyl group of 63 using PdCl2 in AcOH-AcONa at 30 °C for 4 h under ultrasonic conditions. Oxidation of 64 using DMSO-Ac2O produced the desired 65. Introduction of the vinyl group to the C-1 position of 65 to produce 66 was successfully achieved in 62% yield using vinylmagnesium chloride in PhMe in the presence of CeCl3 at −78 °C for 1 h. The anhydroketopyranosylation of 66 smoothly proceeded to afford the desired compound 67 in 88% yield using 5 mol% TfOH in the presence of CaSO4 in MeCN at 0 °C for 2 h. It is unnecessary to remove the TBS group at C-6 of 66 prior to the anhydroketopyranosylation because the TBS group was removed by TfOH during the anhydroketopyranosylation as mentioned above. We converted the vinyl group of 67 into a hydroxymethyl group to give the desired 57 in 72% yield by ozone oxidation of 67 and treatment with triphenylphosphine at −78 °C for 45 min and subsequent reduction using NaBH4 at 0 °C for 3 h.
CONCLUSIONS
This paper summarizes our recent studies on the nucleophilic substitutions to form the ketopyranosidic linkages from the artificial ketoses having a naturally occurring aldohexopyranose backbone. We also reviewed the reports from other research groups. We believe that this paper can contribute to the further development of novel utilizations of 1-C-modified sugars.
ACKNOWLEDGEMENTS
We thank Prof. Kaname Katsuraya (Wayo Wemen's University) for NMR analyses, and Prof. Toshiyuki Inazu (Tokai University) and Dr. Akihiro Yoshida (The Noguchi Institute) for their helpful discussions. We are also grateful to Mr. Ippo Yamazaki, Mr. Yoshiki Oda, Mr. Kenji Morimoto, Ms. Masae Shinbara, Mr. Kazuhide Matsumura, Ms. Yukari Nara and Mr. Ryo Inoue for their supports in experiments.
References
1. ‘Comprehensive Glycoscience. From Chemistry to Systems Biology’, Vol. 1-3, ed. by J. P. Kamerling, G.-J. Boons, Y. C. Lee, A. Suzuki, N. Taniguchi, and A. G. J. Voragen, Elsevier Ltd., Oxford, 2007.
2. ‘Carbohydrates in Drug Design’, ed. by Z. J. Witczak and K. A. Nieforth, Marcel Dekker, Inc., New York, 1997.
3. ‘Carbohydrate Mimics. Concepts and Methods’, ed. by Y. Chapleur, H.-J. Altenbach, F. Chretien, J. M. Contelles, F. Nicotra, A. P. Rauter, and S. M. Roberts, Wiley-VCH, 1998.
4. ‘The Chemistry of C-Glycosides’, ed. by D. E. Levy and C. Tang, Pergamon, 1995.
5. (a) M. Benltifa, S. Vidal, D. Gueyrard, P. G. Goekjian, M. Msaddek, and J.-P. Praly, Tetrahedron Lett., 2006, 47, 6143; CrossRef (b) D. Pan, J. Liu, C. Senese, A. J. Hopfinger, and Y. Tseng, J. Med. Chem., 2004, 47, 3075; CrossRef (c) F. Stolz, M. Reiner, A. Blume, W. Reutter, and R. R. Schmidt, J. Org. Chem., 2004, 69, 665; CrossRef (d) L. Somsák, L. Kovács, M. Tóth, E. Ősz, L. Szilágyi, Z. Györgydeák, Z. Dinya, T. Docsa, B. Tóth, and P. Gergely, J. Med. Chem., 2001, 44, 2843. CrossRef
