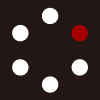
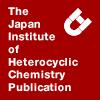
HETEROCYCLES
An International Journal for Reviews and Communications in Heterocyclic ChemistryWeb Edition ISSN: 1881-0942
Published online by The Japan Institute of Heterocyclic Chemistry
e-Journal
Full Text HTML
Received, 16th July, 2010, Accepted, 2nd September, 2010, Published online, 3rd September, 2010.
DOI: 10.3987/REV-10-SR(E)7
■ Further Application of the Multi-Template Approach for Creation of Biological Response Modifiers: Discovery of a New Class of Multifunctional Anti-Diabetic Agents
Kazunori Motoshima, Tomomi Noguchi-Yachide, Minoru Ishikawa, Yuichi Hashimoto, and Kazuyuki Sugita*
Institute of Molecular and Cellular Biosciences, The University of Tokyo, 1-1-1 Yayoi, Bunkyo-ku, Tokyo 113-0032, Japan
Abstract
We have previously employed the multi-template approach to create many types of biologically active compounds. In this review, we focus on extension of the multi-template approach to develop candidate anti-diabetic and anti-metabolic syndrome agents with a well-balanced range of activities.INTRODUCTION
Thalidomide (1), which was used as sedative in the middle of the 20th century, but was withdrawn after it proved to be teratogenic, nevertheless shows versatile biological activities,1-6 and indeed, has been brought back into clinical use for the treatment of multiple myeloma and Hansen’s disease. We hypothesized that thalidomide possesses a versatile structure with affinity for a wide variety of binding sites of endogenous signal messenger proteins. Based on this idea, we have developed many structurally new biological response modifiers (BRMs), including liver X receptor (LXRα and LXRβ) antagonists,7-9 α-glucosidase inhibitors7,10-12 and glycogen phosphorylase inhibitors.13 The foundation of the multi-template approach is that the number of three-dimensional spatial structures (fold structures) of human proteins is only approximately 1000, which is much smaller than the number of human proteins, estimated to be 50,000-70,000.14-16 Therefore, ignoring physical/chemical interactions, one might expect that a template structure that is spatially adapted to one fold structure might serve as a multi-template that would interact specifically with 50-70 different proteins. We have focused on thalidomide as one such multi-template.
Diabetes mellitus (type 2) and the metabolic syndrome are associated with a clustering of many risk factors, which correlate to increased cardiovascular risk. Treatment guidelines highlight the need for stricter management of dyslipidemia to reduce cardiovascular risk in people with type 2 diabetes. Despite optimal therapy for pathology,17 many patients fail to achieve their therapeutic goal, and more effective drugs are required. We have been working on the creation of functional BRMs by means of the multi-template approach. In previous studies,7,13 we chose as a lead structure PP2P (2), which possesses a phthalimide moiety adopted from thalidomide structure and a diphenyl ethane or ethene unit from riccardin C (3). In this review, we describe this new extension of the multi-template approach into the area of anti-diabetic and anti-metabolic syndrome agents, focusing on modulation of PPARs, which are recognized as among the most important drug targets for the treatment of cardiovascular diseases, as well as LXR antagonist,7 α-glucosidase-inhibitory7 and glycogen phosphorylase-inhibitory13 activities. We believe that this multi-template approach represents a novel drug discovery methodology for creation of multi-target BRMs with great therapeutic potential.
1. Liver X receptor antagonists7
The human nuclear receptor superfamily consists of 48 members.18 The nuclear receptors bind to endogenous ligands and elicit conformational changes, recruitment of cofactors, and finally gene expression. Among them, LXRs (LXRα and LXRβ) are believed to act as cholesterol sensors regulating lipid homeostasis.19 Moreover, LXRs regulate the expression of genes involved in hepatic gluconeogenesis (e.g., Pepck) and glucose transport (e.g., Glut4).20-26 All these observations suggest that LXRs may be an effective target for the treatment of metabolic syndrome, including diabetes.
