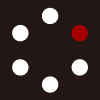
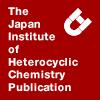
HETEROCYCLES
An International Journal for Reviews and Communications in Heterocyclic ChemistryWeb Edition ISSN: 1881-0942
Published online by The Japan Institute of Heterocyclic Chemistry
e-Journal
Full Text HTML
Received, 20th October, 2009, Accepted, 25th December, 2009, Published online, 29th December, 2009.
DOI: 10.3987/REV-09-662-1
■ Recent Development in Palladium-Mediated Synthesis of Oxygen Heterocycles
Krishna C. Majumdar,* Buddhadeb Chattopadhyay, Pradip K. Maji, Sudip K. Chattopadhyay, and Srikanta Samanta
Department of Chemistry, University of Kalyani, Kalyani 741 235, West Bengal, India
Abstract
This review describes the synthesis of oxygen heterocycles by palladium-mediated cyclization published during 2003 to 2007.CONTENTS
1 Introduction
2 Intramolecular reactions of alkynes, alkenes, allenes: Heck, Suzuki and Sonogashira type reactions
2. 1 Reactions of aryl halides
2. 2 Reactions of vinyl halides
2. 3 Reactions of enolates
3 Cyclization via C-H bond functionalization reactions
3. 1 Functionalization of alkane C-H bonds
3. 2 Functionalization of aromatic C-H bonds
3. 2. 1 Direct functionalization reactions
3. 2. 2 Functionalization via 1,4-palladium migration
4 Cyclization of 1,n-unsaturated systems: Cycloisomerization and cascade addition-cyclization reactions
4. 1 Cycloisomerization reactions
4. 2 Cascade addition-cyclization reactions
5 Cycloaddition reactions
5. 1 [3+2] Cycloaddition reactions
5. 2 [4+2] Cycloaddition reaction
5. 3 [4+3] Cycloaddition reaction
5. 4 [2+2+1] Cycloaddition reactions
6 Heterocyclization reactions: Cyclization via carbon-heteroatom bond formation
6. 1 Intramolecular addition of O-H, C=O bonds across alkene, allene and alkyne
6. 1. 1 Addition to alkene
6. 1. 2 Addition to allene
6. 1. 3 Addition to alkyne
6. 2 Intramolecular coupling of OH with aryl halides
6. 3 Cyclocarbonylation and cyclocarboxylation reactions
7 Cyclization via cascade carbon-carbon/carbon-heteroatom bond formation: Heterocyclization reactions
7. 1 Heterocyclization reactions with alkynes
7. 1. 1 Reactions with terminal alkynes: Sonogashira coupling-cyclization reactions
7. 1. 2 Reactions with internal alkynes
7. 2 Heterocyclization reactions with alkenes
7. 3 Heterocyclization reactions with dienes
8 Palladium-catalyzed multi-component reaction
8. 1 Palladium-catalyzed three-component reaction
9 Miscellaneous
9. 1 Intramolecular Heck Reaction
9. 2 Cycloaddition reaction
9. 3 Intramolecular addition of N-H bonds across alkene
9. 4 Intramolecular coupling of N-H and OH with aryl halide
9. 5 Cyclocarbonylation and cyclocarboxylation reaction
9. 6 Heterocyclization reaction with alkyne
9. 7 Palladium-catalyzed four-component reaction
9. 8 Claisen rearrangement
9. 9 Electrocyclization reaction
10 Conclusion
11 Acknowledgements
12 References
1. INTRODUCTION
The unusual growth of worldwide demand of heterocyclic compounds due to their pharmacological and biological activities have attracted organic chemists for continuous research directed towards the development of novel and more effective synthetic strategies. Many of these strategies involve the formation of either carbon-carbon or carbon-heteroatom bond from the corresponding acyclic precursors. Among several newly developed methodologies, the employment of palladium catalysis in oxygen heterocycles synthesis have proven its efficiency and importance to the level where this is now routinely considered in strategy level planning of complex targets.
Last decade has encountered an overwhelming development in the field of palladium chemistry and its significant contributions in the synthesis of heterocycles and have brought renaissance in chemical laboratories as well as industries. A wealth of books1 and reviews2 covering particular and limited aspects of organopalladium chemistry are available. The wide utility of palladium in organic synthesis is evident from the large number of name reactions where the deep influences of this versatile transition metal enable it in the formation of C-C, C-O, C-N and even C-S bond under relatively mild conditions. The catalytic requirement and excellent tolerance of functional groups avoiding the protection-deprotection chemistry has made possible the use of palladium in the synthesis of small to large ring heterocyclic compounds. Moreover, the development of asymmetric transformation using chiral ligand is a major break through in the palladium-catalyzed synthesis of heterocycles.3 In addition, the Pd-catalyzed domino multiple transformation, in recent years, have been the general need both from economical and ecological ground.4
Palladium metal being a member of the late transition series in the periodic table forms stable palladium complexes of oxidation state: Pd(0), Pd(II) and Pd(IV). Different synthetic strategies employing both Pd(0)-complexes and Pd(II)-salts have been developed and facile reversible redox process due to small energy difference between these two preferred oxidation states have enriched the organic chemistry especially heterocyclic chemistry. Although catalytic amount of palladium is required for successful conversion, the catalyzed processes are in fact strongly dependent on other factors eg. base, ligand, temperature, additives and solvents. A tunable reaction conditions enable the palladium chemistry more flexible for the future endeavors of novel and exciting chemistry, despite the vast amounts of studies reported so far.
The search for various heterocycles and many new methodologies involving palladium has been the central goal in recent years. Our prime objective is to provide a complete and updated summary of palladium-catalyzed approaches for the preparation of oxygen heterocycles developed from 2003 onwards with the emphasis on the underlying principle following each synthetic procedure and the reliance of the results on the appropriate choice of reaction conditions. As industrial preparations of many heterocyclic compounds have only been reported as patents outlining only the manufacturing route, a thorough review of the patent literature, which is often difficult to interpret, is beyond the scope of this review. Procedures where palladium-catalysis are not involved in the final construction of heterocyclic ring has not been included in this review.
2. INTRAMOLECULAR REACTIONS WITH ALKYNES, ALKENES, ALLENES, ARENES AND HETEROARENES: HECK, SUZUKI, STILLE AND SONOGASHIRA TYPE REACTIONS
The potentiality of C-C unsaturated bond as carbon source to get coupled with aryl- and vinyl halides or organometallics in a palladium mediated reaction is well documented.2,5 Heck reaction1a,2a,6 is of particular prominence in the synthesis of heterocycles. The intramolecular version of this reaction with aryl or vinyl halides generally proceeds through a sequence of oxidative addition to C-X (X = halogen) bond, insertion and β-elimination (for olefins) or protonolysis (for alkynes) to generate the heterocycles ranging from small to macrocyclic compounds. The observed rate of oxidative addition with C-X bonds decreases according to the following order: C-I> C-Br> C-Cl> C-F.7 The reactivity of aryl triflates is in between that of aryl iodides and aryl bromides. Additives also play a significant role in controlling the reaction outcome of palladium-catalyzed reactions.8 Recently intramolecular reactions have been developed involving related carbopalladation reactions followed by trapping with nucleophilic reagents.9
For macrocyclization via C-C bond formation with olefin or alkyne, the well-known Heck, Suzuki, Sonogashira and Stille reactions are now routinely considered.10 A different approach to C-C bond formation involving the palladium-catalyzed intramolecular reaction of enolate derived from ketones, esters and amides with aryl or vinyl halide11 or oxidative coupling with olefin12 has been utilized for the synthesis of five- and six-membered heterocyclic compounds. It is well established that for small sized ring the intramolecular Heck reaction usually favours exo-cyclization as due to steric reason endo-cyclization, which generate energetically favorable substituted alkene product, is less probable.2a By contrast, macrocycle formation proceeds through a favourable endo-cyclization pathway.10
2.1. REACTIONS OF ARYL HALIDES
In palladium-catalyzed Heck reaction, aryl iodide has been found to be the most commonly used halide source.13 However, the use of aryl bromide or aryl chloride for the construction of heterocycles would be very much interesting from the synthetic point of view.14 In an investigation, Liu et al. reported the use of aryl bromide 1 for the synthesis of 2,3-dihydrobenzofurans 2 under ligand free Pd(OAc)2–catalyzed reductive Heck15 cyclization (Scheme 1).16 However, an excellent improvement in the yield of 2 (87%) was observed under the Buchwald condition.17 By employing various palladium complexes [(t-Bu)2P(OH)]2-PdCl2, [(t-Bu)2P(OH)(t-Bu)]2-PdCl2 and [(t-Bu)2P(OH)-PdCl2]2 developed by Li,18 a 70% yield of 2 was obtained.
The efficiency of the methodology was explored by the synthesis of chromans and isochromans in excellent yields. Quite interestingly, the greater propensity of formation of normal Heck product 7 instead of reductive cyclization product 5 from the ether 3, under reductive Heck condition, clearly indicated faster β-H elimination in comparison to competing hydride reduction to 5. The exo-alkene intermediate 6 isomerized under the reaction conditions to afford thermodynamically more stable 7 in 74% isolated yield (Scheme 2).
A systematic study to account for the conformational effects on the intramolecular Heck reactions of ether and ester tethered aryl iodides were carried out by Branchaud et al.19 Allyl-2-iodobenzyl ether 8 when subjected to established Heck condition (Scheme 3, condition A) afforded the six-membered products 6 and 7 (82:18) in good yields.
In contrast to ether, the intramolecular Heck reaction20 cyclohexyl-2-iodobenzoate 9 suffered from a competition between cyclization over deiodination leading to the product 12 predominated over exo-cyclization and afforded cyclized products 10 + 11 in poor yields (Scheme 4).
The use of ionic liquids21 in PdCl2-catalyzed intramolecular Heck reaction for the synthesis of benzofuran derivative 14 was demonstrated by She et al.22 The ionic liquid, [bmim]BF4 was utilized to conduct the reaction and compound 14 was obtained from o-iodophenyl allyl ether 13 (Scheme 5).
Lautens and Fang reported23 that the catalytic combination of Pd2(dba)3/HP(t-Bu)3.BF4 and DABCO in dioxane afforded an unusual intramolecular Heck reaction with dihydronaphthalene substrates 15 yielding formal anti-hydride elimination24 products 16 via 17 in good-to-excellent yields under mild conditions. It is easily understood that the base removed a proton from the benzylic palladium intermediate 17 in an antiperiplanar fashion in the rate determining step25 (Scheme 6).
The Pd(OAc)2-catalyzed asymmetric allylic alkylation of chiral o-bromoarylcyclohexenyl ethers 18a,b for the construction of tricyclic benzofuran moieties 19a,b was described.26 Utilizing Jeffery condition27 and also in the presence of monodentate ligand P(o-tol)3, the cyclization is always accompanied by competitive ionization product. At this point different ligand was used to get more Heck reaction product. It is observed that in presence of Pd(OAc)2/bis(1,4-diphenylphosphino)propane(dppp) and silver carbone the enantiopure o-bromoarylcyclohexenyl ethers 18a,b give correspong Heck product 19a,b in excellent yield (Scheme 7).
Pattenden and co-workers in an investigation towards the formation of quarternary stereocenter and heterocyclic core of diazonide A, reported that iodoalkene 20, as a 3:1 mixture of Z- and E-isomers, when subjected to Pd(OAc)2 in DMF in the presence of Ag2CO3 and PPh3 for 4 days underwent 5-exo-trig cyclization to afford benzodihydrofuran 21 in 72% yield (Scheme 8).28 The same catalytic combination in THF when applied to the mixture of syn and anti diastereomers 22, gave spiro chromene products 23 and 24, resulting from a 6-exo-trig cyclization. However, in N,N-dimethylacetamide at higher temperature (85–90 oC), a small amount (~ 8%) of the corresponding isomeric ether 25, resulting from a competing 7-endo-trig cyclization, was produced concurrently (Scheme 8).
A similar intramolecular 5-exo-trig cyclization to generate quarternary stereocenter was also observed during the synthesis of tyrosine derived model benzofuranone 27.29 The intramolecular Heck reaction of benzoate 26 in the presence of Pd2(dba)3.CHCl3 as catalyst, (S)-BINAP as ligand and Ag3PO4 as additive afforded the cyclized product 27 as a 3:2 mixture of diastereomers (Scheme 9).
Norbornene-mediated Pd(OAc)2-catalyzed reaction of o-iodo alkenyl ether 28 in the presence of different alkyl bromides 29 afforded chromene-4-ylidines 30 and 1-benzoxepin-5-ylidines 31 (Scheme 10).30 The ring forming step involves the palladium-catalyzed ortho-alkylation with an intramolecular exo-trig Heck type cyclization where two carbon-carbon bonds (of which one from an unactivated aryl C-H bond) are formed in one-pot.
Methyl-1,2-dihydro-1-napthalenol substituted benzofuran and 1H-isochromene derivative 34 were prepared31 by a palladium-catalyzed cascade ring closure/ring opening reaction. By using Pd(PPh3)2Cl2 as catalyst and Zn metal as mild Lewis acid, 2-iodophenoxyallenes 32a and 2-iodobenzyloxyallenes 32b in the presence of oxabenzonorbornadienes 33 generated the desired cyclized products 34a,b in good yields (Scheme 11). The reaction also works well when X = NTs affording corresponding nitrogen heterocycles in good yields.31
We have reported the synthesis of medium-sized naphthalene based oxygen heterocycles by intramolecular Heck reaction.32 The Pd(OAc)2-catalyzed reaction of 2- and 1-naphthyl ethers 35 afforded eight-membered oxa-cycle 36 in good yield via 8-exo-trig cyclization. The 9-endo product 37 was isolated in 20% yield only in the case of ether 35a (Scheme 12).
Intramolecular hydroarylation reaction of the homopropargyl ether 38 with Pd(OAc)2 catalyst was reported to afford the chromene 39 along with inseparable alkyne byproduct 40 (Scheme 13).33
We have utilized the Jeffery’s two-phase protocol for the construction of a number of tetracycyclic coumarin- and quinolone-annulated oxygen heterocycles 42 and 44 (Scheme 14).34 The process is quite general, regioselective and afforded the desired products in good-to-excellent yields. Here it is observed that for quinolone moiety the reaction yield is slight lower than that for corresponding coumarin moiety. By using Pd(OAc)2 as catalyst, KOAc as base, and n-Bu4NBr as additive in DMF a series of coumarin and quinolone derivatives 42a-d and 44a-d were synthesized.
2.2 REACTIONS OF VINYL HALIDES
Intramolecular reactions of vinyl halides with C-C unsaturated bond have also been applied to the construction of heterocyclic compounds. The intramolecular Heck reaction of compound (+)-45 with Pd(OAc)2 afforded 8-exo-trig and 9-endo-trig cyclzed products 46 and 4735 (Scheme 15).
In an attempt to synthesize highly strained polyunsaturated macrolactone, parent moiety of the natural product oximidine II,36 employing intermolecular transesterification and Suzuki type cross-coupling10 between trifluoroborate 48 and secondary alcohol 49, the 11-membered macrolactone 50 bearing an exo-methylene moiety was obtained in 7% isolated yield (Scheme 16).37 The base lability of 49 in DMF, wherein the vinyl bromide is prone to dehydrohalogenation to give enyne 51, was responsible for the formation of 50. Intramolecular carbometalation of 51 generated 52 which after protonolysis led to the contracted macrolactone 50.
2.3 REACTIONS OF ENOLATES
Palladium-catalyzed coupling between ketone enolate and aryl or vinyl halides is regarded as a viable route for α-arylation or α-alkenylation of ketones.38 Thus the intramolecular coupling reaction of tethered vinyl or aryl halides and ketone enolates provides a useful route to a wide variety of heterocycles.11
The enolate generated from the γ-heteroalkenyl β-keto amides 53 underwent oxidative coupling39 with the tethered alkene when subjected to Pd(MeCN)2Cl2 and Yb(OTf)3 in dry THF under 1 atm O2 as terminal oxidant.40,41 A variety of six-, seven- and eight-membered oxygen heterocycles 54 were synthesized regioselectively from the heteroalkenyl β-ketoamides 53 in excellent yields (Scheme 17). The added Yb(OTf)3 in this reaction acted as a Lewis acid and enhanced the enol formation and the intramolecular attack of the nucleophilic enol toward Pd(II)-activated olefin.41
3. CYCLIZATION VIA C-H BOND FUNCTIONALIZATION
Palladium-catalyzed functionalization of C-H bond42 has undergone a rapid development over the past decade.43 The potentiality of this excellent protocol has proven to be extremely useful for the synthesis of a wide variery of N- and O-heterocycles under mild conditions.44 In general the cyclization via aromatic C-H functionalization proceeds through Pd(IV) intermediate generated by electrophilic palladation on second aromatic or heteroaromatic ring followed by reductive elimination of palladium to afford the heterocycles.45 However, with unactivated alkanes, the functionalization reaction depends on less clearly defined substrate catalyst interactions.46 Both intra- and intermolecular versions of this reaction are discussed in the following section.