6. (a) R. Namme, T. Mitsugi, H. Takahashi, and S. Ikegami, Tetrahedron Lett., 2005, 46, 3033; CrossRef (b) X. Li, H.Ohtake, H. Takahashi, and S. Ikegami, Synlett, 2001, 1885. CrossRef
7. (a) Y. Yamamoto, K. Yamashita, T. Hotta, T. Hashimoto, M. Kikuchi, and H. Nishiyama, Chem. Asian J., 2007, 2, 1388; CrossRef (b) P. A. V. van Hooft, F. E. Oualid, H. S. Overkleeft, G. A. van der Marel, J. H. van Boom, and M. A. Leeuwenburgh, Org. Biomol. Chem., 2004, 2, 1395; CrossRef (c) X. Li, H. Takahashi, H. Ohtake, M. Shiro, and S. Ikegami, Tetrahedoron, 2001, 57, 8053; CrossRef (d) S. Czernecki and M.-C. Perlat, J. Org. Chem., 1991, 56, 6289; CrossRef (e) S. Hanessian and A. Ugolini, Carbohydr. Res., 1984, 130, 261. CrossRef
8. (a) Y. Oda and T. Yamanoi, Synthesis, 2007, 3021; CrossRef (b) T. Yamanoi, Y. Oda, S. Matsuda, I. Yamazaki, K. Matsumura, K. Katsuraya, M. Watanabe, and T. Inazu, Tetrahedron, 2006, 62, 10383; CrossRef (c) T. Yamanoi, S. Matsuda, I. Yamazaki, R. Inoue, K. Hamasaki, and M. Watanabe, Heterocycles, 2006, 68, 673; CrossRef (d) T. Yamanoi, K. Matsumura, S. Matsuda, and Y. Oda, Synlett, 2005, 2973; CrossRef (e) T. Yamanoi, Y. Oda, I. Yamazaki, M. Shinbara, K. Morimoto, and S. Matsuda, Lett. Org. Chem., 2005, 2, 242; CrossRef (f) T. Yamanoi and Y. Oda, Heterocycles, 2002, 57, 229. CrossRef
9. T. Yamanoi, Y. Nara, S. Matsuda, Y. Oda, A. Yoshida, K. Katsuraya, and M. Watanabe, Synlett, 2007, 785. CrossRef
10. T. Yamanoi, R. Inoue, S. Matsuda, K. Katsuraya, and K. Hamasaki, Tetrahedron: Asymmetry, 2006, 17, 2914. CrossRef
11. (a) S. Matsuda, T. Yamanoi, and M. Watanabe, Tetrahedron, 2008, 64, 8082; CrossRef (b) S. Matsuda, K. Matsumura, M. Watanabe, and T. Yamanoi, Tetrahedron Lett., 2007, 48, 5807. CrossRef
12. X. Li, H. Ohtake, H. Takahashi, and S. Ikegami, Tetrahedron, 2001, 57, 4297. CrossRef
13. A. M. Gómez, C. Uriel, S. Jarosz, S. Valverde, and J. C. López, Eur. J. Org. Chem., 2003, 4830. CrossRef
14. B. I. Glänzer and R. Csuk, Carbohydr. Res., 1991, 220, 79. CrossRef
15. R. Namme, T. Mitsugi, H. Takahashi, and S. Ikegami, Eur. J. Org. Chem., 2007, 3758. CrossRef
16. G. Májer, A. Borbás, T. Z. Illyés, L. Szilágyi, A. C. Bényei, and A. Lipták, Carbohydr. Res., 2007, 342, 1393. CrossRef
17. M. A. Leeuwenburgh, C. C. M. Appeldoorn, P. A. V. van Hooft, H. S. Overkleeft, G. A. van der Marel, and J. H. van Boom, Eur. J. Org. Chem., 2000, 873. CrossRef
18. K. Tomooka, Y. Nakamura, and T. Nakai, Synlett, 1995, 321. CrossRef
19. P. A. V. van Hooft, M. A. Leeuwenburgh, H. S. Overkleeft, G. A. van der Marel, C. A. A. van Boechel, and J. H. van Boom, Tetrahedron Lett., 1998, 39, 6061. CrossRef
20. L. Lay, M. Meldal, F. Nicotra, L. Panza, and G. Russo, Chem. Commun., 1997, 1469. CrossRef
21. T. Yamanoi, Y. Oda, H. Muraishi, and S. Matsuda, Molecules, 2008, 13, 1840. CrossRef