Oxysterols, 24(S),25-epoxycholesterol and 22(R)-hydroxycholesterol, are endogenous ligands of LXRs,27 while T0901317 (4)28 and GW3965 (5)29 are potent LXR pan-agonists which are used experimentally as biological tools. These nonselective LXR agonists, however, cause undesired activation of triglyceride (TG) synthesis and this activity prohibits their clinical application. LXR agonists increase the level of sterol regulatory binding protein 1c (SREBP1c)30,31 and carbohydrate response element-binding protein (ChREBP),32 followed by activation of hepatic lipogenesis, such as fatty acid synthesis. LXR-mediated lipogenesis is attributed to a massive increase of SREBP1c expression. However, knockout of the srebp1c gene in rodents caused only a 50% reduction of hepatic fatty acid synthesis. Furthermore, when srebp1c-knockout mice were treated with the LXR agonist T0901317 (4), up-regulation of lipogenic genes and elevation of fatty acid synthesis were still observed. ChREBP promotes lipogenesis by acting as a glucose-sensitive transcription factor and increasing the hepatic conversion of excess carbohydrate to lipid. LXR agonists are expected to be potent cardiovascular agents, if their strong lipogenic activity mediated by SREBP1c and ChREBP could be nullified. Dentin et al. reported that liver-specific inhibition of ChREBP in ob/ob mice improved hepatic steatosis and insulin resistance.33 LXRα is mostly expressed in liver, whereas LXRβ has a more widespread pattern of expression, being found in nearly every tissue.34 Thus, LXRα-selective antagonists might be effective inhibitors of hepatic lipogenesis, if a decrease of HDL levels can be avoided. We have created various LXR antagonists, including PP2P (2),8,9 and therefore, we aimed at generating LXRα-selective antagonists by the structural development of PP2P (2).
LXR-antagonistic activity of the synthesized compounds is summarized in Table 1 (phenethylphenylphthalimide derivatives) and Table 2 (4,5,6,7-tetrachlorophenethylphenylphthalimide derivatives).
PP2P (2) possesses weak LXRα-selective antagonistic activity. Compound (25) which bears a 4”-methoxyphenethyl unit in the 4’-position exhibited LXRα-selective antagonistic activity with weak LXRβ-antagonistic activity (LXRα : IC50 = 0.2 μM, LXRβ : IC50 >30 μM). There is no difference in amino acid sequences in the ligand-binding site region between LXRα and β,35,36 and we consider that the selectivity arises from the interaction between helix-3 of LXR, in which one amino acid difference exists (LXRβ Ile277/LXRα Val263)37 and the 4”-methoxy group in the 4’-phenethyl moiety.7 On the other hand, compounds (18) and (23), possessing a 3”-methoxy group in the 4’-phenethyl moiety, exhibited LXR-antagonistic activity predominantly over α-glucosidase-inhibitory activity.
2. α-Glucosidase inhibitors7
α-Glucosidase belongs to family 13 of glycoside hydrolase (GH13) and hydrolyzes 1,4-linked α-D-glucose residues with release of free α-D-glucose.38 Its inhibitors can retard the uptake of dietary carbohydrates and suppress postprandial hyperglycemia; therefore, effective α-glucosidase inhibitors are candidate drugs to treat diabetic and/or obese patients.39-41 Voglibose is a representative α-glucosidase inhibitor which is commercially available.42 α-Glucosidase inhibitors show a powerful hypoglycemic effect in clinical use. 1-Deoxynojirimycin (dNM),43 a classical α-glucosidase inhibitor often used as a standard, showed weak α-glucosidase-inhibitory activity with an IC50 value of 250 μM under our experimental conditions.
During our studies, we found that several thalidomide-related phthalimide derivatives, including PP2P (2) (Fig. 1), possess both LXR-antagonistic activity and α-glucosidase-inhibitory activity. The co-existence of LXR-modulating activity and α-glucosidase-inhibitory activity may be a rather general phenomenon, because typical LXR ligands, including T0901317 (4), GW3965 (5), 22-(R)-hydroxycholesterol and riccardin C (3), were found to possess potent α-glucosidase-inhibitory activity. However, we succeeded in generating LXR-selective antagonists (18, 23) and an LXRα-selective antagonist (25), without α-glucosidase-inhibitory activity. On the other hand, these two activities of riccardin C (3) have been only partially separated by structural development. Thus, we next aimed to obtain specific α-glucosidase inhibitors without LXR-antagonistic activity.