3.1 FUNCTIONALIZATION OF ALKANE C-H BONDS
The development of catalytic system for direct functionalization of alkane sp3 C-H bond is of considerable interest.47 The sp3 C-H bond adjacent to amines are realatively activated and can be functionalized under special condition.48 Recently, a new and exciting methodology for the Pd-catalyzed intramolecular alkylations with aryl bromides and chlorides has been developed.50 The Pd(OAc)2/PCy3.HBF4/Cs2CO3/pivalic acid catalyzed reaction, involving sp3 C-H cleavage/functionalization, of ether 55 resulted in complete and clean conversion to 56 in 97%, X = Br and 77 %, X = Cl (Scheme 18).
Density functional theory (DFT) calculations49 indicated that a concerted palladation-deprotonation pathway enabled by the presence of three-center agostic interactions at the transition state50 61, 62 and 63 accounts for the formation of compounds 56a,b. Furthermore, higher ΔG‡ value leading to 59 (reaction at secondary carbon atom) compared to that leading to 58 (reaction at methyl carbon atom) might be responsible for the experimentally observed selectivity for the reaction at the methyl group. The reaction at more remote position leading to 60 is also kinetically and thermodynamically disfavoured (Scheme 19).
3.2 FUNCTIONALIZATION OF AROMATIC C-H BONDS
3.2.1 DIRECT FUNCTINALIZATION REACTIONS
The palladium-catalyzed direct functionalization of aromatic or heteroaromatic C-H bond51 via C-H activation is a versatile way to generate a wide variety of O-heterocycles under mild conditions.52 The added advantage of this exciting protocol is the rapid access to target molecules without any activating group. The intramolecular biarylation of aryl benzyl ether 64 was successfully accomplished by the catalyst generated in situ from Pd(OAc)2 and ligand, 2-(diphenylphosphino)-2'-(N,N-dimethylamino)-biphenyl 6753 with low catalyst loading.54 An excellent yield of dibenzopyran 65 (96%) along with 4% of debrominated product 66 was obtained with 5 mol% Pd(OAc)2 and 10 mol% 67 (Scheme 20).
Fagnou et al. reported that by employing a single catalytic system Pd(OAc)2/P(t-Bu)3-HBF4/K2CO3 in the presence of styrene, compound 68 underwent domino intramolecular Heck reaction and direct arylation reaction to give the products 70a in one pot55 (Scheme 21). A p-acetoxy group on the styrenyl component becomes cleaved under the reaction conditions.
Palladium-catalyzed intramolecular biaryl coupling of phenyl benzoate derivatives 71a,b was investigated.56 The reactions of 71a,b in the presence of Pd(OAc)2, P(n-Bu)3 and K2CO3 in DMA under refluxing condition proceeded smoothly to give benzo[c]chromen-6-ones 72a,b (Scheme 22).
A microwave-assisted, palladium-catalyzed, norbornene-mediated domino process involving two intramolecular ortho alkylations of aromatic C-H bonds followed by an intermolecular Heck reaction was developed.57,58 The interesting feature of this reaction is that two alkyl-aryl bonds and one alkenyl-aryl bond were formed in one-pot. Using various olefins as Heck acceptor and lengthening the bromoalkyl chain of the aryl iodide 73, the syntheses of [5,6,5] ring, symmetrical [6,6,6]- and unsymmetrical [5,6,6; 7,6,7] systems 75 were achieved. The primary differences in their preparation were the reaction time, which was longer for the higher membered ring system (Scheme 23).
For the synthesis of sulfur and nitrogen heterocycles, slight modification of the reaction conditions (increased the catalyst loading to 20 mol%) was required to obtain a modest yield of the cyclized products58,59
An extensive work on the palladium-catalyzed domino three C-H functionalization for the synthesis of polycyclic heterocycles has recently been reported.60 The norbornene-mediated and Pd(OAc)2-catalyzed reaction of 77 and 76 afforded tricylic heterocycle containing benzoxepine moiety 78 in moderate to excellent yield 35-80%. The yield affected when the newly formed ring size increase (Scheme 24).
The addition of aryl boronic acid to phenoxyacetonitrile 80 under the catalysis of cationic palladium complex [(bpy)Pd+(µ-OH)2](-OTf)2 (catalyst B) or [(bpy)Pd+2(Η2Ο)2](-OTf)2 (catalyst C) afforded 3-substituted benzofuran derivatives 81 and the byproduct 82. Nitromethane is the choice of solvent for achieving the desired cyclization (Scheme 25).61
The reactions were performed under two different conditions (condition A and condition B). Aryl boronic acid with electron-donating groups gave better yields than those with electron-withdrawing groups. However, excellent yields were obtained using α-substituted 3,5-dimethoxyphenoxyacetonitrile 80b as the substrate than using 80a. The catalyst C was more effective than the catalyst B in this reaction. The catalyzed reaction is assumed to proceed through the initial generation of ketone 82, which in the presence of cationic palladium species generated the benzofuran derivatives via C-H activation and dehydration. An alternative Friedel Crafts pathway is also probable for the formation of the benzofuran derivatives.
Tanaka et al. reported direct construction of fused aromatic ring systems by palladium-catalyzed “zipper-mode”62 double C-H bond activation process. Treatment of (Z)-3-bromo-2-methylphenyl-(2-bromo-3-phenylprop-2-enyl)ether derivative 83 with a catalytic amount of Pd(OAc)2 and PCy3.HBF4 in the presence of Cs2CO3 in dioxane afforded63 5H-phenanthro[1,10-bc]furan derivative 84 (Scheme 26).
3.2.2 CYCLIZATION VIA 1,4-PALLADIUM MIGRATION
Excellent ability of palladium to insert into unactivated C-H bond is of great interest as it affords wide variety of useful synthetic processes.43 The through-space palladium rearrangement with simultaneous C-H activation provides a novel way to introduce palladium into a specific location within organic molecules. These novel palladium migration process are not only mechanically important but also synthetically useful because they afford an alternative way to introduce a palladium moiety into an organic molecule.
Recently, Larock et al. have applied their vinylic to aryl palladium migration strategy for the synthesis of substituted dibenzofurans,64 although Pd-O coordination would be expected to be much weaker than Pd-N coordination. A series of 3-iodophenyl phenyl ether 85 reacted with various internal alkynes under the reaction conditions Pd(OAc)2, bis(diphenylphosphino)methane (dppm) and CsO2CCMe3 (CsPiv) in DMF at 100 oC to afford two isomeric dibenzofurans 87a and 87b in moderate to excellent yield.65 The efficiency of the reaction is highly dependent on the nature of the group attached to the aromatic ring as more electron rich aromatic ring facilitate the vinyl to aryl palladium migration (Scheme 27).
A novel methodology based on C-H activation via 1,4-palladium alkyl to aryl migration64 followed by intramolecular arylation providing an expedient route to fused ring systems has been reported. Under the optimized reaction conditions aryl halides 88 afforded the compounds 89 and 90 (Scheme 28).66
4. CYCLIZATION OF 1,n-UNSATURATED SYSTEMS: CYCLOISOMERIZATION AND CASCADE ADDITION-CYCLIZATION REACTIONS
Cyclization of 1,n-unsaturated systems catalyzed by palladium has emerged as a convenient way for the preparation of heterocyclic compounds.67 The reactions are broadly classified into two major categories: (a) cycloisomerization reaction68 and (b) cascade addition-cyclization69 reaction. The cyclization reaction usually proceeds through the generation of vinyl or alkyl palladium species either via hydro metallation pathway70 (for cycloisomerization) or via addition of species to carbon-carbon triple bond (cascade addition-cyclization reaction). In both categories the heterocycles are formed via carbon-carbon bond formation.
4.1 CYCLOISOMERIZATION REACTIONS
Palladium-catalyzed cycloisomerization reaction has proven as one of the versatile process to construct cyclic compounds from the acyclic 1,n-unsaturated precursors.71 In general, 1,6-unsaturated system affords five-membered cyclic product. The cycloisomerization of enyne 91 in the presence of palladium source Pd2(dba)3.CHCl3/PPh3/AcOH afforded five-membered heterocycles 92 (Scheme 29).72 By utilizing this protocol, a number of five-membered oxygen heterocycles were synthesized.
Zhang and collaborators extensively investigated the palladium-catalyzed domino cycloisomerization/Suzuki coupling of 1,6-enynes.73 By utilizing Pd(PPh3)4 as catalyst, both electron rich (93a) and electron poor (93b) enynes underwent this cascade cyclization-coupling reaction to afford five-membered heterocycles 94a and 94b with an exo double bond (Scheme 30).
2-Methylenecyclopropanyl ketones 95a owing to the presence of the exo-cyclic C=C bond and the strained cyclopropane, underwent highly selective ring-opening cycloisomerization using Pd(MeCN)2Cl2 as catalyst to afford 4H-pyrans 96 (Scheme 31).74 Interestingly, the reaction of the cyclopropyl ketones 95b in the presence of NaI with the same catalyst afforded substituted furan derivatives 97.
The cycloisomerization of [1’-(1”-cyclopropylideneethyl)cyclopropylmethyl](prop-2”-ynyl)ether 98 having a terminal cyclopropane unit, when subjected to Pd2(dba)3.CHCl3, P(o-tol)3 and AcOH in PhH gave two products, a non cross conjugated triene, seven-membered oxacycle 99 and an intermolecular coupling product trienyne 100, the ratio of which depended on the dilution of the starting material (Scheme 32).75
The oligounsaturated open chain compounds 101 also underwent palladium-catalyzed oligocyclization for the construction of an elegant and efficient assembly of oligocyclic systems.76 Thus enediyne 101 under the same reaction conditions as above afforded cross conjugated tetraene 102 with Z-configured double bond between the two rings (Scheme 33).
A new palladium-catalyzed chemoselective cycloisomerization of cis-2,4-diene-1-als 103 to 4-alkylidene-3,4-dihydro-2H-pyrans 104 and 3-cyclopentenones 105 was reported by Liu et al.77 The reaction was carried out in the presence of Pd(PhCN)2Cl2 in PhMe efficient for efficient construction of 2H-pyranderivatives 104 except only for three substrates [(i) R1 = Me, R2 = Et; (ii) R1 = Me, R2 = i-Pr; (iii) R1 = R2 = -{CH2}4-] where 3-cyclopentenones 105 were formed as major products. This chemoselectivity problem was circumvented by using 2,6-lutidine (5%) as additive and in such cases 2H-pyran derivatives 104 were obtained exclusively in 75-78% yields (Scheme 34).
Mechanistically the cis-2,4-dien-als 103a were expected to follow the pathway 106→107→108→109. The added 2,6-lutidine accelerated the deprotonation reaction of intermediate 108 and preferably gave 2H-pyrans 104a with the change of chemoselectivity (Scheme 35).
4.2 CASCADE ADDITION-CYCLIZATION REACTIONS
The divalent palladium-catalyzed intramolecular enyne coupling reaction initiated by acetoxypalladation78 and halopalladation of alkyne was developed for the construction of a wide variety of five-membered oxygen and nitrogen heterocycles.79 An assays of oxygen- tethered enynes 110 on treatment with Pd(OAc)2 and 2,2’-bipyridine in AcOH at 80-100 oC were converted to five-membered oxygen heterocycles 111 bearing γ,δ-unsaturation in moderate-to-excellent yields (Scheme 36).80 The reactions were highly stereoselective as all the products contained Z-configured double bonds.
An expedient route to the synthesis of stereo-defined α-halomethylene-γ-butyrolactones, lactams and tetrahydrofurans via PdCl2-catalyzed cis-chloropalladation-cyclization of 1,6 enynes 112a in AcOH was developed by Zhang et al.81 The reaction showed excellent stereoselectivity (E/Z > 99/1) as only five membered (E)-113 were obtained in good yields. The halopalladation-cyclization of 1,6-enynes 112b using PdBr2 as catalyst was somewhat less selective as compared to PdCl2 as both (E) and (Z) isomers 114 were produced during cyclization. The trans-bromopalladation to (Z) product is due to the increased polarity of Pd-Br bond (Scheme 37).
Cationic Pd(II)-catalyzed82 intramolecular cyclization of aroylmethyl 2-alkynoates 115 initiated by carbopalladation of alkynes with arylboronic acids was reported by Lu et al.83 In the presence of [(dppp)-Pd(H2O)2](OTf)284 as catalyst the reaction between 115 and arylboronic acids afforded α-alkylidene-β-hydroxy-γ-lactones 116 in excellent yields with E-configured exocyclic double bond. The asymmetric version of this reaction was conducted using Pd(CF3CO2)2/(S,S)-BDPP catalyst-(117) which gave the hydroxylactone 116 with high ee value. It is noteworthy that the reaction proceeded under mild condition without a Pd(II)/Pd(0) redox system with high enantioselectivity. It is proposed that the high Lewis acidity of the Pd center in the cationic species-118 activated the carbonyl group by coordination with the unshared electron pairs on the oxygen atom to facilitate the cyclization and enantioface discrimination of ketones resulting in high ee values (Scheme 38).85
Lewis acid additive showed high influence on the diastereoselectivity of the intramolecular allyl transfer reaction of allenic aldehydes 119 with hexamethylditin catalyzed by (π-allyl)2PdCl2 (Scheme 39).86 Moderate-to-good yields of trans-products 121 were obtained by using B(C6F5)3 as Lewis acid additive. In the absence of any additive cis-products 120 were obtained in good yields. Mechanistically, the cis-isomer formation proceeds through the transition state 122 where as a reverse π-facial selectivity to yield trans-121 may proceed via 123.
5. CYCLOADDITION REACTIONS
5.1. [3+2] CYCLOADDITION REACTIONS
Palladium-catalyzed [3+2] cycloaddition87 reaction is an efficient method to prepare five-membered heterocycles. A number of oxazolidine88 and imidazolidine89 derivatives were synthesized by [3+2] cycloaddition between oxiranes and aziridines with heterocumulenes. An extensive investigation on the palladium-catalyzed [3+2] cycloaddition reaction between 2-(acetoxymethyl)-3-(trimethylsilyl)propene 124a and carbon dioxide was reported by Greco et al.90 The reaction is presumed to proceed via the in situ generation of η3-Pd trimethylenemethane (TMM) complex-(A), the three atom partner, which underwent [3+2] cycloaddition with carbon dioxide to afford γ-butyro lacone 125 as the major product along with acetylated compound 126 (Scheme 40).
5.2 [4+2] CYCLOADDITION (DIELS-ALDER REACTION)
A palladium-catalyzed endo-cycloaddition reaction has recently ben demonstrated. Two equivalent of ethyl vinyl ether, a commonly used dienophile in cycloaddition reactions, was subjected to cycloaddition reaction with o-vinylphenol 128 using Pd[(-)-Sparteine]Cl2 as catalyst to afford the endo-product 129 as a single diastereomer (Schem 41).91 The yield of the product 129 could be increased to 30% when 10% Et3N was added to the reaction. The formation of the compound 129 is assumed to proceed via in situ generation of o-quinone methide13 which undergoes cycloaddition with ethyl vinyl ether in an endo manner.
5.3. [4+3] CYCLOADDITION REACTIONS
First example of palladium-catalyzed [4+3] intramolecular cycloaddition of alkylidenecyclopropanes and dienes has recently been reported.92 The reaction is successfully carried out in the presence of Pd2(dba)3 as catalyst in dioxane using phosphine ligand 133 or 134 and dienylidenecyclopropanes 130. Here the cisoid-diene activated by the presence of electron-withdrawing CO2Et group gave stereochemically rich 5,7-fused bicyclic compounds 131 predominantly along with very little amount of 5,5-fused bicyclic compounds 132. The terminally phenyl-substituted substrate 130b also underwent the cycloaddition providing the adduct 131b. Interestingly, the nonactivated diene also underwent this cycloaddition reaction. The selectivity ratio (seven versus five) was found better using ligand 134 (Scheme 42).
It is envisaged that in the presence of conjugated diene, a π-allyl rearrangement might be responsible for the formation of the seven-membered ring. With transoid diene 130a, cycloaddition reaction afforded the epimeric cycloadduct 131a with poor selectivity of the seven- over the five-membered cycloadduct (Scheme 43).