22. P. A. V. van Hooft, G. A. van der Marel, C. A. A. van Boechel, and J. H. van Boom, Tetrahedron Lett., 2001, 42, 1769. CrossRef
23. P. A. V. van Hooft, P. F. van Swieten, G. A. van der Marel, C. A. A. van Boechel, and J. H. van Boom, Synlett, 2001, 269. CrossRef
24. A. M. Gómez, C. Uriel, S. Valverde, S. Jarosz, and J. C. López, Tetrahedoron Lett., 2002, 43, 8935. CrossRef
25. F. E. McDonald, H. Y. H. Zhu, and C. R. Holmquist, J. Am. Chem. Soc., 1995, 117, 6605. CrossRef
26. S. Groothuys, S. (B.) A. M. W. van den Broek, B. H. M. Kuijpers, M. IJsselstijn, F. L. van Delft, and F. P. J. T. Rutjes, Synlett, 2008, 111. CrossRef
27. A. M. Gómez, C. Uriel, S. Valverde, and J. C. López, Org. Lett., 2006, 8, 3187. CrossRef
28. A. Dondoni, A. Marra, I. Roji, and M.-C. Scherrmann, Tetrahedron, 1996, 52, 3057. CrossRef
29. A. Dondoni, S. Daninos, A. Marra, and P. Formaglio, Tetrahedron, 1998, 54, 9859. CrossRef
30. D. Noort, N. C. R. van Straten, G. J. P. H. Boons, G. A. van der Marel, X. Bossuyt, N. Blanckaert, G. J. Mulder, and J. H. van Boom, Bioorg. Med. Chem. Lett., 1992, 2, 583. CrossRef
31. A. Dondoni, A. Marra, M.-C. Scherrmann, and V. Bertolasi, Chem. Eur. J., 2001, 7, 1371. CrossRef
32. B. M. Heskamp, D. Noort, G. A. van der Marel, and J. H. van Boom, Synlett, 1992, 713. CrossRef
33. D. Noort, G. A. van der Marel, G. J. Mulder, and J. H. van Boom, Synlett, 1992, 224. CrossRef
34. K. Czifrák, L. Kovács, K. E. Kövér, and L. Somsák, Carbohydr. Res., 2005, 340, 2328. CrossRef
35. D. Crich and L. B. L. Lim, Tetrahedron Lett., 1991, 32, 2565. CrossRef
36. L. Somsák, L. Kovács, V. Gyóllai, and E. Ősz, Chem. Commun., 1999, 591. CrossRef
37. A. Borbás, G. Szabovik, Z. Antal, P. Herczegh, A. Agócs, and A. Lipták, Tetrahedron Lett., 1999, 40, 3639. CrossRef
38. A. Borbás, G. Szabovik, Z. Antal, K. Fehér, M. Csávás, L. Szilágyi, P. Herczegh, and A. Lipták, Tetrahedron: Asymmetry, 2000, 11, 549. CrossRef
39. A. Borbás, M. Csávás, L. Szilágyi, G. Májer, and A. Lipták, J. Carbohydr. Chem., 2004, 23, 133. CrossRef
40. F. Schweizer, A. Otter, and O. Hindsgaul, Synlett, 2001, 1743. CrossRef
41. M. Penner, D. Taylor, D. Desautels, K. Marat, and F. Schweizer, Synlett, 2005, 212. CrossRef
42. M. Penner and F. Schweizer, Carbohydr. Res., 2007, 342, 7. CrossRef
43. S. Czernecki and M.-C. Perlat, J. Carbohydr. Chem., 1990, 9, 915. CrossRef
44. R. Caputo, A. Guaragna, G. Palumbo, S. Pedatella, and F. Solla, Eur. J. Org. Chem., 2002, 534. CrossRef
45. M. de Kort, A. D. Regenbogen, A. R. P. M. Valentjin, R. A. J. Challiss, Y. Iwata, S. Miyamoto, G. A. van der Marel, and J. H. van Boom, Chem. Eur. J., 2000, 6, 2696. CrossRef
46. R. Caputo, U. Ciriello, P. Festa, A. Guaragna, G. Palumbo, and S. Pedatella, Eur. J. Org. Chem., 2003, 2617. CrossRef