PP2P(2), which has the phenethyl moiety at the 2’-position, showed relatively strong α-glucosidase inhibition with an IC50 value of 16.2 μM. Shift of the phenethyl moiety from the 2’-position to the 3’- or 4’-position elicited the disappearance of this inhibitory activity. Only compound (16), which has a 3’-phenethyl group bearing 3”- and 4”-OH groups, showed α-glucosidase-inhibitory activity with an IC50 value of 38 μM, while compound (20), which has an unsubstituted styryl group at the 4’-position, exhibited weak activity. Broadly speaking, 3’- and 4’-substituted compounds tend to lose α-glucosidase-inhibitory activity. Next, based on our previous finding that tetrachlorophthalimide derivatives tend to possess α-glucosidase-inhibitory activity, tetrachlorophthalimide analogs (27–49) were prepared and their activities were evaluated (Table 2). 4,5,6,7-Tetrachlorophthalimide derivatives showed higher α-glucosidase-inhibitory activity than unsubstituted phthalimide derivatives.
Lineweaver-Burk plot analysis of α-glucosidase-inhibitory activity for riccardin C (3),44 deoxynojirimycin (50)43 and compound (34) indicated that compound (34) is a non-competitive inhibitor, like riccardin C, whereas deoxynojirimycin is a competitive inhibitor.
3. Glycogen phosphorylase inhibitors13
Glycogen phosphorylase catalyzes breakdown of glycogen to glucose-1-phosphate, which is metabolized in the liver to glucose for delivery to the entire body. The liver accounts for ca. 90% of endogenous glucose production. This hepatic glucose production (HGP) is controlled by insulin. HGP is composed of glycogenolysis, in which a glucose monomer is released from glycogen, and gluconeogenesis, in which glucose is synthesized from lactate, amino acids and glycerol. Glycogenolysis is estimated to account for 12% to 75% of total HPG.45-47 Glycogen phosphorylase consists of two interconvertible forms, that is, glycogen phosphorylase a (GPa), which is the phosphorylated form (high activity, high substrate affinity), and glycogen phosphorylase b (GPb), which is the nonphosphorylated form (low activity, low substrate affinity).48 Therefore, inhibitors of GPa are expected to have potential application as novel antihyperglycemic agents. There are at least six known binding sites in GPa.49 The first class of nonphysiological inhibitors of glycogen phosphorylase comprises glucose analogues (e.g., spirohydantoin (51)50), which bind to the catalytic site of GPa. Another class of GPa inhibitors binding at the catalytic site is the iminosugars (e.g., 1,4-dideoxy-1,4-imino-D-arabinitol: DAB (11)).51-54
Using the multi-template approach, we have developed PP2P (2), which possesses LXR-antagonistic activity and α-glucosidase-inhibitory activity. α-Glucosidase catalyzes the final step in the digestion of carbohydrates. LXRs regulate important aspects of cholesterol homeostasis by not only controlling expression of their target genes, including the ATP binding cassette ABCA1 and cytochrome P450 7A (CYP7A) genes, but also acting as a glucose sensor. Our previous studies demonstrated the co-existence of α-glucosidase-inhibitory activity and LXR-antagonistic activity in various compounds, including phenethylphenylphthalimides Therefore, we speculated that the phenethylphenylphthalimide skeleton might be recognized by GPa, which acts on glycogen (vide supra).
Although PP2P (2), the lead for this series of compounds, showed potent α-glucosidase-inhibitory activity, it completely lacked glycogen phosphorylase-inhibitory activity under our experimental conditions. As mentioned above, two OH groups at the 3”- and 4”-positions seem to be essential for appearance of glycogen phosphorylase-inhibitory activity. Among unsubstituted phthalimide derivatives, none showed IC50 values below 100 μM, except compounds (19) and (20). However, compounds (13), (16), (19) and (22), which bear two OH groups at the 3”- and 4”- positions showed relatively high inhibition ratio at 100 μM among the tested compounds. In contrast, introduction of two methoxy groups at the same positions was ineffective. Furthermore, incorporation of one substituent was not effective. On the other hand, the 4,5,6,7-tetrachlorophthalimide unit was an effective skeleton to elicit high glycogen phosphorylase -inhibitory activity, and among tetrachlorophthalimide derivatives, introduction of two OH groups was also very effective; in these compounds, even two OMe units were effective. Here, the 4’-substituted phenylphthalimide derivative (19), which possesses OH groups at the 3”- and 4”-positions, exhibited the most potent glycogen phosphorylase-inhibitory activity, with an IC50 value of 2.7 μM. Several other compounds showed potent glycogen phosphorylase-inhibitory activity, and further development along this line is expected.