5.4. [2+2+1] CYCLOADDITION REACTIONS
Transition metal-catalyzed [2+2+1] cycloaddition, in particular Pauson-Khand reaction, between two olefin units with carbon monoxide have been well investigated and is considered to be a general method for the construction of five-membered ring compounds containing carbonyl group.93 In comparison to extensive utilization of Co, Ti, Ni, Ru, Rh, and Ir complexes in Pauson-Khand reaction, very few successful examples of palladium-catalyzed94 Pauson-Khand reaction are available. Yang et al. reported successful application of the palladium catalysis in the [2+2+1] cycloaddition reaction for the construction of bicyclic oxygen and nitrogen heterocycles.95 Different allylpropargyl ethers when subjected to Pauson-Khand reaction utilizing PdCl2 as catalyst and tetramethyl thiourea (tmtu)96 135 as additive in the presence of CO using THF as solvent at 50 oC produced the desired cycloadducts 136 in moderate yields (Scheme 44). In absence of tmtu the Pd(II)-catalyst was precipitated immediately. The yield of the reaction was poor for allylpropergyl ethers compared to allylpropergyl amines.
6. HETEROCYCLIZATION REACTIONS: CYCLIZATION VIA CARBON-HETEROATOM BOND FORMATION
6.1. INTRAMOLECULAR ADDITION OF O-H, C=O BONDS ACROSS ALKENE, ALLENE AND ALKYNE
The applications of Pd catalysis in the formation of carbon-heteroatom bond via intramolecular addition of heteroatom nucleophiles on to carbon-carbon unsaturated bond have turned out to be the most attractive and important tools in the transition metal-catalyzed synthesis of heterocycles.1,2 From the synthetic point of view, these reactions employing various nucleophiles like amines, alcohols, carboxylic acids, esters, amides, carbonyl and imines are very important as the addition reactons can be performed with excellent atom efficiency without any waste formation. In the presence of higher valent Pd(II) the reaction proceeds with the initial formtion of π-complex through the coordination of nucleophile to the C-C unsaturated bond followed by intramolecular nucleophilic addion to the electron deficient unsaturated bond.
6.1.1. ADDITION TO ALKENE
Alkene appended heteroatom nucleophiles have been found to undergo palladiun(II)-catalyzed heterocyclization to produce a variety of heterocyclic compounds.97 Both activated and unactivated double bonds can react with the nucleophiles. The synthesis of a number of five- and six-membered oxygen heterocycles 138 has been reported via oxidative cyclization of a variety of nucleophiles such as phenol onto unactivated double bonds catalyzed by Pd(CF3CO2)2 and pyridine in the presence of molecular oxygen as the sole stoichiometric oxidant in a nonpolar solvent (PhMe) (Scheme 45).98
The asymmetric version of this reaction was successfully carried out by using pre-synthesized Pd(CF3CO2)2 complex and 100 mol% of (-)-sparteine. Under the optimized reaction conditions, phenol 139a was cyclized to provide dihydrobenzofuran (+)-140a’ in 72% yield with 76% ee (Scheme 46).
Quite similarly, 1,7-dien-3-ol 141 on treatment with Pd(PhCN)2Cl2 and BQ as oxidant underwent 6-exo Wacker-type oxidative99 cyclization, followed by alkene migration to the more highly substituted position to afford hexahydrochromene derivative 142 (Scheme 47).100 However, 1,5-dien-3-ols 143a,b under the same reaction conditions reacted rapidly to give aromatized products 144a,b.
The stereo- and regioselectivity of the intramolecular palladium(II)-catalyzed 1,4-oxidation reactions of 1,3-dienes bearing a side chain with a carboxylic or alcoholic nucleophiles 145a and 145b, were highly affected by the presence of additives and solvent composition101 (AcOH/acetone) (Scheme 48). The carboxylic acid 145a gave cis-isomer of δ-lactone 146a as the major product along with 147b when the reaction was conducted in AcOH/acetone (1:4) in the absence and presence of LiCl and LiOAc. However, in the absene of LiCl and LiOAc the cis-isomer was formed exclusively in 53% yield. 1,4-Oxidation of alocohol when performed in AcOH without the addition of MnO2 also followed the same pathway.
The use of chiral bisoxazoline ligands based on binaphthyl102 and biphenyl backbone103 in the Pd(II)-catalyzed enantioselective Wacker-type cyclization of o-allylphenols has been well documented. In a related study, C2-asymmetric bisoxazoline ligand bearing an axis-unfixed biphenyl backbone-151 was utilized in highly enantioselective Pd(II) catalyzed Wacker-type cyclization of 2-allylphenols 149 (Scheme 49). The reactions were catalyzed by Pd(II)-151 complex generated in situ by mixing Pd(CF3CO2)2 with bisoxazoline-151 (Pd/ligand 1:2) and BQ to afford the corresponding chiral 2,3-dihydrobenzofuran 150 with excellent enantioselectivity (90-98% ee).104
Asymmetric cyclization of 2-(3-methylbut-2-enyl)phenol 152 with a chiral ligand and catalytic palladium afforded105 both the desired product 2-isopropenyl-2,3-dihydrobenzofuran 153 and the unwanted pyran derivative 154 (Scheme 50). However, the reaction suffered from poor conversion and poor enantioselectivity. Koning et al. showed that Trost condition for the synthesis of chiral 2-substituted-2-vinyl chromans using chiral ligand (157a)106 and catalytic Pd(dba)2 furnished the required volatile 2-isopropenyl-2,3-dihydrobenzofuran 156a107 from the alcohol 155a. The opposite enantiomer of 156b was also synthesized by similar treatment of 155b with Pd(dba)2 using the opposite Trost chiral ligand (157b).
Trost and collaborators demonstrated a highly efficient and atom economic dual catalytic approach comprised of two distinctly separate reaction strategy (i) Ru-catalyzed intermolecular enyne cross-coupling108 to 1,4-diene followed by (ii) intramolecular nucleophilic trapping of π-alkylpalladium species, in a one-pot reaction sequence for the synthesis of enantio- and diastereo pure O-heterocycles.109 A different catalytic combination Pd2(dba)3.CHCl3, and L-1 were employed for the enantioselective O-heterocyclization. The furan and pyran rings 160 were formed in good yields, with pyran affording the highest enantioselectivity. The enantioselectivity of the furan ring synthesis was slightly improved (79%) by the addition of tetrabutyl ammonium chloride. The seven-membered ring formation was also unsuccessful under this condition due to competitive formation of the triene product (Scheme 51).
It is worthwhile to note that oxygen and nitrogen nucleophiles showed opposite selectivity in the enantioselective heterocyclization reaction. In case of sulfonamide substrates, the enantiodetermining110 matched ionisation111 was followed by fast intramolecular mismatched nucleophilic addition. As the rate of mismatched attack becomes faster than the equilibration of 163, the (S)-stereochemistry is predicted from the (R,R)-ligand though the ring closure involves a mismatched event. On the contrary, if the rate of addition in the cyclization of alcohol nucleophile 161b is slower than equlibration of the palladium-allyl diastereomer, the nucleophlic addition becomes enantiodetermining. Hence, the 1,4-diene 161b undergoes a matched ionization followed by rapid equilibration of 164 and 165. Matched nucleophilic attack through 165 leads to 162b when (R,R)-ligand is utilized (Scheme 52).
Wolfe et al. reported that the reaction between 4-penten-1-ol 166 and 2-naphthylbromide 167 employing a combination of Pd2(dba)3/dpe-phos/NaOt-Bu catalysts presumably proceeded via palladium(aryl) (alkoxide) intermediate112 169 which underwent intramolecular insertion of the alkene into the Pd-O bond113 followed by reductive elimination to afford the 2-naphthyltetrahydrofuran 168 (Scheme 53).114 It is evident from the mechanism that the generation of palladium(aryl)(alkoxide) intermediate 169 and intramolecular insertion of olefin into Pd-O bond are the controlling steps of this catalytic transformation. A precise mechanistic detail of the alkene insertion into the Pd-O bond is depicted in Scheme 53. The most likely pathways involved either direct insertion of alkene via five-coordinated intermediate 174 (Scheme 53, path A)113a or insertion through four-coordinated intermediate 172, formed by an associative ligand substitution process that too proceeds through the five-coordinated complex 170.115
The stereochemical outcome of the carboetherification and carboamination116 reactions of γ-hydroxy 176 and 177 with tethered aryl bromides are highly influenced by the nature of the heteroatom and added ligand. Phosphine ligand controls the syn- versus anti-addition pathways in the catalytic cycle, which allows for stereoselective construction of either of the two possible product diastereomers from a given substrate. For example, the (1S*,2R*)-2-indan-1-yl tetrahydrofuran 178 (51%, syn addition product) was synthesized in 51% and 60% yields from both E- and Z-alkene alcohols (176 and 177) via the Pd2(dba)3/PCy3.HBF4 and Pd2(dba)3/(±)-BINAP-catalyzed syn- and anti-additions respectively (Scheme 54).117 Similarly, both the syn- and anti-additions are observed during the preparation of (1S*,2S*)-isomer 179 from both the alkenes (176 and 177) by changing from chelating dppb to monodentate P[(p-MeO)C6H4]3 keeping the other condition fixed. Substrates bearing tertiary alcohols also behave similarly to afford products of either syn- or anti-addition.
As predicted, the catalyst-induced change in stereochemistry is likely due to a change in the reaction mechanism in the carboetherification reaction. The syn-addition products are assumed to be derived from an unexpected transannular alkene insertion of an 11-membered Pd(Ar)OR complex 181 where as Wacker-type anti-oxypalladation,118 i.e. via the ordered intermediate 184 gives anti-addition product. The stereochemistry around the tetrahydrofuran ring is dictated by non-bonding interactions in the transition state with a preference for pesudoequatorial orientation of substituents. The intramolecular carboamination reaction may proceed via a mechanism similar to that for syn-addition reactions of alcohol substrates involving a transannular alkene insertion of an 11-membered palladium (aryl)(amido) intermediate through transition state 186 or 187 (Scheme 55).
Palladium-catalyzed enantioselective C-3 allylation of 3-substituted-1H-indoles using trialkylboranes was developed by Trost et al.119 Indoles with pendant alcohols 188a,b and allylic alcohol when treated with Pd2(dba)3.CHCl3, 189 as ligand and 9-BBN-(C6H13) as promoter afforded cis-5,5- and 5,6-fused heterocycles 190a-e. The selectivity is highly dependent on the borane reagent used in the reaction as in addition to promoting the ionization of allyl alcohol,120 9-BBN-(C6H13) is directly involved in the enantiodiscriminating step (Scheme 56).
The stereoselective palladium-catalyzed cyclization of THP ether 191 gave four diastereomeric 2,4,6-trisubstituted tetrahydropyrans 192a and 192b of which the 4,6-cis-isomer was produced as the major product.121 The cyclization was carried out employing different alcohol using Pd(PhCN)2Cl2 as catalyst. In the case of 2α and 2β products, major products were 2β isomer and the stereoselectivity (2β:2α) was almost 3:1-2:1 (Scheme 57).
The above reaction may proceed through the formation of hemiacetal intermediate. Four possible conformations (A-D) are assumed which allow the alcohol of the hemiacetal to attack the olefin. The 4,6-cis products are predominantly formed from the chair-like transition state A and B with the allylic alcohol equatorially oriented. The conformations C and D, where the allylic alcohol is in the axial position, causing destabilization by 1,3-diaxial interactions may afford the 4,6-trans product. The hemiacetal reacts intramolecularly with palladium-coordinated olefin shown in A to result in the observed major 2β-isomer 192aa (Scheme 58).
6.1.2. ADDITION TO ALLENE
The transition metal-catalyzed cyclization of functionalized allenes has caught the attention of many synthetic chemists due to its unique reactivity and stereoselectivity.122 Palladium-catalyzed addition of heteroatom nucleophiles to allenes followed by trapping of the intermediate alkenyl palladium intermediate by proton or any electrophilic species has found extensive applications in the synthesis of heterocycles.123
In particular, the reaction between two same or differently functionalized allenes, i.e, homodimerization reaction of functionalized allenes 194 and 195 is of considerable interest. Hasmi et al. reported the homodimerization reaction of allenyl ketone.124 The intermolecular dimerization of 2,3-allenoic acids using PdCl2 as catalyst afforded bicyclic butenolide.125 Moreover, in the heterodimerization the reactions between 2,3-allenoic acids or 2,3-allenamides and 1,2-allenyl ketones, both allenes were cyclized to form products with two different rings.126 An interesting β-hydroxy elimination to dienyl unit was observed during the palladium-catalyzed cyclization of 2,3-allenoic acids in the presence of 2,3-allenols.127 Alcaide and co-workers also reported similar cross-coupling cyclization reactions of R-allenols in the presence of 2,3-allenyl carboxylates (Scheme 59).128
Intermolecular cross couplig reaction between 2,3-allenoic acids 194 and simple allenes 197 by Pd(OAc)2 and BQ afforded highly substituted furan-2(5H)-ones Z-198 (Scheme 60).129
The catalytic cycle that leads to furan-2(5H)-ones is suggested to proceed via initial cyclic oxypalladation of 2,3-allenoic acids with Pd(II) to generate furanonyl palladium intermediates 199 which is trapped by the simple allenes to afford π-allylic intermediates (Scheme 61).
The synthesis of sterically congested bicyclic tetrahydrofurans 201a,b from allenyltetrahydroxyfurans 200a,b by palladium-catalyzed sequential cyclization-coupling reactions has recently been reported.130
The PdCl2-CuCl2-catalyzed reaction showed excellent results for the construction of bicyclic tetrahydrofurans 201a (98%) (Scheme 62).
6.1.3. ADDITION TO ALKYNE
The palladium-catalyzed reaction of alkyne with proximate nucleophilic center represents a useful reaction to afford products via intermolecular nucleophilic attack at the π-palladium alkyne complex, which is generated in situ by the coordination of Pd(II) to carbon-carbon triple bond.1,2 This provides a straightforward approach to the synthesis of a large number of oxygen heterocycles.
A base free palladium-catalyzed heterocyclization of 2-alkylphenols for the synthesis of 2-substituted 3-halobenzo[b]furans 204a,b131 has been reported by employing two different catalytic combinations (condition-A: PdCl2-CuCl2 and condition-B: PdBr2-CuBr2) to afford 2-phenyl 3-bromobenzo[b]furan 204a and 2-phenyl 3-chlorobenzo[b]furan 204b by treating 202 to condition-A and B respectively. The dehalogenated product 203 in 17% yields was obtained as a byproduct when the reaction was performed under condition-B (Scheme 63). It is suggested that HEt3NX, employed in the reaction, may labilize the palladium-carbon σ-bond, thereby converting palladium into a good leaving group.132
The combination of PdI2/KI catalyst has been found to be quite effective for the divergent synthesis of (Z)-1-alkylidene-1,3-dihydroisobenzofurans 206 and 1H-isochromenes 207 via 5-exo-dig or 6-endo-dig cyclization of 2-alkynylbenzyl alcohols 205 (Scheme 64).133
Electronic factors show high impact on the regioselectivity of the palladium-catalyzed hydroalkoxylation of alkynols by controlling the mode of cyclization i.e. 5-exo-dig versus 6-endo-dig.134 This is in contrast to the base promoted cyclization where the cyclization proceeds through 5-exo-dig mode exclusively.135 In the Pd(MeCN)2Cl2 mediated cycloisomerization of sugar acetylenic derivatives 208a-e both 5-exo-dig and 6-endo-dig cyclizations were observed depending on the nature of the substituent on the aromatic ring leading to the formation of products 209 and 210 in poor to excellent yields.136 It is interesting to note that electron-donating group attached to the aromatic ring favors a 6-endo-dig while electron attracting group favors a 5-exo-dig mode of cyclization. The formation of ketals 209a, 210b and 210e occur due to further cyclization of the free hydroxyl group at C6 (Scheme 65).
Palladium-catalyzed cyclization and subsequent conjugate addition of the carbopalladium intermediates have been achieved by successive hydroalkoxylation of 212 with Pd(MeCN)2Cl2, conjugate addition with acrolein and LAH reduction in one-pot to give a mixture of compounds 213 and 214 (Scheme 66).
3,3,3-Trifluoroprop-1-en-2-yl substituted furans 215 were efficiently synthesized by Pd(MeCN)2Cl2-catalyzed cyclization-isomerization of 1,1,1-trifluoro-2-[t-butyldimethylsilyloxy)methyl]-3-alkynylbut-2-en-1-ols (Scheme 67).137 The catalysts like Pd(OAc)2, Pd(PPh3)4, Pd2(dba)3 and Pd(PPh3)2Cl2 were found to be inactive under the reaction conditions. The formation of the furan ring may be easily explained by considering a 5-endo-dig ring closure of the hydroxyl group at the activated alkyne.
Substituted 2,3-dihydrofurans 218 were synthesized by the palladium-catalyzed reaction of propargylic carbonate 217 containing a homopropargylic hydroxyl group with different phenols (Scheme 68).138 The reaction was carried out using Pd2(dba)3.CHCl3-dppf catalytic system in dioxane.