47. R. Preuss, K.-H. Jung, and R. R. Schmidt, Liebigs Ann. Chem., 1992, 377. CrossRef
48. D. Crich and T. J. Ritchie, J. Chem. Soc., Perkin Trans. 1, 1990, 945. CrossRef
49. D. Crich and T. J. Ritchie, J. Chem. Soc., Chem. Commun., 1988, 1461. CrossRef
50. D. Crich and T. J. Ritchie, J. Chem. Soc., Chem. Commun., 1988, 985. CrossRef
51. D. Crich and F. Hermann, Tetrahedron Lett., 1993, 34, 3385. CrossRef
52. E. Modica, F. Compostella, D. Colombo, L. Franchini, M. Cavallari, L. Mori, G. De Libero, L. Panza, and F. Ronchetti, Org. Lett., 2006, 8, 3255. CrossRef
53. A. Marra, A. Dondoni, and F. Sansone, J. Org. Chem., 1996, 61, 5155. CrossRef
54. A. Dondoni, M.-C. Scherrmann, A. Marra, and J.-L. Delépine, J. Org. Chem., 1994, 59, 7517. CrossRef
55. A. Dondoni and A. Marra, Chem. Rev., 2000, 100, 4395. CrossRef
56. B. M. Heskamp, G. H. Veeneman, G. A. van der Marel, C. A. A. van Boeckel, and J. H. van Boom, Tetrahedron, 1995, 51, 5657. CrossRef
57. F. Schweizer, A. Lohse, A. Otter, and O. Hindsgaul, Synlett, 2001, 1434. CrossRef
58. A. Lohse, F. Schweizer, and O. Hindsgaul, Comb. Chem. High Throughput Screen., 2002, 5, 389.
59. F. Schweizer and O. Hindsgaul, Carbohydr. Res., 2006, 341, 1730. CrossRef
60. J. L. Koviach, M. D. Chappell, and R. L. Halcomb, J. Org. Chem., 2001, 66, 2318. CrossRef
61. J. W. Lane and R. L. Halcomb, Tetrahedron, 2001, 57, 6531. CrossRef
62. J. W. Lane and R. L. Halcomb, J. Org. Chem., 2003, 68, 1348. CrossRef
63. C.-C. Lin, M. Shimazaki, M.-P. Heck, S. Aoki, R. Wang, T. Kimura, H. Ritzèn, S. Takayama, S.-H. Wu, G. Weitz-Schmidt, and C.-H. Wong, J. Am. Chem. Soc., 1996, 118, 6826. CrossRef
64. D. Crich and T. J. Ritchie, Carbohydr. Res., 1989, 190, C3. CrossRef
65. F. P. Franke, M. Kapuscinski, and P. Lyndon, Carbohydr. Res., 1985, 143, 69. CrossRef
66. D. Crich, J.-T. Hwang, and H. Yuan, J. Org. Chem., 1996, 61, 6189. CrossRef
67. B. M. Heskamp, G. H. Veeneman, G. A. van der Marel, C. A. A. van Boeckel, and J. H. van Boom, Tetrahedron, 1995, 51, 8397. CrossRef
68. W.-B. Yang, Y.-Y. Yang, Y.-F. Gu, S.-H. Wang, C.-C. Chang, and C.-H. Lin, J. Org. Chem., 2002, 67, 3773. CrossRef
69. (a) B. Bourdon, M. Corbet, P. Fontaine, P. G. Goekjian, and D. Gueyrard, Tetrahedron Lett., 2008, 49, 747; CrossRef (b) M. Corbet, B. Bourdon, D. Gueyrard, and P. G. Goekjian, Tetrahedron Lett., 2008, 49, 750; CrossRef (c) X. Zhu, Y. Jin, and J. Wickham, J. Org. Chem., 2007, 72, 2670; CrossRef (d) G. S. Coumbarides, M. Motevalli, W. A. Muse, and P. B. Wyatt, J. Org. Chem., 2006, 71, 7888; CrossRef (e) R. J. K. Taylor, G. D. McAllister, and R. W. Franck, Carbohydr. Res., 2006, 341, 1298; CrossRef (f) C.-H. Lin, H.-C. Lin, and W.-B. Yang, Current Topics in Med. Chem., 2005, 5, 1431; CrossRef (g) C. Taillefumier and Y. Chapleur, Chem. Rev., 2004, 104, 263; CrossRef (h) P. A. Colinas, A. Ponzinibbio, A. Lieberknecht, and R. D. Bravo, Tetrahedron Lett., 2003, 44, 7985; CrossRef (i) P. A. Colinas, A. Lieberknecht, and R. D. Bravo, Tetrahedron Lett., 2002, 43, 9065; CrossRef (j) J.-P. Praly, G.-R. Chen, J. Gola, and G. Hetzer, Eur. J. Org. Chem., 2000, 2831. CrossRef