The hydroxypalladation-reductive elimination reaction between o-alkynylcardanol 219 and iodobenzene utilizing Pd2(dba)3, 2,2'-bipyridyl (bpy) and K2CO3 in acetonitrile at 50 oC afforded 2,3-disubstituted benzo[b]furan derivative 220 (Scheme 69).139
Gouverneur et al. devised a palladium(II)-catalyzed Wacker-Heck reaction involving the union of structurally diversed hydroxy-ynones 221 and ethyl acrylate, two electron deficient species. The reaction, generally, proceeds through intramolecular hydroxylation to generate the σ-alkenyl palladium intermediate which may be trapped by olefin intramolecularly.140 An optimization studies for the ring closure of 221 into 222 revealed that the choice of catalyst and additive play an important role on the success of this cascade process. It was observed that except in two cases when the reaction was carried out in 10 mol% of Pd(MeCN)4(BF4)2, K2CO3, PPh3, Cu(OAc)2-H2O or in 10 mol% of Pd(MeCN)4(BF4)2, K2CO3 respectively, the desired product was obtained exclusively along with side product 223 due to the protonolysis of the σ-alkenyl palladium intermediate. Interstingly, addition of LiBr minimised the formation of 223 and increased the yield of 222 to 43%. The best result with an improved 58% yield of 222 was obtained when the reaction was carried out in the presence of 10 mol% of Pd(MeCN)2Cl2, Cu(OAc)2, PPh3 and 20 mol% of LiBr in DME at 65 oC under an atmospheric pressure of oxygen (Scheme 70).
Gabriele et al. recently developed a PdI2-catalyzed new, selective and atom economical methodology for the synthesis of 2-[(dialkylcarbamoyl)methylene]-2,3-dihydrobenzo[1,4]dioxine 225 starting from 2-prop-2-ynyloxyphenols 224 as a mixture of E- and Z-isomers of which Z-isomer was produced predominantly.141 The methodology was also extended to other secondary amines such as morpholine where also Z-isomers were formed as the major products (Scheme 71).
A similar sequential oxidative aminocarbonylation-intramolecular conjugate addition-cyclization was observed during the synthesis of 2-[(dialkylcarbamoyl)methylene]tetrahydrofuran derivatives142 227 starting from pent-4-yn-1-ols 226, under the aforesaid reaction conditions (Scheme 72).
Gabriele et al. recently reported palladium-catalyzed oxidative aminocarbonylation143 of various substituted alkynols for the construction of different heterocyclic skeletons. The product fromation depends on the position of the OH group with respect to the triple bond. The alkynols 228 were reacted with PdI2, KI and amine in dioxane under CO/air (4:1) to afford the 6-hydroxy-2-ynamide 229 along with the formation of furan derivatives 230. The reaction follows two sequential processes – a palladium-catalyzed oxidative aminocarbonylation of the triple bond, followd by a 5-exo-dig cyclization leading to the formation of dihydrofuran derivatives 230 (Scheme 73).144
Bioactive benzofurans 232 were synthesized by sequential homobimetallic145,146 from precursors 231 using Pd(PPh3)4 and PdI2 (homobimetallic catalyst) and KI (excess) as the catalytic system. The major products 232 in this reaction were obtained along with small amount of byproducts 233, 234 and 235 (Scheme 74).
An efficient general synthesis of 2-benzofuran-2-ylacetamides147 238 starting from 1-(2’-allyloxyaryl)-2-yn-1-ols 236, amines 237 and CO with PdI2-PPh3-KI has been developed utilizing sequential homobimtallic concept (Scheme 75).
The scope of the oxidative carbonylation-cyclization protocol was further extended148 by synthesizing benzofurans 240 and benzopyran 241 derivatives (Scheme 76).
Propargylic esters 242 in the presence of Pd(MeCN)2Cl2/BQ underwent oxidative cyclization-carbonylation reaction to afford methoxycarbonylated orthoester 243 (Scheme 77).149 The uncyclized product 244 was obtained only when R1 is p-methoxyphenyl. Very poor yield of the compound 244 was observed only with the benzoate having electron-attracting group attached to the para-position of the aromatic ring.
Wu et al. reported Pd(0)-catalyzed cascade addition-oxidation reaction of 2-alkynylbenzaldehyde 245 with aryliodides in methanol.150 The one-step reaction afforded the regio- and stereoselective synthesis of stereoisomeric methyl-2-(2,2-disubstituted-vinyl) benzoates 247 along with the addition product 246. The exclusive formation of the products 247 was observed only when electron-withdrawing substituent was present in the aromatic ring. This regio- and stereoselective domino process simultaneously coupled the oxidation of an aldehyde to an ester with the hydroarylation of an alkyne to an alkene (Scheme 78).
The reaction of alkenyl aldehydes with methanol in the presence of Pd(II) catalyst to afforded a mixture of five- and six-membered alkenyl ethers.151 However with alkynyl benzaldehyde 248, the cyclization in the presence of Pd(OAc)2, BQ and MeOH gave exclusively six-membered product 249 in moderate yield. In this transformation Pd(OAc)2 acted as dual role catalyst exhibiting both as a Lewis acid for enhancing the electrophilicity of aldehyde as well as a transition metal catalyst for enhancing the electrophilicity of the alkyne bond, for constructing the R-methoxycyclic alkenyl ether from the o-alkynylaryl aldehyde (Scheme 79).
xidative carbonylation of the substrate 250 was carried out with PdI2 catalyst with KI leading to complete conversion of the substrate with the formation of products 251 and the benzoxazine derivative 252. The product 251 is formed by a 6-exo-dig mode of cyclization (Scheme 80).152 The dihydroindolone derivative 252 is formed due to in situ formation of 2-ethynylaniline by cleavage of the amide bond.
This protocol was further extended to the synthesis of tetrahydrofuran derivatives153 with fair diastereoselectivity. The compounds 253 when subjected to oxidative carbonylation-cyclization by PdI2 and KI afforded 2-methoxy-5-[methoxycarbonyl)methylene]tetrahydrofurans 254 and 255 and respectable diastereoselectivity (Scheme 81).
Palladium-catalyzed cyclization of the dihydroxy pyrrole 256 with propargylic carbonate 257 afforded 2-alkylidenepyrrolo[c]-1,4-dioxane derivatives 258 and 259 with around 85:15 regioselectivity (Scheme 82).154 The reactions of alkynes 257a,c,d were conducted using Pd(PPh3)4 and dppb to afford dioxane compounds 258a,c,d in excellent yields along with traces of compounds 259a,c,d where as excellent yields of 259b,e were obtained from alkynes 257b,e.
6.2. INTRAMOLECULAR COUPLING OF OH WITH ARYL HALIDE
Pd-catalyzed cross-coupling between Csp2-halides or triflates represent some of the most powerful and versatile tools for the construction of C-N and C-O bonds in modern synthetic organic chemistry.1 Methodologies for the construction of this type of bonds intramolecularly have become extraordinarily popular, as they represent a very efficient entry into different types of important nitrogenated and oxygenated heterocyclic compounds.2
A palladium-catalyzed highly efficient enantioselective synthesis of chiral substituted 2-methylchromans was developed.155 The product distribution in this reaction is controlled by the steric environment adjacent to the alcohol. Thus from the substrate having both primary and secondary alcohol at the tethering under the Buchwald condition employing Pd(OAc)2/ligand-263 afforded exclusively the six-membered oxacycle 261a (Scheme 83). In contrast, the substrate 260b bearing both tertiary and primary alcohol at the tethering under the same reaction conditions found to afford the chroman along with seven-membered oxacycle 261c. This regioselectivity may be due to the propensity for forming a seven-membered palladacycle 262a, versus an eight-membered palladacycle 262b.
Synthesis of ladder-type furan rings, dibenzo[d,d’]benzo[1,2-b:4,5-b’]difurans 265a-c was achieved156 by Pd(OAc)2-catalyzed intramolecular O-arylation of 264a-c using 2-di-tert-butylphosphino-2’-methylbiphenyl (L1) as ligand and 2,6-di-tert-butyl-4-methylphenol (BHT) as additive. A substantial base and solvent effect on the O-arylation was observed and the compounds 265a-c were obtained. Substitution on the aryl rings affect the reaction yields and in case of electron-withdrawing group this effect increases (Scheme 84).
6.3. CYCLOCARBONYLATION AND CYCLOCARBOXYLATION REACTIONS
The palladium-mediated carbonylation157 and carboxylation158 reactions have been extensively employed in the synthesis of heterocycles. Ma et al. reported a mild and efficient Pd-catalyzed carbonylation of propargylic alcohols with various structural patterns for the synthesis of (Z)-R-chloroalkylidene-β-lactones selectively.159 In the presence of PdCl2-CuCl2 catalytic system and carbon monoxide optically active propargylic alcohols 266 allowed a convenient synthesis of the corresponding (Z)-α-chloroalkylidene-β-lactones 267 with high ee values (Scheme 85).
The reaction between 2-methylbut-3-yn-2-ol 268 and thiophenol in the presence of Pd(OAc)2, PPh3, p-TsOH and CO afforded thiolactonization product 269 as the major product along with mono- and dithiocarboxylation products 270 and 271 (Scheme 86).160
Palladium-catalyzed annulation of internal alkynes by o-iodophenol in the presence of CO employing Pd(OAc)2, pyridine, and n-Bu4NCl afforded coumarin derivative 274161 (Scheme 87).
PdI2-catalyzed oxidative aminocarbonylation of the terminal alkynes 275 has been described as a facile route for the synthesis of five-membered oxygen heterocycles 276.162 Thus, propargyl alcohol and amine when subjected to PdI2, carbon monoxide and oxygen in the presence of secondary amine afforded 4-dialkylamino-5H-furan-2-ones 276 by a sequential oxidative aminocarbonylation-intramolecular conjugate addition-cyclization route (Scheme 88). The reaction also works well when hetero atom is nitrogen giving corresponding N-heterocycles.
Another interesting example of palladium-catalyzed cyclocarbonylation reaction was encountered during the synthesis of the lactone ring of (+)-Ricciocarpin A1 279.163 The lactone ring (+)-278 was constructed via intramolecular carbonyl insertion of the alcohol (-)-277 in the presence Pd(OAc)2 and triphenylphosphine. Reduction of compound 278 with sodium borohydride resulted in the formation of (+)-ricciocarpin 279 (Scheme 89).
It was observed that palladium-catalyzed cyclocarbonylation and thiocarbonylation reaction of enynols with thiols gave usually thioester-containing 6-membered-ring lactones 281 using THF as the solvent.164 Similar reaction of enynols 280 with a variety of thiols when conducted with 500 psi carbon monoxide in the presence of a catalytic amount of Pd(OAc)2 (2 mol%) and PPh3 (8 mol%) in ionic liquid [bmim]PF6 or [bmim][Tf2N], exhibited excellent chemoselectivity and resulted in the formation of the monocarbonylated product 281 (Scheme 90).165
The reaction of chiral propargylic carbonates 282a,b was shown to proceed in a highly enantiospecific manner to give chiral cyclic carbonates 283 via an overall cascade chirality transfer process (Scheme 91).166
The enantiospecificity of this cascade reaction, performed by using Pd2(dba)3.CHCl3 as catalyst is highly dependent on the choice of phosphine ligand. Thus, by using dppe as a ligand enantiomerically enriched chiral propargylic carbonate 282a afforded both cyclic carbonate (Z,S)- and (E,R)-283aa in a 10:1 ratio with 95% enantiomeric excess for each product. The same reaction with (S)-282a in the presence of dppp selectively afforded (E,R)-283aa without any loss of enantiomeric purity or dppe as ligand in the presence of phenol as nucleophile afforded both (Z,S)- and (E,R)-283 in high enantiomeric purity. A similar highly enantiospecific cascade reaction occurred between 282a,b and various phenols to afford the corresponding cyclic carbonates (Z,S)- and (E,R)-283ab-ae.
7. CYCLIZATION VIA CASCADE CARBON-CARBON/CARBON-HETEROATOM BOND FORMATION: HETEROCYCLIZATION REACTIONS
7.1 HETEROCYCLIZATION REATIONS WITH ALKYNES
Palladium-catalyzed intermolecular heterocyclization of alkynes has emerged as an accomplished route to he synthesis of large varieties of heterocycles.167 Depending upon the nature of the alkynes employed, the annulation proceeds through two distinctively different mechanistic pathways. For terminal alkynes, widely popular Sonogashira coupling and base mediated cylization approach afford the heterocycles.168 However, intermolecular addition of the nucleophile to C-C triple bond followed by intramolecular reaction appeared to be a convenient pathway for the heterocyclization with internal alkynes.169
7.1.1. REACTIONS WITH TERMINAL ALKYNES: SONOGASHIRA COUPLING-CYCLIZATION REACTIONS
3-Iodothiophene-2-carboxylic acid 284 reacted smoothly with terminal alkynes 285 in the presence of Pd(PPh3)2Cl2-Et3N-CuI affording predominantly 5-substituted 4-alkynylthieno[2,3-c]pyran-7-ones 286 in good yields along with a minor amount of 5-substituted thieno[2,3-c]pyran-7-ones 287.170 The reaction showed high regioselectivity as no isomeric thieno[2,3-c]furan-6-one resulting from 5-exo-dig cyclization was detected. Among the different solvents used for the reaction of Pd(PPh3)2Cl2 catalysts, DMF was found to give better results (Scheme 92).
An interesting substituent effect on the mode of cyclization during palladium-catalyzed domino Sonograshira cross coupling cyclization of o-iodocardanol 288 was reported. Substituent effect was found to be very effective for the synthesis of lipophilic benzo[b]furans 290 from terminal alkynes and o-iodophenols.139,171 With (2-methoxyethoxy)methyl protected 2-methylbut-3-yn-2-ol utilized as terminal alkyne precursor, chroman-4-one 292 was produced presumably via initially formed cross-coupling intermediate, addition of water to carbon-carbon triple bond, an eliminition step and conjugate addition of the phenolic oxygen to the resultant α,β-unsaturated carbonyl compound. However, 2-methylbut-3-yn-2-ol afforded the benzo[b]furan derivative 290 low yield along with the acetophenone derivative 291 as the major product (Scheme 93). In the latter case, instead of conjugate addition, removal of hydroxy group as acetone through retroaldol reaction led to the formation of acetophenone derivative 291. Apprarently, the addition of water to the C-C triple bond of the coupling intermediate in these cases is faster than the desired intramolecular cyclization onto the phenolic oxygen. An improved reaction conditions utilizing Pd(PPh3)2Cl2 (0.02 equiv), CuI (0.04 equiv), Et2NH (2 equiv) in DMF at 60 oC was found to be effective for the preparation of 2-substituted benzo[b]furans in good-to-excellent yields.
Regio- and stereocontrolled synthesis of stannylated butenolides was achieved through palladium-catalyzed domino cross-coupling/cyclization reaction of tributylstannyl (Z)-3-substituted iodopropenoate with tributylstannylacetylene.172 Pd(PPh3)4 is an efficient catalyst for the preparation of (E)-5-tributylstannylmethylidene-5H-furan-2-ones 294a-e (Scheme 94).
(2E)-2,3-Difluoro-3-iodoacrylic acid 295 underwent coupling-cyclization with terminal alkyne under co-catalysis of Pd(PPh3)2Cl2 (2 mol%) and CuI (5 mol%) to provide 3,4-difluoro-6-substituted-2-pyrone 296 in 43-71% yields.173 The formation of the compound 296 (when R = Ph) was assumed to involve in situ generation of ynenoic acid. Interestingly, exclusive formation of the six-membered pyrone ring may be due to the partial polarization of the triple bond by the two fluorine atoms facilitating the attack of the carboxylate anion at the partial positive charged carbon atom (Scheme 95).
Heterogenous catalyst, Pd/MgLa mixed metal oxide has been utilized to conduct the copper free Sonogashira coupling between o-halogenated phenols and phenylacetylene.174 The reaction is efficient only in the case of 2-iodophenol giving the 2-arylbenzofuran derivative 299 while with bromo- and chloro-compounds due to low reactivity afforded the desired benzofuran derivative in low yield (Scheme 96).
One pot synthesis of diverse 2-substituted furo[3,2-c]quinoline derivatives175 based on domino coupling-cyclization176 between 3-iodoquinoline-4-ones 300 and terminal acetylenes afforded the compounds 301 in excellent yields (Scheme 97).
7.1.2. REACTIONS WITH INTERNAL ALKYNES
Double annulation of bis(allyloxy)bis(alkynyl)benzene 302 in the presence of Pd2(dba)3.CHCl3 catalyst and PPh3 as ligand afforded the tetrasubstituted benzodifuran 303 in 71% yield along with a trace amount of 304177 (Scheme 98).
7.2 HETEROCYCLIZATION REACTIONS WITH ALKENES
Pd(II)-catalyzed oxidative annulation of alkenes with oxygen nucleophiles, commonly known as Oshima Utimoto reaction,178a has received much attention in synthetic organic chemistry. The use of Pd(II)-catalyst along with large amount of Cu(II) as promoter of the catalyst in the oxidative addition of allylic alcohol to olefin has been well established.178 Mechanistically, the reaction proceeds via oxypalladation followed by 5-exo cyclization and β-hydrogen elimination to give the cyclic ether 309 (Scheme 99).
Recently, Pd(II)-Cu(II) catalysis has been utilized in the synthesis of 2-alkoxytetrahydrofurans 310 from allylic alcohols and vinyl ethers. Using catechol as an activator, the reaction afforded cyclic ethers in moderate-to-excellent yields (Scheme 100).179 The role of catechol may be in (i) enhancement of catalyst stability by construction of a Pd-Cu heterometallic species bearing catechol as ligand of Cu (II) effective capture of O2 by its activator by the Cu-catechol moiety. The occurance of 5-exo cyclization of the oxypalladium intermediate 307 rather than 6-endo cyclization may be due to conformational compatibility of the oxypalladation adduct 307 for undergoing the cyclization towards the internal alkene, which must coordinate to Pd(II). It has been observed that the rate of cyclization depends on the Ph-group attached and thus the presence of electron-withdrawing group retards the reaction.
Palladium-catalyzed stereoselective Oshima-Utimoto reaction was successfully achieved for the construction of five-membered oxacycle 312 employed for the preparation of lactone ring in the asymmetric synthesis of (-)-11R,1,3-dihydroxanthatin.180 The allylic alcohol 311 on treatment with Pd(OAc)2/Cu(OAc)2 catalytic system in acetonitrile afforded the tetrhydrofuran derivative in 68% yield and with >12:1 1,2-stereoinduction (Scheme 101). Presumably, the Pd-catalyzed process proceeded via the chair like transition state 313 and was found to result in a separable 1:1 mixture of α and β anomers. The target compound was subsequently synthesized in few steps in 66% yield.
The allylic alcohol 314 and vinyl ether 306b, when subjected Oshima-Utimoto reaction under the conditions 10 mol% Pd(OAc)2, 2.5 equiv Cu(OAc)2 in MeCN at 55 oC for 15 h afforded the furan derivative 315 in 69% yield with >15:1 1,2 diastereoinduction (Scheme 102).181
Stereocontrolled synthesis of tricyclic core cis-lactone 317 of the natural product (-)-panacene 765 via intramolecular alkoxycarbonylation-lactonization protocol was achieved182 by Pd(OAc)2-catalyzed domino cyclization of the phenol 316. The natural (-)-panacene 318 was then synthesized from the tricyclic lactone 317 (Scheme 103).
Catalytic amounts of Pd(II), H4PMo11VO40.28H2O (HPMo11V), and Lewis acid-like CeCl3183 were found to be very effective for the construction of ethyl-5-methyl-3-furoate 321 in 86% yield from ethyl acrylate 319 and propanaldehyde 320.184 The reaction was conducted in the presence of 1 atmosphere of oxygen using solvent mixture (MeOH-AcOH). It is proposed that the acrylate initially formed acetal 322 or its synthetic equivalent 323 may undergo aldol type condensation with 320 by CeCl3 to give an α,β-unsaturated carbonyl condensate 326 which on subsequent enolization by Pd(II) followed by intramolecular cyclization may produce ethyl furoate 321 through Pd-H elimination from a dihydrofuran intermediate 329. Finally, the reduced Pd(0) was reoxidized to Pd(II) by the action of HPMo11V/O2 reoxidation system (Scheme 104).185
7.3. HETEROCYCLIZATION REACTIONS WITH DIENES
Larock et al. reported an efficient approach to biologically interesting dihydrofuroflavonoids 332 via palladium-catalyzed annulation of 1,3-dienes by o-iodoacetoxyflavonoids 330.186 The regioselective reaction was accomplished by employing catalyst Pd(dba)2/dppe and base Ag2CO3 in dioxane/H2O as solvent (4:1) to afford compound 332 (Scheme 105).
In the case of cyclic 1,3-dienes 334, an increase in the ring size of the cyclic 1,3-diene led to a significantly lower yield of annulation products 335 (Scheme 106).187
8. PALLADIUM-CATALYZED MULTI-COMPONENT REACTION
Recently there is flurry of activities in the development of efficient synthetic procedure that can affect multiple chemical reactions in a one-pot event.188, 189 The ability of the palladium-catalysts to affect multicomponent reactions has enable to rapidly generate the libraries of heterocyclic compounds.1,190 The reactions are highly chemo-, regio- and stereoselective and show excellent tolerence of functional groups.
8.1. PALLADIUM-CATALYZED THREE-COMPONENT REACTION
Three-component reaction between 1,3-diketone with terminal alkyne in the presence of carbon monoxide was effected in the presence of Pd(PPh3)2Cl2 as catalyst and dppp as ligand in ionic liquid [bmim][Tf2N] gave highly substituted endocyclic enol lactones 338 in moderate-to-good yields (Scheme 107).191 The reaction is assumed to proceed via the generation of alkoxy- and acyl palladium complex 339 and 340. Regioselective intramolecular acylpalladation of the latter and subsequent reductive elimination may produce the linear precursor 341 and regenerate the palladium(0) species. An intramolecular cyclization of the vinyl acetate with the activated double bond may then give the 6-membered enol lactone 338.
Meijere et al. developed a Pd(0)-catalyzed novel multicomponents queuing cascade reaction for the construction of spiro-annulated five- and six-membered heterocycles.192 The three-component cross coupling reactions of bicyclopropylidene 342 with with o-iodophenols were investigated using various catalytic conditions. However for o-iodophenol this condition favored the formation of 345 (24%) over 344 (11%). The formation of the product 345 was avoided by protecting the OH group with TMS, which gave the product 344 (29%) (Scheme 108). The reaction also works well when heteroatom is nitrogen affording corresponding nitrogen heterocycles.
A palladium-catalyzed one-pot three-component reaction was developed for the synthesis of 6H-dibenzopyran derivatives.193 The reaction of aryl iodide, o-bromophenol and methyl acrylate in the presence of Pd(OAc)2, and norbornene as catalyst and K2CO3 as a base in DMF at 80 oC for 24 h under nitrogen afforded the compounds 348 (Scheme 109).
Recently, a novel three-component reaction affording new dihydrobenzofurans has been developed.194 The domino reaction of 2-iodophenol, methyl bromomethylacrylate and phenylboronic acid in the presence of Pd(OAc)2, K2CO3 and n-Bu4NCl in DMF provided 3,3-disubstituted 2,3-dihydro benzofuran 350. By employing a single catalytic system this reaction proceeded through allylic alkylation of 2-iodophenol followed by exo-trig cyclization via carbopalladation of the acrylate and then Suzuki cross coupling of the resulting alkylpalladium species (Scheme 110).
9. MISCELLANEOUS
9.1 INTRAMOLECULAR HECK REACTION
The syntheses of the macrocyclic taxoids195 352a and 352b from 351a and 351b were achieved by using the catalysts Pd(PPh3)4 and Pd2(dba)3/AsPh3 respectively by an exclusive exo-cyclization mode (Scheme 111). Likewise, the macrocycles 354 were also obtained in good yields.
Synthesis of a range of highly constrained cyclic tripeptides, a mimic of vancomycine’s carboxylate-binding pocket, via intramolecular Sonogashira coupling as the ring-closing reaction was reported by Liskamp and co-workers.196 The linear precursors 355a-c when subjected to Pd(PPh3)4, CuI and Et2NH furnished the cyclic peptides 356a-c. Interestingly, increasing steric bulk (leucine, 355c) or the absence of a turn-inducing motif (glycine, 355a) resulted in a lower yield of cyclization and formation of undesired byproducts (nonstrained diynes 357) and cyclic dimer 358 were obtained along with the strained 15-membered peptide macrocycles 356 (Scheme 112).
9.2. CYCLOADDITION REACTIONS
The behavior of oxime ether in the presence of palladium catalyst is quite interesting. It has been observed that the oxime O-allylether 359 in the presence of Pd(MeCN)2Cl2 as catalyst in CHCl3 at 60 oC underwent formal [2,3] sigmatropic rearrangement to generate unstable nitrone intermediate 360 which could be trapped by 1,3-dipolar cycloaddition with dipolarophiles like maleimide 361 to give the cycloadduct 361a (Scheme 113).197 This reaction is nonselective as diastereomeric mixture is obtained.
To test the intramolecular nature of the nitrone formation, a cross over experiment was set to allow scrambling of the partners (Scheme 114). However, after 72 h the formation of products 361b and 363 clearly indicate that the nitrone formation proceeds completely in an intramolecular fashion.
9.3. INTRAMOLECULAR ADDITION OF N-H BONDS ACROSS ALKENE
Domino N-arylation/cyclization/C-arylation of O-homoallylhydroxylamines 364 with aryl bromides in the presence of Pd2(dba)3, x-phos and NaOt-Bu afforded cis-disubstituted isoxazolidine derivatives 366 (Scheme 115).198
A highly diastereoselective Pd(0)-mediated cascade C-C and C-N bond formation was achieved in a one-pot event for the synthesis of isoxazolidine derivatives 368.199 The reactions of O-homoallyl hydroxylamines 367 with aryl iodides were best carried out in the presence of Pd2(dba)3, P(o-tol)3 and Cs2CO3 in refluxing PhMe for 20 h to afford the isoxazolidine derivatives 368 together with normal Heck coupling adducts 369 (Scheme 116).
The effect of base and reaction time on the enantioselectivity of the palladium-catalyzed asymmetric domino allylic substitution of (Z)-1,4-diacyloxy- and (Z)-1,4-bis(alkoxycarbonyloxy)-2-butene 370a-c using 2-(phosphinophenyl)pyridine (375)200 as chiral ligand have been reported.201 All the reactions were carried out using [Pd(C3H5)Cl] as catalyst in CH2Cl2 at room temperature employing various nucleophiles. For 2-(benzylamino)phenol and 2-(benzylamino)ethanol, excellent yields and good enantioselectivities were observed when the reaction was carried out for 72 h using KF as a base where as the reaction with 1,2-bis(benzylamino)ethane was best effected with K2CO3 as the base with good enantioselectivity. In all the cases the products 373 and 374 were formed with R-configuration (Scheme 117).
Formation of the products 373 and 374 proceeds via π-allylpalladium complexes. The possible diastereomeic π-allylpalladium complexes (376, 377, 379 and 380) are assumed to be in rapid equilibrium. The strong trans-effect of phosphorous causing preferential attack of the carbon trans to P-atom along with rotation of allyl system in the direction causing less steric repulsion during nucleophilic attack might be responsible for exclusive formation of the R-product via intermediate 378 (Scheme 118).
Palladium(0)-catalyzed regiospecific domino allylation of 2-aminophenols with 2-butene-1,4-diol produced two cyclized compounds 384 and 385 in comparable yields.202 The reaction was carried out in the presence of Pd(OAc)2, PPh3 and Ti(Oi-Pr)4. Interestingly, formation of the compound 385 was not observed in case of aromatic ring containing strongly electron-withdrawing group (NO2). The addition of Ti(Oi-Pr)4 in this reaction promoted the allyl-OH bond cleavage, thus enhancing the rate of the reaction and the yield (Scheme 119). The same reaction was also applicable to the diamino substrates.203
The reaction of 2-aminophenols with cyclic meso-allylic diacetates 387 catalyzed by Pd(dba)2/PPh3 gave phenoxazines 388 (Scheme 120).204 Based on well-known π-allylpalladium chemistry it is assumed that the relative stereochemistry of the morpholine ring and cyclopentene and / or the cyclohexene ring should be cis.
9.4. INTRAMOLECULAR COUPLING OF NH AND OH WITH ARYL HALIDE
The Pd-catalyzed intramolecular arylamination and aryl etherification on carbohydrate derived substrates by using bulky biarylphosphine ligands has been reported.205 A variety of highly functionalized cis-fused tricyclic furobenzoxazocines 390 were prepared from D-glucose-derived substrates 389 utilizing Pd2(dba)3 as catalyst and (±)-BINAP as ligand. KOt-Bu + K2CO3 was found to give excellent results for the bromo substrates 389 while the iodo substrate afforded better result with Cs2CO3 (Scheme 121).
An efficient synthetic protocol for the synthesis of a wide range of N-arylated 5-, 6- and 7-membered heterocycles involving palladium-mediated sequential intramolecular and intermolecular arylamination reactions has been reported.206 The use of an in situ generated Pd(0) catalyst associated to N-heterocyclic carbene, N,N-bis(2,6-diisopropylphenyl)dihydroimidazol-2-ylidine (SIPr) as ligand, NaOt-Bu and Pd(OAc)2 was found to be effective. This diarylation protocol was also applied to the synthesis of benzoxazines 392a and benzoxazepines 392b from 391 by employing the same conditions (Scheme 122).
Ma et al. reported the synthesis of oxazepine ring 394 containing pyridazinone moiety via palladium catalyzed intramolecular coupling of an aryl bromide with an alcohol 393.207 The pyridazinone when treated with Pd(OAc)2 and (dppf) afforded the product 394. The reaction is assumed to proceed via palladium oxapalladacycle followed by C-O bond forming reductive elimination (Scheme 123).
Palladium-catalyzed intramolecular cycloamination strategy for the synthesis of an assembly of oxazepine 396a and thiazepine ring 396b systems208 were carried out using Pd2(dba)3, P(t-Bu)3 and NaOt-Bu or with K2CO3 in PhMe. In general this worked well for the substrates, 2-[(2-bromobenzyloxy]aniline hydrochloride 395a and 2-[(2-bromobenzyl)thio]aniline hydrochloride 395b to give the desired substituted oxazepine and thiazepine tricyclic core (Scheme 124).
9.5. CYCLOCARBONYLATION AND CYCLOCARBOXYLATION REACTIONS
Gabriele et al. reported209,210 that a combination of PdI2 and KI in DME exhibited excellent catalytic efficieny in the oxidative cyclocarbonylation reaction of β-amino alcohols and 2-aminophenol (Scheme 125).
9.6. HETEROCYCLIZATION REACTION WITH ALKYNE
A palladium-catalyzed one-pot heterocyclization for the construction of polycyclic indole derivatives 400 starting from 2-chloroanilines 399 bearing tethered acetylenes was achieved211 by using Dt-BPF as ligand, K2CO3 as base and NMP as solvent at 130 oC (Scheme 126). The reaction is also applicable when X = nitrogen affording corresponding nitrogen heterocycles.
9.7. PALLADIUM-CATALYZED FOUR-COMPONENT REACTION
Gabriele et al. reported an efficient concatenation of aminocarbonylation and cyclocarbonylation for the synthesis of 2-oxazolidinones 403a,b.212 The one-pot method consists of the reaction of α,α-disubstituted 2-ynylamines with CO, O2, and morpholine in the presence of catalytic amounts of PdI2 in conjunction with KI and H2O (PdI2/KI/1/2/H2O molar ratio1:10:100:500: 500), in DME as the solvent at 100 oC under 20 atm of a 4:1 mixture of CO/air to afford 403 in excellent yield. The reaction in the presence of secondary amine like piperidine and diethyl amine also proceeded smoothly with good yield of oxazolidinones. In the case α-monosubstituted propargylamine, such as benzyl-[1-(1-ethylpropyl)prop-2-ynyl]amine under the same reaction conditions, the initially formed oxazolidinone underwent a spontaneous double bond shift with the formation of 3H-oxazol-2-one derivative 403b (Scheme 127).
9.8. CLAISEN REARRANGEMENT
Pd-catalyzed domino Claisen rearrangement213/oxidative cyclization of allyl aryl ethers 404 into benzofurans 405 was investigated by Youn et al.214 The scope of this sequential process was tested with different allyl aryl ethers in the presence of Pd(MeCN)2Cl2 as catalyst, Na2CO3 as base and BQ as oxidant in dioxane to afford benzofuran derivatives. This reaction is facile for the electron rich arenes where as for the electron deficient arenes higher catalysts loading and increased temperatures are required (Scheme 128).
9.9. ELECTROCYCLIZATION REACTION
Stoltz et al. developed a diastereoselective tandem Stille-oxa-electrocyclization reaction to generate the pyran ring.215 A combination of catalytic Pd(PPh3)4, CuI and DMF with exclusion of light facilitated coupling of 407 with 406 to yield the furan appended tricyclic spiro compound 409 (Scheme 129).
The presence of light and CuI is very much essential for this domino Stille-oxa-electrocyclization reaction. However, in the presence of light only Stille coupling occurs along with cis-trans isomerization, resulting in the formation of trans-dienone 410.
10. CONCLUSION
Synthetic strategies involving palladium is highly desirable due to its versatility in catalyzing a broad spectrum of chemical transformations under mild conditions and tolerance of functional groups. Heterocyclic compounds are widely used in chemical, pharmaceutical and agricultural industries. The applications of palladium catalysis in the synthesis of heterocyclic compounds are now at its premium level of achievement. In this review, we have demonstrated an enclave of novel and exciting palladium chemistry, which has now become a powerful tool in the synthesis of heterocycles.