70. Enzymatic method; (a) E. M. H. Duke, S. Wakatsuki, A. Hadfield, and L. N. Johnson, Protein Science, 1994, 3, 1178; CrossRef (b) W. Weiser and J. Lehmannv, Biochemistry, 1988, 27, 2294; CrossRef (c) H. W. Klein, M. J. Im, and D. Palm, Eur. J. Biochem., 1986, 157, 107; CrossRef (d) P. Schlessmann, H. Fritz, J. Lehmann, T. Uchiyama, C. F. Brewer, and E. J. Hehre, Biochemistry, 1982, 21, 6606; CrossRef Chemical method; (e) R. Namme, T. Mitsugi, H. Takahashi, M. Shiro, and S. Ikegami, Tetrahedron, 2006, 62, 9183; CrossRef (f) S.-J. Hsu, H.-C. Lin, and C.-H. Lin, Carbohydr. Res., 2006, 341, 1428; CrossRef (g) H.-C. Lin, W.-P. Du, C.-C. Chang, and C.-H. Lin, Tetrahedron Lett., 2005, 46, 5071; CrossRef (h) H.-C. Lin, C.-C. Chang, J.-Y. Chen, and C.-H. Lin, Tetrahedron: Asymmetry, 2005, 16, 297; CrossRef (i) G. V. M. Sharma, Rakesh, A. S. Chander, V. G. Reddy, M. H. V. R. Rao, and A. C. Kunwar, Tetrahedron: Asymmetry, 2003, 14, 2991; CrossRef (j) H.-C. Lin, W.-B. Yang, Y.-F. Gu, C.-Y. Chen, C.-Y. Wu, C.-H. Lin, Org. Lett., 2003, 5, 1087; CrossRef (k) C.-F. Chang, W.-B. Yang, C.-C. Chang, and C.-H. Lin, Tetrahedron Lett., 2002, 43, 6515; CrossRef (l) D. E. Paterson, F. K. Giffin, M.-L. Alcaraz, and R. J. K. Taylar, Eur. J. Org. Chem., 2002, 1323; CrossRef (m) X. Li, H. Ohtake, H. Takahashi, and S. Ikegami, Tetrahedron, 2001, 57, 4283; CrossRef (n) M.-L. Alcaraz, F. K. Giffin, D. E. Paterson, and R. J. K. Taylor, Tetrahedron Lett., 1998, 39, 8183. CrossRef
71. (a) T. Yamanoi, H. Hamada, Y. Oda, and K. Hattori, Lett. Drug Design Discovery, 2006, 3, 547; CrossRef (b) T. Yamanoi, J. Inagaki, M. Mizuno, and T. Inazu, Heterocycles, 2000, 52, 921; CrossRef (c) T. Yamanoi, A. Fujioka, and T. Inazu, Bull. Chem. Soc. Jpn., 1994, 67, 1488; CrossRef (d) T. Yamanoi, K. Nakamura, H. Takeyama, K. Yanagihara, and T. Inazu, Bull. Chem. Soc. Jpn., 1994, 67, 1359; CrossRef (e) T. Yamanoi, K. Nakamura, S. Sada, M. Goto, Y. Furusawa, M. Takano, A. Fujioka, K. Yanagihara, Y. Satoh, H. Hosokawa, and T. Inazu, Bull. Chem. Soc. Jpn., 1993, 66, 2617; CrossRef (f) T. Yamanoi, K. Nakamura, H. Takeyama, K. Yanagihara, and T. Inazu, Chem. Lett., 1993, 343; CrossRef (g) T. Yamanoi and T. Inazu, Chem. Lett., 1990, 849; CrossRef (h) T. Inazu and T. Yamanoi, Chem. Lett., 1989, 69. CrossRef
72. T. Inazu and T. Yamanoi, Jpn. Kokai Tokkyo Koho, 1990, JP 02240093.
73. It was reported that the acetylation of a ketopyranose derivative with DMAP-Ac2O gave the open ring compound. See ref. 32.