Strategically, palladium-catalysis in the synthesis of oxygen heterocyclic compounds is very important, as this constitute an efficient strategy for carbon-carbon and carbon-heteroatom bond formation. Although significant contributions have already been made in this area, new challenges are continuously forthcoming. Proper tuning of the reaction conditions will lead to the development of new methodologies that can widen the scope of the application of palladium-catalysts even for the synthesis of complex biologically active molecules. We hope that this review will be useful to the synthetic chemists in general and heterocyclic and medicinal chemists in particular.
11. ACKNOWLEDGEMENTS
We thank the DST (New Delhi) for financial assistance. Two of us (B. C. and S. S.) are grateful to the CSIR (New Delhi) for a senior research fellowship.
References
1. (a) J. Tsuji, Palladium Reagents and Catalysts – New Perspectives for the 21st Century; John Wiley & Sons: New York, 2004; CrossRef (b) Palladium in Organic Synthesis; J. Tsuji, Ed.; Springer: Berlin, 2005; (c) In Handbook of Organopalladium Chemistry for Organic Synthesis; E. Negishi, Ed.; Wiley & Sons: New York, 2002, 2169.
2. (a) E. Negishi and L. Anastasia, Chem. Rev., 2003, 103, 1979; CrossRef (b) L. A Agrofoglio, I. Gillaizeau, and Y. Saito, Chem. Rev., 2003, 103, 1875; CrossRef (c) I. Nakamura and Y. Yamamoto, Chem. Rev., 2004, 104, 2127; CrossRef (d) S. Cacchi and G. Fabrizi, Chem. Rev., 2005, 105, 2873; CrossRef (e) G. Zeni and R. C. Larock, Chem. Rev., 2006, 106, 4644; CrossRef (f) E. M. Beccalli, G. Broggini, M. Martinelli, and S. Sottocornola, Chem. Rev., 2007, 107, 5318; CrossRef (g) D. Conreaux, D. Bouyssi, N. Monteiro, and G. Balme, Curr. Org. Chem., 2006, 10, 1325; CrossRef (h) S. Cacchi, G. Fabrizi, and A. Goggiamani, Curr. Org. Chem., 2006, 10, 1423; CrossRef (i) B. Gabriele, G. Salerno, and M. Costa, Synlett., 2004, 2468; CrossRef (j) G. Zeni and R. C. Larock, Chem. Rev., 2004, 104, 2285. CrossRef
3. (a) A. B. Dounay and L. E. Overman, Chem. Rev., 2003, 103, 2945; CrossRef (b) H. P. Bell, H. Ila, and L. F. Tietze, Chem. Rev., 2004, 104, 3453; CrossRef (c) P. J. Guiry and H. A. McManus, Chem. Rev., 2004, 104, 4151. CrossRef
4. (a) L. F. Tietze and F. Haunert, In Stimulating concepts in chemistry, F. Vögtle, J. F. Stoddart, and M. Shibasaki, Eds., Wiley-VCH: Weinheim, 2000, 39; CrossRef (b) L. F. Tietze and A. Modi, Med. Chem. Rev., 2000, 20, 304; (c) C. E. Garret and K. Prasad, Adv. Synth. Catal., 2004, 346, 889. CrossRef
5. (a) G. Dyker, In Handbook of Organopalladium Chemistry for Organic Synthesis, E. Negishi, Ed.; John Wiley & Sons: New York, 2000, IV.2.2.2,1255; (b) L. F. Tietze, H. Schirok, and M. Wöhrmann, Chem. Eur. J., 2000, 6, 510; CrossRef (c) R. Grigg and V. Savic, Chem. Commun., 2000, 873; CrossRef (d) L. F. Tietze, K. Thede, R. Schimpf, and F. Sannicolό, Chem. Commun., 2000, 583; (e) L. Bhat, A. G. Steinig, R. Appelbe, and A. de Meijere, Eur. J. Org. Chem., 2001, 1673; CrossRef (f) K. Wakabayashi, H. Yorimitsu, and K. Oshima, J. Am. Chem. Soc., 2001, 123, 5374. CrossRef
6. R. F. Heck, Org. React., (N.Y.) 1982, 27, 345.
7. A. Jutand, Eur. J. Inorg. Chem., 2003, 2017. CrossRef
8. K. Fagnou and M. Lautens, Angew. Chem. Int. Ed., 2002, 41, 26. CrossRef
9. (a) U. Anwar, A. Casaschi, R. Grigg, and J. M. Sansano, Tetrahedron, 2001, 57, 1361; CrossRef (b) A. de Meijere and S. Bräse, J. Organomet. Chem., 1999, 576, 88; CrossRef (c) G. Poli, G. Giambastiani, and A. Heumann, Tetrahedron, 2000, 56, 5959; CrossRef (d) M. Lautens, E. Tayama, and C. Herse, J. Am. Chem. Soc., 2005, 127, 72. CrossRef
10. (a) M. Bauer and M. E. Maier, Org. Lett., 2002, 4, 2205; CrossRef (b) G. A. Holloway, H. M. Hügel, and M. A. Rizzacasa, J. Org. Chem., 2003, 68, 2200; CrossRef for Heck Macrocyclization see, (c) K. Akaji, K. Teruya, M. Akaji, and S. Aimoto, Tetrahedron, 2001, 57, 2293; CrossRef (d) V. Balraju, D. S. Reddy, M. Periasamy, and J. Iqbal, Tetrahedron Lett., 2005, 46, 5207; CrossRef for Suzuki Macrocyclization (e) W. Li, and K. Burgess, Tetrahedron Lett., 1999, 40, 6527; CrossRef for Stille Macrocyclization (f) H. Deng, J.-K. Jung, T. Liu, K. W. Kuntz, M. L. Snapper, and A. H. Hoveyda, J. Am. Chem. Soc., 2003, 125, 9032. CrossRef
11. (a) D. Solé, L. Vallverdú, E. Peidró, and J. Bonjoch, Chem. Commun., 2001, 1888; (b) D. Solé, L. Vallverdú, and J. Bonjoch, Adv. Synth. Catal., 2001, 343, 439; CrossRef (c) O. Gaertzen and S. L. Buchwald, J. Org. Chem., 2002, 67, 465; CrossRef (d) D. Solé, L. Vallverdú, X. Solans, M. Font-Bardı´a, and J. Bonjoch, J. Am. Chem. Soc., 2003, 125, 1587; CrossRef (e) J. Bonjoch, F. Diaba, G. Puigbó, E. Peidró, and D.Solé, Tetrahedron Lett., 2003, 44, 8387; CrossRef (f) D. A. Culkin and J. F. Hartwig, Acc. Chem. Res., 2003, 36, 234. CrossRef
12. (a) T. Honda, H. Namiki, and F. Satoh, Org. Lett., 2001, 3, 631; CrossRef (b) R. Imbos, A. J. Minnaard, and B. L. Feringa, J. Am. Chem. Soc., 2002, 124, 184; CrossRef (c) R. Ferraccioli, D. Carenzi, and M. Catellani, Synlett, 2002, 1860; (d) D. Solé, F. Diaba, and J. Bonjoch, J. Org. Chem., 2003, 68, 5746. CrossRef
13. (a) Y. Fukuyama, H. Yuasa, Y. Tonoi, K. Harada, M. Wada, Y. Asakawa, and T. Hashimoto, Tetrahedron, 2001, 57, 9299; CrossRef (b) B. M. Trost, J. Org. Chem., 2004, 69, 5813; CrossRef (c) Q. Huang, A. Fazio, G. Dai, M. A. Campo, and R. C. Larock, J. Am. Chem. Soc., 2004, 126, 7460. CrossRef
14. R. C. Larock and S. Babu, Tetrahedron Lett., 1987, 28, 5291. CrossRef
15. (a) M. T. Reetz and J. G. de Vries, Chem. Commun., 2004, 1559; CrossRef (b) M. B. Thathager, J. E. ten Elshof, and G. Rothenberg, Angew. Chem. Int. Ed., 2006, 45, 2886; CrossRef (c) M. B. Thathager, P. J. Kooyman, R. Boerleider, E. Jansen, and C. J. Vlsevir, Adv. Synth. Catal., 2005, 347, 1965; CrossRef (d) C. C. Cassol, A. P. Umpierre, G. Machado, S. I. Wolke, and J. Dupont, J. Am. Chem. Soc., 2005, 127, 3298. CrossRef
16. P. Liu, L. Huang, Y. Lu, M. Dilmeghani, J. Baum, T. Xiang, J. Adams, A. Tasker, R. Larsen, and M. M. Faul, Tetrahedron Lett., 2007, 48, 2307. CrossRef
17. C. Gutler and S. L. Buchwald, Chem. Eur. J., 1999, 5, 3107. CrossRef
18. G. Y. Li, G. Zheng, and A. F. Noonan, J. Org. Chem., 2001, 66, 8677. CrossRef
19. S. R. Woodcock and B. P. Branchaud, Tetrahedron Lett., 2005, 46, 7213. CrossRef
20. (a) E. L. Eliel and S. H. Wilen, In Stereochemistry of Organic Compounds, 2nd ed.; Wiley-Interscience: New York, 1994, 618; (b) T. Imase, S. Kawauchi, and J. Watanabe, Macromol. Theory Simul., 2001, 10, 434. CrossRef
21. (a) J. Dupont, R. F. de Souza, and P. A. Z. Suarez, Chem. Rev., 2002, 102, 3667; CrossRef (b) R. J. Yogesh, Synlett, 2004, 746.
22. X. Xie, B. Chen, J. Lu, J. Han, X. She, and X. Pan, Tetrahedron Lett., 2004, 45, 6235. CrossRef
23. M. Lautens and Y-Q. Fang, Org. Lett., 2003, 5, 3679. CrossRef
24. K. Maeda, E. J. Farrington, E. Galardon, B. D. John, and J. M. Brown, Adv. Synth. Catal., 2002, 344, 104. CrossRef
25. M. Lautens, K. Fagnou, and M. Taylor, Org. Lett., 2000, 2, 1677. CrossRef
26. B. M. Trost, W. Tang, and F. D. Toste, J. Am. Chem. Soc., 2005, 127, 14785. CrossRef
27. T. Jeffery, J. Chem. Soc., Chem. Commun., 1984, 1287. CrossRef
28. J. E. M. Booker, A. Boto, G. H. Churchill, C. P. Green, M. Ling, G. Meek, J. Prabhakaran, D. Sinclair, A. J. Blake, and G. Pattenden, Org. Biomol. Chem., 2006, 4, 4193. CrossRef
29. F. N. Palmer, F. Lach, C. Poriel, A. G. Pepper, M. C. Bagley, A. M. Z. Slawin, and C. J. Moody, Org. Biomol. Chem., 2005, 3, 3805. CrossRef
30. A. Martins, U. Marquardt, N. Kasravi, D. Alberico, and M. Lautens, J. Org. Chem., 2006, 71, 4937. CrossRef
31. K. Parthasarathy, M. Jeganmohan, and C.-H. Cheng, Org. Lett., 2006, 8, 621. CrossRef
32. K. C. Majumdar, B. Chattopadhyay, and K. Ray, Tetrahedron Lett., 2007, 48, 7633. CrossRef
33. F. L. Strat, D. C. Harrowven, and J. Maddaluno, J. Org. Chem., 2005, 70, 489. CrossRef
34. K. C. Majumdar, A. K. Pal, A. Taher, and P. Debnath, Synthesis, 2007, 1707. CrossRef
35. B. Alcaide, P. Almendros, and R. Rodriguez-Acebes, J. Org. Chem., 2005, 70, 2713. CrossRef
36. X. Wang and J. A. Porco, Jr., J. Am. Chem. Soc., 2003, 125, 6040. CrossRef
37. G. A. Molander, and F. Dehmel, J. Am. Chem. Soc., 2004, 126, 10313. CrossRef
38. (a) G. C. Lloyd-Jones, Angew. Chem. Int. Ed., 2002, 41, 953; CrossRef (b) E. Piers and P. C. Marais, J. Org. Chem., 1990, 55, 3454; CrossRef (c) T. Wang and J. M. Cook, Org. Lett., 2000, 2, 2057; CrossRef (d) A. Chieffi, K. Kamikawa, J. Ahman, J. M. Fox, and S. L. Buchwald, Org. Lett., 2001, 3, 1897. CrossRef
39. (a) T. Pei, X. Wang, and R. A. Widenhoefer, J. Am. Chem. Soc., 2003, 125, 648; CrossRef (b) C. Liu, X. Wang, T. Pei, and R. A. Widenhoefer, Chem. Eur. J., 2004, 10, 634; CrossRef (c) X. Han, X. Wang, T. Pei, and R. A. Widenhoefer, Chem. Eur. J., 2004, 10, 6333; CrossRef (d) J.-H. Li, Q.-M. Zhu, Y. Liang, and D. Yang, J. Org. Chem., 2005, 70, 5347. CrossRef
40. K.-T. Yip, J.-H. Li, O.-Y. Lee, and D. Yang, Org. Lett., 2005, 7, 5717. CrossRef
41. D. Yang, J.-H. Li, Q. Gao, and Y.-L. Yan, Org. Lett., 2003, 5, 2869. CrossRef
42. (a) F. Kakiuchi and S. Murai, Acc. Chem. Res., 2002, 35, 826; CrossRef (b) F. Kakiuchi and N. Chatani, Adv. Synth. Catal., 2003, 345, 1077; CrossRef (c) L.-C. Campeau and K. Fagnou, Chem. Commun., 2005, 1253; (d) C. G. Espino and J. Du Bois, In Modern Rhodium-Catalyzed Organic Reactions; P. A. Evans, Ed.; Wiley-VCH: Weinheim, Germany, 2005, 379; (e) H. M. L. Davies, Angew. Chem. Int. Ed., 2006, 45, 6422; CrossRef (f) A. R. Dick and M. S. Sanford, Tetrahedron, 2006, 62, 2439; CrossRef (g) I. V. Seregin and V. Gevorgyan, Chem. Soc., Rev., 2007, 36, 1173; CrossRef (h) K. Godula and D. Sames, Science, 2006, 312, 67. CrossRef
43. (a) V. Ritleng, C. Sirlin, and M. Pfeffer, Chem. Rev., 2002, 102, 1731; CrossRef (b) C.-J. Li, Acc. Chem. Res., 2002, 35, 533; CrossRef (c) C. Jia, T. Kitamura, and Y. Fujiwara, Acc. Chem. Res., 2001, 34, 633; CrossRef (d) C. Jia, D. Piao, J. Oyamada, W. Lu, T. Kitamura, and Y. Fujiwara, Science, 2000, 287, 1992. CrossRef
44. (a) D. E. Ames and A. Opalko, Synthesis, 1983, 234; CrossRef (b) W. S. Yue and J. J. Li, Org. Lett. , 2002, 4, 2201; CrossRef (c) M. Lautens, J.-F. Paquin, and S. Piguel, J. Org. Chem., 2002, 67, 3972; CrossRef (d) S. Pache and M. Lautens, Org. Lett., 2003, 5, 4827; CrossRef (e) D. Alberico, J.-F. Paquin, and M. Lautens, Tetrahedron, 2005, 61, 6283; CrossRef (f) A. Martins, U. Marquardt, N. Kasravi, D. Alberico, and M. Lautens, J. Org. Chem., 2006, 71, 4937; CrossRef (g) D. Alberico and M. Lautens, Synlett, 2006, 2969; (h) T. A. Dwight, N. R. Rue, D. Charyk, R. Josselyn, and B. Deboef, Org. Lett., 2007, 9, 3137. CrossRef
45. (a) M. Catellani, In Handbook of Organopalladium Chemistry for Organic Synthesis; E. I. Negishi and A. de Meijere, Eds.; John Wiley & Sons: Hoboken, NJ, 2002, 1479; (b) M. Catellani, Synlett, 2003, 298; CrossRef (c) J. Tsuji, Palladium Reagents and Catalysis-New Perspectives for the 21st Century; John Wiley & Sons: New York, 2004, 409. CrossRef
46. (a) M. Lersch and M. Tislet, Chem. Rev., 2005, 105, 2471; CrossRef (b) A. E. Shilov and G. B. Shuípin, Chem. Rev., 1997, 97, 2879. CrossRef
47. (a) R. Giri, N. Maugel, J.-J. Li, D.-H. Wang, S. P. Breazzano, L. B. Saunders, and J.-Q. Yu, J. Am. Chem. Soc., 2007, 129, 3510; CrossRef (b) X. Chen, C. E. Goodhue, and J.- Q. Yu, J. Am. Chem. Soc., 2006, 128, 12634; CrossRef (c) V. G. Zaitsev, D. Shabashov, and O. Daugulis, J. Am. Chem. Soc., 2005, 127, 13154; CrossRef (d) D. Shaabashov and O. Daugulis, Org. Lett., 2005, 7, 3657; CrossRef (e) T. E. Barder, S. D. Walker, J. R. Martinelli, and S. L. Buchwald, J. Am. Chem. Soc., 2005, 127, 4685; CrossRef (f) H. Ren and P. Knochel, Angew. Chem. Int. Ed., 2006, 45, 3462; CrossRef (g) C.-G. Dong and Q.-G. Hu, Angew. Chem. Int. Ed., 2006, 45, 2289; CrossRef (h) S. J. Pastine, D. V. Gribkov, and D. Sames, J. Am. Chem. Soc., 2006, 128, 14220. CrossRef
48. (a) Z. Li and C.-J. Li, J. Am. Chem. Soc., 2004, 126, 11810; CrossRef (b) Z. Li and C.-J. Li, J. Am. Chem. Soc., 2005, 127, 6968; CrossRef (c) B. Deboef, S. J. Pastine, and D. Sames, J. Am. Chem. Soc., 2004, 126, 6556; CrossRef (d) H. Chen, L. Gan, Y. Shi, and X. Wei, J. Org. Chem., 2001, 66, 6369; CrossRef (e) Z. Li and C.-J. Li, J. Am. Chem. Soc., 2005, 127, 3672. CrossRef
49. M. Lafrance, S. I. Gorelsky, and K. Fagnou, J. Am. Chem. Soc., 2007, 129, 14570. CrossRef
50. M. Brookhart, M. L. H. Green, and G. Parkin, Proc. Natl. Acad. Sci., U.S.A. 2007, 104, 6908. CrossRef
51. (a) D. Alberico, M. E. Scott, and M. Lautens, Chem. Rev., 2007, 107, 174; CrossRef (b) G. Dyker, Angew. Chem, Int. Ed., 1999, 38, 1698; CrossRef (c) J. P. Wolfe and J. S. Thomas, Curr. Org. Chem., 2005, 9, 625. CrossRef
52. (a) F. Bellina, S. Cauteruccio, L. Mannina, R. Rossi, and S. Viel, J. Org. Chem., 2005, 70, 3997; CrossRef (b) J. G. Zeevaart, C. J. Parkinson, and C. B. de Koning, Tetrahedron Lett., 2004, 45, 4261; CrossRef (c) A. Yokooji, T. Okazawa, T. Satoh, M. Miura, and M. Nomura, Tetrahedron, 2003, 59, 5685; CrossRef (d) I. E. Nifant’ev, A. A. Sitnikov, N. V. Andriukhova, I. P. Laishevtsev, and Y. N. Luzikov, Tetrahedron Lett., 2002, 43, 3213; CrossRef (e) K. Li, Y. Zeng, B. Neuenswander, and J. A. Tunge, J. Org. Chem., 2005, 70, 6515; CrossRef (f) K. Inamoto, T. Saito, M. Katsuno, T. Sakamoto, and K. Hiroya, Org. Lett., 2007, 9, 2931. CrossRef
53. M. C. Harris, O. Geis, and S. L. Buchwald, J. Org. Chem., 1999, 64, 6019. CrossRef
54. L.-C. Campeau, M. Parisien, M. Leblanc, and K. Fagnou, J. Am. Chem. Soc., 2004, 126, 9186. CrossRef
55. J.-P. Leclere, M. André, and K. Fagnou, J. Org. Chem., 2006, 71, 1711. CrossRef
56. H. Abe, S. Takeda, T. Fujita, K. Nishioka, Y. Takeuchia, and T. Harayamaa, Tetrahedron Lett., 2004, 45, 2327. CrossRef
57. D. Alberico and M. Lautens, Synlett, 2006, 18, 2969.
58. D. Alberico, A. Rudolph, and M. Lautens, J. Org. Chem., 2007, 72, 775. CrossRef
59. M. Lautens, J. -F. Paquin, S. Piguel, and M. Dahlmann, J. Org. Chem., 2001, 66, 8127. CrossRef
60. F. Jafarpour and M. Lautens, Org. Lett., 2006, 8, 3601. CrossRef
61. B. Zhao and X. Lu, Org. Lett., 2006, 8, 5987. CrossRef
62. (a) H. Ohno, K. Miyamura, Y. Takeoka, and T. Tanaka, Angew. Chem. Int. Ed., 2003, 42, 2647; CrossRef (b) H. Ohno, M. Yamamoto, M. Iuchi, and T. Tanaka, Angew. Chem. Int. Ed., 2005, 44, 5103; CrossRef (c) H. Ohno, K. Miyamura, T. Mizutani, Y. Kadoh, Y. Takeoka, H. Hamaguchi, and T. Tanaka, Chem. Eur. J., 2005, 11, 3728. CrossRef
63. H. Ohno, M. Iuchi, N. Fujii, and T. Tanaka, Org. Lett., 2007, 9, 4813. CrossRef
64. (a) C. Bour and J. Suffert, Org. Lett., 2005, 7, 653; CrossRef (b) J. Zhao, M. A. Campo, and R. C. Larock, Angew. Chem. Int. Ed., 2005, 44, 1873; CrossRef (c) H.-J. Knölker, R. R. Kethiri, Chem. Rev., 2002, 102, 4303; CrossRef (d) E. Duval and G. D. Cuny, Tetrahedron Lett., 2004, 45, 5411. CrossRef
65. J. Zhao and R. C. Larock, J. Org. Chem., 2006, 71, 5340. CrossRef
66. Q. Huang, A. Fazio, G. Dai, M. A. Campo, and R. C. Larock, J. Am. Chem. Soc., 2004, 126, 7460. CrossRef
67. (a) H.-W. Frühauf, Chem. Rev., 1997, 97, 523; CrossRef (b) B. Schmidt, Angew. Chem. Int. Ed., 2003, 42, 4996. CrossRef
68. (a) B. M. Trost, F. D. Tosate, and A. B. Pinkerton, Chem. Rev., 2001, 101, 2067; CrossRef (b) C. Aubert, O. Buisine, and M. Malacria, Chem. Rev., 2002, 102, 813. CrossRef
69. R. A Widenhoefer, Acc. Chem. Res., 2002, 35, 905. CrossRef
70. (a) B. M. Trost and F. D. Toste, J. Am. Chem. Soc., 1996, 118, 6305; CrossRef (b) A. Goeke, M. Sawamura, R. Kuwano, and Y. Ito, Angew. Chem., Int. Ed. Engl., 1996, 35, 662. CrossRef
71. (a) B. M. Trost and F. D. Toste, J. Am. Chem. Soc., 2000, 122, 714; CrossRef (b) P. Cao, B. Wang, and X. Zhang, J. Am. Chem. Soc., 2000, 122, 6490; CrossRef (c) Y. Yamamoto, Y. Nakagai, N. Ohkoshi, and K. Itoh, J. Am. Chem. Soc., 2001, 123, 6372; CrossRef (d) K. M. Brummond, H. Chen, P. Sill, and L. You, J. Am. Chem. Soc., 2002, 124, 15186. CrossRef
72. A. Hercouet, F. Berrée, C. H. Lin, L. Toupet, and B. Carboni, Org. Lett., 2007, 9, 1717. CrossRef
73. (a) G. Zhu, X. Tong, J. Cheng, Y. Sun, D. Li, and Z. Zhang, J. Org. Chem., 2005, 70, 1712; CrossRef (b) G. Zhu, and Z. Zhang, Org. Lett., 2003, 5, 3645. CrossRef
74. S. Ma, L. Lu, and J. Zhang, J. Am. Chem. Soc., 2004, 126, 9645. CrossRef
75. S. Brase, H. Wertal, D. Franf, D. Vidovic, and A. de Meijere, Eur. J. Org. Chem., 2005, 70, 4167. CrossRef
76. (a) B. M. Trost, Acc. Chem. Res., 1990, 23, 34; CrossRef (b) M. Toyota, T. Wada, M. Matsuura, and K. Fukumoto, Synlett, 1995, 761. CrossRef
77. C.-Y. Lo, C.-C. Lin, H. –M. Cheng, and R.-S. Liu, Org. Lett., 2006, 8, 3153. CrossRef
78. (a) X. Lu and Q. Zhang, Pure Appl. Chem., 2001, 73, 247; CrossRef (b) H. Zhao, A. Ariafard, and Z. Lin, Organometallics, 2006, 25, 812. CrossRef
79. (a) X. Tong, Z. Zhang, and X. Zhang, J. Am. Chem. Soc., 2003, 125, 6370; CrossRef (b) X. Tong, D. Li, Z. Zhang, and X. Zhang, J. Am. Chem. Soc., 2004, 126, 7601. CrossRef
80. (a) L. Zhao, X. Lu, and W. Xu, J. Org. Chem., 2005, 70, 4059; CrossRef (b) W. Xu, A. Kong, and X. Lu, J. Org. Chem., 2006, 71, 3854. CrossRef
81. G. Zhu and Z. Zhang, J. Org. Chem., 2005, 70, 3339. CrossRef
82. (a) G. W. Coates, Chem. Rev., 2000, 100, 1223; CrossRef (b) K. Mikami, M. Hatano, and K. Akiyama, Top. Organomet. Chem., 2005, 14, 279.
83. J. Song, Q. Shen, F. Xu, and X. Lu, Org. Lett., 2007, 9, 2947. CrossRef
84. P. J. Stang, D. H. Cao, G. T. Poulter, and A. M. Arif, Organometallics, 1995, 14, 1110. CrossRef
85. D. J. Ramón and M. Yus, Angew. Chem. Int. Ed., 2004, 43, 284. CrossRef
86. C.–M. Yu, J. Youn, and M.–K. Lee, Org. Lett., 2005, 7, 3733. CrossRef
87. (a) S. Yamago and E. Nakamura, Org. React., 2002, 61, 1; (b) A. Brandi, S. Cachi, F. M. Cordero, and A. Goti, Chem. Rev., 2003, 103, 1213; CrossRef (c) A. Delgado, J. R. Rodríguez, L. Castedo, and J. L. Mascarenãs, J. Am. Chem. Soc., 2003, 125, 9282. CrossRef
88. B. M. Trost, R. C. Bunt, R. C. Lemoine, and T. L. Calins, J. Am. Chem. Soc., 2000, 122, 5968. CrossRef
89. C. Larksarp and H. Alper, J. Org. Chem., 2001, 66, 3502. CrossRef
90. G. E. Greco, B. L. Gleason, T. A. Lowery, M. J. Kier, L. B. Hollander, S. A.Gibbs, and A. D. Worthy, Org. Lett., 2007, 9, 3817. CrossRef
91. Y. Zhang and M. Sigman, Org. Lett., 2006, 8, 5557. CrossRef
92. M. Gulías, J. Durán, F. López, L. Castedo, and J. L. Mascarenãs, J. Am. Chem. Soc., 2007, 129, 11026. CrossRef
93. (a) I. U. Khand, G. R. Knox, P. L. Pauson, W. E. Watts, and M. I. Foreman, J. Chem. Soc., Perkin Trans. 1 1973, 977; CrossRef (b) O. Geis, and H. G. Schmalz, Angew. Chem. Int. Ed., 1998, 37, 911; CrossRef (c) L. V. R. Bonaga and M. E. Krafft, Tetrahedron, 2004, 60, 9795. CrossRef
94. R. Grigg, L. Zhang, S. Collard, and A. Keep, Chem. Commun., 2003,1902.
95. Y. Tang, L. Deng, Y. Zhang, G. Dong, J. Chen, and Z. Yang, Org. Lett., 2005, 7, 1657. CrossRef
96. Y. Tang, L. Deng, Y. Zhang, G. Dong, J. Chen, and Z. Yang, Org. Lett., 2005, 7, 593. CrossRef
97. (a) S. R. Fix, J. L. Brice, and S. S. Stahl, Angew. Chem. Int. Ed., 2002, 41, 164; CrossRef (b) R. W. Bates and K. Sa-Ei, Org. Lett., 2002, 4, 4225; CrossRef (c) H. Sasai, Tetrahedron Lett., 2003, 44, 711. CrossRef
98. R. M. Trend, Y. K. Ramtohul, and B. M. Stoltz, J. Am. Chem. Soc., 2005, 127, 17778. CrossRef
99. J. Muzart, Tetrahedron, 2005, 61, 5955. CrossRef
100. V. N. Korotchenko and M. R. Gagne, J. Org. Chem., 2007, 72, 4877. CrossRef
101. R. C. B. Verboom, A. Persson, and J.-E. Bäckvall, J. Org. Chem., 2004, 69, 3102. CrossRef
102. T. Hayashi, K. Yamasaki, M. Mimura, and Y. Uozumi, J. Am. Chem. Soc., 2004, 126, 3036. CrossRef
103. (a) Y. Imai, W. Zhang, T. Kida, Y. Nakatsuji, and I. Ikeda, J. Org. Chem., 2000, 65, 3326; CrossRef (b) W. Zhang, F. Xie, S. Matsuo, M. Imahori, T. Kida, Y. Nakatsuji, and I. Ikeda, Tetrahedron: Asymmetry, 2006, 17, 767. CrossRef
104. F. Wang, Y. J. Zhang, G. Yang, and W. Zhang, Tetrahedron Lett., 2007, 48, 4179. CrossRef
105. S. Yamaguchi, S. Muro, M. Kobayashi, M. Miyazawa, and Y. Hirai, J. Org. Chem., 2003, 68, 6274. CrossRef
106. B. M. Trost, H. C. Shen, L. Dong, J.-P. Surivet, and C. Sylvain, J. Am. Chem. Soc., 2004, 126, 11966. CrossRef
107. S. C. Pelly, S. Govender, M. A. Fernandes, H.-G. Schmalz, and C. B. de Koning, J. Org. Chem., 2007, 72, 2857. CrossRef
108. (a) J. Tsuji, Transition Metal Reagents and Catalysts; John Wiley & Sons Ltd.: New York, 2000, 305; (b) F. Z. Dörwald, Chem. Rev., 2004, 104, 2191.
109. B. M. Trost, M. R. Machacek, B. D. Faulk, J. Am. Chem. Soc., 2006, 128, 6745. CrossRef
110. (a) B. M. Trost and D. E. Patterson, J. Org. Chem., 1998, 63, 1339; CrossRef (b) B. M. Trost and D. E. Patterson, Chem. Eur. J., 1999, 5, 3279. CrossRef
111. (a) B. M. Trost and F. D. Toste, J. Am. Chem. Soc., 1999, 121, 4525; (b) B. M. Trost, Acc. Chem. Res., 1996, 29, 355. CrossRef
112. R. A. Widenhoefer and S. L. Buchwald, J. Am. Chem. Soc., 1998, 120, 6504. CrossRef
113. (a) H. E. Bryndza, Organometallics, 1985, 4, 406; CrossRef (b) H. E. Bryndza, J. C. Calabrese, and S. S. Wreford, Organometallics, 1984, 3, 1603. CrossRef
114. M. B. Hay, A. R. Hardin, and J. P. Wolfe, J. Org. Chem., 2005, 70, 3099. CrossRef
115. M. Oestreich, F. Sempere-Culler, and A. B. Machotta, Angew. Chem. Int. Ed., 2005, 44, 149. CrossRef
116. J. P. Wolfe, Eur. J. Org. Chem., 2007, 571. CrossRef
117. J. S. Nakhla, J. W. Kampf, and J. P. Wolfe, J. Am. Chem. Soc., 2006, 128, 2893. CrossRef
118. B. Hulin, L. S. Newton, S. Cabral, A. J. Walker, and J. Bordner, Org. Lett., 2004, 6, 4343. CrossRef
119. B. M. Trost and J. Quancard, J. Am. Chem. Soc., 2006, 128, 6314. CrossRef
120. Y. Tamaru, Eur. J. Org. Chem., 2005, 2647. CrossRef
121. M. Miyazawa, Y. Hirose, M. Narantsetseg, H. Yokoyama, S. Yamaguchi, and Y. Hirai, Tetrahedron Lett., 2004, 45, 2883. CrossRef
122. (a) H. F. Schuster and G. M. Coppola, Allenes in Organic Synthesis; John Wiley & Sons: New York, 1984; (b) S. Ma, Chem. Rev., 2005, 105, 2829 and references therein; CrossRef (c) J. D. Winkler and J. R. Ragains, Org. Lett., 2006, 8, 4031; CrossRef (d) B. M. Trost and J. Xie, J. Am. Chem. Soc., 2006, 128, 6044; CrossRef (e) T. Yoshino, F. Ng, and S. J. Danishefsky, J. Am. Chem. Soc., 2006, 128, 14185. CrossRef
123. (a) G. Liu and X. Lu, Org. Lett., 2001, 3, 3879; CrossRef (b) N. Krause, A. Hoffmann-Roder, and J. Canisius, Synthesis, 2002, 1759; CrossRef (c) N. A. Nedolya, N. I. Schlyakhtina, V. P. Zinov’eva, A. I. Albanov, and L. Brandsma, Tetrahedron Lett., 2002, 43, 1569. CrossRef
124. A. S. K. Hashmi, T. L. Ruppert, T. Knöfel, and J. W. Bats, J. Org. Chem., 1997, 62, 7295. CrossRef
125. (a) S. Ma and Z. Yu, Org. Lett., 2003, 5, 2581; CrossRef (b) S. Ma, Z. Yu, and Z. Gu, Chem. Eur. J., 2005, 11, 2351. CrossRef
126. (a) S. Ma and Z. Yu, Angew. Chem., Int. Ed., 2002, 41, 1775; CrossRef (b) S. Ma, Z. Gu, and Z. Yu, J. Org. Chem., 2005, 70, 6291. CrossRef
127. S. Ma and Z. Gu, J. Am. Chem. Soc., 2005, 127, 6182. CrossRef
128. B. Alcaide, P. Almendros, and T. M. Campo, Angew. Chem., Int. Ed., 2006, 45, 4501. CrossRef
129. Z. Gu, X. Wang, W. Shu, and S. Ma, J. Am. Chem. Soc., 2007, 129, 10948. CrossRef
130. C. Shin, Y. Oh, J. H. Cha, A. N. Pae, H. Choo, and Y. S. Cho, Tetrahedron, 2007, 63, 2182. CrossRef
131. Y. Liag, T. X.-D. Zhang, L.-Q. Mao, Y.-X. Xie, and J.-H. Li, Org. Lett., 2006, 8, 3017. CrossRef
132. (a) G. Zhu and X. Lu, J. Organomet. Chem., 1996, 508, 83; CrossRef (b) A. Jeevanandam, K. Nakamura, and Y.–C. Liang, J. Org. Chem., 2001, 66, 514. CrossRef
133. B. Gabriele, G. Salerno, A. Fazio, and R. Pittelli, Tetrahedron, 2003, 59, 6251. CrossRef
134. (a) J. E. Baldwin, J. Chem. Soc., Chem. Commun., 1976, 734; CrossRef (b) J. E. Baldwin, J. Cutting, W. Dupont, L.Kruse, L. Silberman, and R. C. Thomas, J. Chem. Soc., Chem. Commun., 1976, 736. CrossRef
135. (a) K. Hiroya, R. Jouka, M. Kameda, A. Yasuhara, and T. Sakamoto, Tetrahedron, 2001, 57, 9697; CrossRef (b) A. Padwa, K. E. Krumpe, and M. D. Weingarten, J. Org. Chem., 1995, 60, 5595. CrossRef