74. The ketosidation system using Sc(OTf)3 was also successfully applicable to the O-ketosidation of D-fructofuranose derivatives. T. Yamanoi, N. Misawa, and M. Watanabe, Tetrahedron Lett., 2007, 48, 6458. CrossRef
75. We also reported the glycosidation methods using glycosyl acetates. (a) T. Yamanoi, S. Nagayama, H.-K. Ishida, J. Nishikido, and T. Inazu, Synth. Commun., 2001, 31, 899; CrossRef (b) T. Yamanoi and I. Yamazaki, Tetrahedron Lett., 2001, 42, 4009; CrossRef (c) T. Yamanoi, Y. Iwai, and T. Inazu, Heterocycles, 2000, 53, 1263; CrossRef (d) T. Yamanoi, Y. Iwai, and T. Inazu, J. Carbohydr. Chem., 1998, 17, 819. CrossRef
76. J. Inanaga, Y. Yokoyama, and T. Hanamoto, Tetrahedron Lett., 1993, 34, 2791. CrossRef
77. There were several glycosidation reports using Bi(OTf)3 as follows. (a) K. Ikeda, Y. Ueno, S. Kitani, R. Nishino, and M. Sato, Synlett, 2008, 1027; CrossRef (b) S. Valerio, A. Iadonisi, M. Adinolfi, and A. Ravida, J. Org. Chem., 2007, 72, 6097; CrossRef (c) M. Adinolfi, A. Iadonisi, A. Ravida, and S. Valerio, Tetrahedron Lett., 2006, 47, 2595; CrossRef (d) J. Babu, A. Khare, and Y. Vankar, Molecules, 2005, 10, 884; CrossRef (e) J. S. Yadav, B. V. S. Reddy, R. K. Srinivasa, L. Chandraiah, and V. Sunitha, Synthesis, 2004, 2523; CrossRef (f) K. Ikeda, Y. Torisawa, T. Nishi, J. Minamikawa, K. Tanaka, and M. Sato, Bioorg. Med. Chem., 2003, 11, 3073. CrossRef
78. We also found that Bi(OTf)3 was effectively catalyzed the glcosidaton using 1-hydroxy sugars. (a) T. Yamanoi, R. Inoue, S. Matsuda, and K. Hamasaki, Lett. Org. Chem., 2008, 5, 30; CrossRef (b) T. Yamanoi, R. Inoue, S. Matsuda, K. Iwao, Y. Oda, A. Yoshida, and K. Hamasaki, Heterocycles, 2009, 77, 445. CrossRef
79. A similar paper was reported. See ref. 15.
80. F. B. La Forge and C. S. J. Hudson, Biol. Chem., 1917, 30, 61.
81. M. Takenaka and H. Ono, Tetrahedron Lett., 2003, 44, 999. CrossRef
82. T. Hatano, R. Yoshihara, S. Hattori, M. Yoshizaki, T. Shingu, and T. Okuda, Chem. Pharm. Bull., 1992, 40, 1703.
83. Other synthetic approaches to an anhydro-D-altro-heptulose were as follows. (a) C. G. Francisco, A. J. Herrera, and E. Suárez, J. Org. Chem., 2002, 67, 7439; CrossRef (b) Z. Hricovíniová-Bíliková and L. Petruš, Carbohydr. Res., 1999, 320, 31; CrossRef (c) V. Dalmas and C. Demuynck, Tetrahedron: Asymmetry, 1993, 4, 1169. CrossRef
84. T. Ogawa, S. Nakabayashi, and T. Kitajima, Carbohydr. Res., 1983, 114, 225. CrossRef