136. C. V. Ramana, R. Mallik, R. G. Gonnade, and M. K.Gurjar, Tetrahedron Lett., 2006, 47, 3649. CrossRef
137. J. Zhang, X. Zhao, and L. Lu, Tetrahedron Lett., 2007, 48, 1911. CrossRef
138. M. Yoshida, Y. Morishita, M. Fujita, and M. Ihara, Tetrahedron Lett., 2004, 45, 1861. CrossRef
139. R. Bernini, S. Cacchi, I. De Salve, and G. Fabrizi, Synthesis, 2007, 873.
140. F. Silva, M. Reiter, R. Mills-Webb, M. Sawicki, D. Klar, N. Bensel, A. Wagner, and V. Gouverneur, J. Org. Chem., 2006, 71, 8390. CrossRef
141. B. Gabriele, G. Salerno, U. Veltri, R. Mancuso, Z. Li, A. Crispini, and A. Bellusci, J. Org. Chem., 2006, 71, 7895. CrossRef
142. B. Gabriele, G. Salerno, and P. Plastina, Lett. Org. Chem., 2004, 1, 134. CrossRef
143. P. Plastina, B. Gabriele, and G. Salerno, Synthesis, 2007, 3083. CrossRef
144. B. Gabriele, P. Plastina, G. Salerno, and R. Mancuso, Synthesis, 2006, 4247. CrossRef
145. B. Gabriele, R. Mancuso, G. Salerno, and L. Veltri, Chem. Commun., 2005, 271. CrossRef
146. B. Gabriele, R. Mancuso, G. Salerno, and M. Costa, Adv. Synth. Catal., 2006, 348, 1101. CrossRef
147. B. Gabriele, R. Mancuso, G. Salerno, and M. Costa, J. Org. Chem., 2007, 72, 9278. CrossRef
148. A. Bacchi, M. Costa, N. D. Ca, M. Fabricatore, A. Fazio, B. Gabriele, C. Nasi, and G. Salerno, Eur. J. Org. Chem., 2004, 574. CrossRef
149. K. Kato, H. Nouchi, K. Ishikura, S. Takaishi, S. Motodate, H. Tanaka, K. Okudaira, T. Mochida, R. Nishigaki, K. Shigenoubu, and H. Akita, Tetrahedron, 2006, 62, 2545. CrossRef
150. L.-L. Wei, L.-M. Wei, W.-B. Pan, and M.-J. Wu, Synlett, 2004, 1497.
151. S. Mondal, T. Nogami, N. Asao, and Y. Yamamoto, J. Org. Chem., 2003, 68, 9496. CrossRef
152. M. Costa, N. D. Ca, B. Gabriele, C. Massera, G. Salerno, and M. Soliani, J. Org. Chem., 2004, 69, 2469. CrossRef
153. A. Bacchi, M. Costa, N. D. Ca, B. Gabriele, G. Salerno, and S. Cassoni, J. Org. Chem., 2005, 70, 4971. CrossRef
154. K. Zong, K. A. Abboud, and J. R. Reynolds, Tetrahedron Lett., 2004, 45, 4973. CrossRef
155. M. Palucki and N. Yasuda, Tetrahedron Lett., 2005, 46, 987. CrossRef
156. K. Kawaguchi, K. Nakano, and K. Nozaki, J. Org. Chem., 2007, 72, 5119. CrossRef
157. (a) Top. Organomet. Chem. 2006, Vol. 18, Ed. by M. Beller; (b) S. A. Vizer, K. B. Yerzhanov, A. A. A. A. Quntar, and V. M. Dembitsky, Tetrahedron, 2004, 60, 5499; CrossRef (c) J. Tsuji, Palladium Reagents and Catalysts: Innovation in Organic Synthesis; John Wiley & Sons: Chichester, U.K., 1995.
158. (a) B. Gabriele, G. Salerno, M. Costa, and G. P. Chiusoli, Curr. Org. Chem., 2004, 8, 919; CrossRef (b) G. Vasapollo, and G. Mele, Curr. Org. Chem., 2006, 10, 1397; CrossRef (c) D. H. Gibson, Chem. Rev., 1996, 96, 2063. CrossRef
159. S. Ma, B. Wu, and S. Zhao, Org. Lett., 2003, 5, 4429. CrossRef
160. W.-J. Xiao and H. Alper, J. Org. Chem., 2005, 70, 1082.
161. D. V. Kadnikov and R. C. Larock, J. Org. Chem., 2003, 68, 9423. CrossRef
162. (a) B. Gabriele, G. Salerno, P. Plastina, M. Costa, and A. Crispini, Adv. Synth. Catal., 2004, 346, 351; CrossRef (b) B. Gabriele, P. Plastina, G. Salerno, and M. Costa, Synlett, 2005, 935. CrossRef
163. N.-W. Jan and H.-J. Liu, Org. Lett., 2006, 8, 151. CrossRef
164. H. Cao, W.-J. Xiao, and H. Alper, Adv. Synth. Catal., 2006, 348, 1807. CrossRef
165. H. Cao, W.-J. Xiao, and H. Alper, J. Org. Chem., 2007, 72, 8562. CrossRef
166. M. Yoshida, M. Fujita, and M. Ihara, Org. Lett., 2003, 5, 3325. CrossRef
167. (a) G. Battistuzzi, S. Cacchi, and G. Fabrizi, Eur. J. Org. Chem., 2002, 58, 2671; CrossRef (b) M. C. Willis, D. Taylor, and A. T. Gillmore, Org. Lett., 2004, 6, 4755; CrossRef (c) R. C. Larock, In Palladium-Catalyzed Annulation of Alkynes; J. Tsuji, Ed.; Topics in Organometallic Chemistry; Spring-Verlag: Berlin, Heidelberg, 2005, 14, 147.
168. (a) K. Sonogashira, In Comprehensive Organic Synthesis; B. M. Trost, and I. Fleming, Eds.; Pergamon: New York,1991, 3, 521; (b) S. Thorand and N. Krause, J. Org. Chem.,1998, 63, 8551. CrossRef
169. (a) T. Konno, J. Chae, T. Ishihara, and H. Yamanaka, Tetrahedron, 2004, 60, 11695; CrossRef (b) M. Shen, G. Li, B. Z. Lu, A. Hossain, F. Roschangar, V. Farina, C. H. Senanayake, Org. Lett., 2004, 6, 4129; CrossRef (c) N. Gathergood and P. J. Scammells, Org. Lett., 2003, 5, 921; CrossRef (d) S. Cacchi, G. Fabrizi, D. Lamba, F. Marinelli, and L. M. Parisi, Synthesis, 2003, 728; CrossRef (e) G. Dai and R. C. Larock, J. Org. Chem., 2003, 68, 920. CrossRef
170. S. Raju, V. R. Batchu, N. K. Swamy, R. V. Dev, J. M. Babu, P. R. Kumar, K. Mukkantic, and M. Pal, Tetrahedron Lett., 2006, 47, 83. CrossRef
171. M. Pal, V. Subramanian, and K. R. Yeleswarapu, Tetrahedron Lett., 2003, 44, 8221. CrossRef
172. A. Duchêne, J. Thibonnet, J.-L. Parrain, E. Anselmi, and M. Abarbri, Synthesis, 2007, 597.
173. Y. Wang and D. J. Burton, J. Org. Chem., 2006, 71, 3859. CrossRef
174. A. Cwik, Z. Hell, and F. Figueras, Tetrahedron Lett., 2006, 47, 3023. CrossRef
175. S. Venkataraman, D. K. Barange, and M. Pal, Tetrahedron Lett., 2006, 47, 7317. CrossRef
176. (a) Z. Janeba, J. Balzarini, G. Andrei, R. Snoeck, E. De Clercq, and M. J. Robins, J. Med. Chem., 2005, 48, 4690; CrossRef (b) N. T. Patil, H. Wu, and Y. Yamamoto, J. Org. Chem., 2005, 70, 4531. CrossRef
177. Z. Liang, S. Ma, J. Yu, and R. Xu, J. Org. Chem., 2007, 72, 9219. CrossRef
178. (a) K. Fugami, K. Oshima, and K. Utimoto, Bull. Chem. Soc. Jpn., 1989, 62, 2050; CrossRef (b) R. C. Larock and N. H. Lee, J. Am. Chem. Soc., 1991, 113, 7815; CrossRef (c) G. A. Kraus and J. Thurston, J. Am. Chem. Soc., 1989, 111, 9203; CrossRef (d) S. Trudeau and J. P. Morken, Org. Lett., 2005, 7, 5465; CrossRef (e) J.-H. Sohn, N. Waizumi, H. M. Zhong, and V. H. Rawal, J. Am. Chem. Soc., 2005, 127, 7290. CrossRef
179. K. Minami, Y. Kawamura, K. Koga, and T. Hosokawa, Org. Lett., 2005, 7, 5689. CrossRef
180. M. A. Evans and J. P. Morken, Org. Lett., 2005, 7, 3367. CrossRef
181. M. A. Evans and J. P. Morken, Org. Lett., 2005, 7, 3371. CrossRef
182. J. Boukouvalas, M. Pouliot, J. Robichaud, S. MacNeil, and V. Snieckus, Org. Lett., 2006, 8, 3597. CrossRef
183. K.Tamaso, Y. Hatamoto, S. Sakaguchi, Y. Obora, and Y. Ishii, J. Org. Chem., 2007, 72, 3603. CrossRef
184. K.-I. Tamaso, Y. Hatamoto, Y. Obora, S. Sakaguchi, and Y. Ishii, J. Org. Chem., 2007, 72, 8820. CrossRef
185. (a) T. Yokota, M. Tani, S. Sakaguchi, and Y. Ishii, J. Am. Chem. Soc., 2003, 125, 1476; CrossRef (b) M. Tani, S. Sakaguchi, and Y. Ishii, J. Org. Chem., 2004, 69, 1221. CrossRef
186. R. V. Rozhkov and R. C. Larock, Tetrahedron Lett., 2004, 45, 911. CrossRef
187. R. V. Rozhkov and R. C. Larock, J. Org. Chem., 2003, 68, 6314. CrossRef
188. (a) C. Hulme and V. Gore, Curr. Med. Chem., 2003, 10, 51; CrossRef (b) R. V. A. Orru and M. de Greef, Synthesis, 2003, 1471; CrossRef (c) J. Zhu, Eur. J. Org. Chem., 2003, 1133. CrossRef
189. (a) L. Weber, K. Illgen, and M. Almstetter, Synlett, 1999, 366; CrossRef (b) D. J. Ramon and M. Yus, Angew. Chem. Int. Ed., 2005, 44, 1602. CrossRef
190. G. Balme, E. Bossharth, and N. Monteiro, Eur. J. Org. Chem., 2003, 4101. CrossRef
191. Y. Li, Z. Yu, and H. Alper, Org. Lett., 2007, 9, 1647. CrossRef
192. M. von Seebach, R. Grigg, and A. de Meijere, Eur. J. Org. Chem., 2002, 3268. CrossRef
193. E. Motti, F. Faccini, I. Ferrari, M. Catellani, and R. Ferraccioli, Org. Lett., 2006, 8, 3967. CrossRef
194. M. Szlosek-Pinaud, P. Diaz, J. Martineza, and F. Lamaty, Tetrahedron Lett., 2003, 44, 8657. CrossRef
195. X. Geng, M. L. Miller, S. Lin, and I. Ojima, Org. Lett., 2003, 5, 3733. CrossRef
196. H. T. Brink, D. T. S. Rijkers, and R. M. J. Liskamp, J. Org. Chem., 2006, 71, 1817. CrossRef
197. M. Oikawa, Y. Takeda, S. Naito, D. Hashizume, H. Koshinob, and M. Sasaki, Tetrahedron Lett., 2007, 48, 4255. CrossRef
198. J. Peng, W. Lin, S. Yuan, and Y. Chen, J. Org. Chem., 2007, 72, 3145. CrossRef
199. K. G. Dongol and B. Y. Tay, Tetrahedron Lett., 2006, 47, 927. CrossRef
200. P. von Matt and A. Pfaltz, Angew. Chem., Int. Ed. Engl., 1993, 32, 566. CrossRef
201. K. Ito, Y. Imahayashi, T. Kuroda, S. Eno, B. Saito, and T. Katsuki, Tetrahedron Lett., 2004, 45, 7277. CrossRef
202. S.-C. Yang, H.-C. Lai, and Y.-C. Tsai, Tetrahedron Lett., 2004, 45, 2693. CrossRef
203. S.-C. Yang, P.-C. Liu, and W.-H Feng,. Tetrahedron Lett., 2004, 45, 4951. CrossRef
204. S. Tanimori, Y. Kato, and M. Kirihata, Synthesis, 2004, 2103. CrossRef
205. (a) A. Neogi, T. P. Majhi, R. Mukhopadhyay, and P. Chattopadhyay, J. Org. Chem., 2006, 71, 3291; CrossRef (b) A. Neogi, T. P. Majhi, B. Achari, and P. Chattopadhyay, Eur. J. Org. Chem., 2008, 330. CrossRef
206. R. Omar-Amrani, R. Schneider, and Y. Fort, Synthesis, 2004, 2527.
207. C. Ma, S.-J. Liu, L. Xin, J. R. Falck, and D.-S. Shin, Tetrahedron, 2006, 62, 9002. CrossRef
208. B. J. Margolis, J. J. Swidorski, and B. N. Rogers, J. Org. Chem., 2003, 68, 644. CrossRef
209. B. Gabriele, R. Mancuso, G. Salerno, and M. Costa, J. Org. Chem., 2003, 68, 601. CrossRef
210. B. Gabriele, G. Salerno, D. Brindisi, M. Costa, and G. P. Chiusoli, Org. Lett., 2000, 2, 625. CrossRef
211. J. Liu, M. Shen, Y. Zhang, G. Li, A. Khodabocus, S. Rodriguez, B. Qu, V. Farina, C. H. Senanayake, and B. Z. Lu, Org. Lett., 2006, 8, 3573. CrossRef
212. B. Gabriele, P. Plastina, G. Salerno, R. Mancuso, and M. Costa, Org. Lett., 2007, 9, 3319. CrossRef
213. K. C. Majumdar, S. Alam, and B. Chattopadhyay, Tetrahedron, 2008, 64, 597. CrossRef
214. S. W. Youn and J. I. Eom, Org. Lett., 2005, 7, 3355. CrossRef
215. U. K. Tambar, T. Kano, and B. M. Stoltz, Org. Lett., 2005, 7, 2413. CrossRef