4. PPAR (peroxisome proliferator-activated receptor) agonists
PPARs are ligand-activated transcription factors belonging to the nuclear hormone receptor superfamily.55 They exist in three subtypes, PPARα, PPARδ (PPARβ), PPARγ. For all PPARs, lipids are endogenous ligands, linking them to metabolism.56 PPARs are recognized as generic lipid sensors translating nutritional signals into metabolic responses.57,58 PPARs activate transduction of their target genes as heterodimers with retinoic X receptors (RXR). The in vivo relevance of the various possible combinations of heterodimers and their functions in vitro remain unclear,59 but the PPAR response element, a direct repeat of the sequence AGGTCA spaced by a single nucleotide, is considered to play a key role.60 PPARs are important therapeutic targets, and specific ligands for α and γ are commercially available.
PPARα is necessary for the response to signals that induce peroxisome proliferation, hepatomegaly, and up-regulation of lipid metabolism. Knock-out mice do not reveal any gross defects at birth, are viable, and appear healthy.61 However, PPARα knock-out adult mice accumulate lipids in the liver, have problems regulating insulin secretion during fasting, develop skin disease and prolonged inflammatory responses, show increased adipose tissue mass, and develop spontaneous liver tumors.62-64 PPARα is mainly expressed in the liver, where it regulates free fatty acid (FFA) oxidation, and is also expressed to a lesser extent in the heart, skeletal muscle, small intestine, and kidney.62 PPARα-selective ligands, fenofibrate, bezafibrate, etc., have been on the market for many years to treat hyperlipidemia and decrease serum levels of triglyceride, which is one of the risk factors of metabolic syndrome.
PPARδ activation is thought to be a novel approach for the treatment of metabolic syndrome and associated cardiovascular disease. PPARδ is expressed in a variety of tissues, including skin, skeletal muscle, adipose tissue, heart, and various types of cancer. PPARδ knock-out embryos exhibit placental defects, and a substantial proportion of embryos do not survive,65,66 although some survive and appear healthy.67 PPARδ agonists are not yet in clinical use. Endogenous ligands for PPARδ include fatty acids, triglycerides, prostacyclin, and retinoic acid. A specific agonist, GW501516 (52),68 increased circulating HDL cholesterol levels and lowered triglyceride and insulin levels.69 These effects may explain why PPARδ activation prevented metabolic syndrome and obesity, and ameliorated insulin sensitivity.70-72
PPARγ is expressed in white and brown adipose tissue, the gut, and immune cells. It regulates adipocyte differentiation and lipid storage in white adipose tissue.55 Moreover, PPARγ coordinates glucose metabolism to improve insulin sensitivity.73 Thiazolidinediones such as pioglitazone (54) and rosiglitazone (55), which are full PPARγ agonists, are in clinical use for the treatment of type 2 diabetes. PPARγ is transcribed into three splice variants which generate two distinct protein isoforms.74 Adipocytes function as a regulator of systemic glucose and lipid homeostasis and PPARγ is responsible for control of adipogenesis.75 PPARγ-deficient mice display severe lipodystrophy, together with insulin resistance and hypotension.76 Point mutations in the ligand or DNA-binding domain of PPARγ elicit severe insulin resistance in human subjects.77 The major side effects are fluid retention and triglyceride accumulation.
The ligand-binding domains of nuclear receptors have a common overall three-dimensional structure.18 We have suggested that the phenethylphenylphthalimide skeleton might be a superior scaffold for the development of BRMs for a range of proteins involved in anti-diabetic activity. Thus, we attempted to generate PPAR agonists by the incorporation of aliphatic carboxylic acid units, adopted from fatty acids, which are endogenous ligands for PPARs, into phenethylphenylphthalimides (Figure 8). These compounds were synthesized as follows (Chart 1-3).
The PPAR-agonistic activities of the synthesized compounds are shown in Table 3. Although compound (57), which possesses a carboxyethyl unit, showed 53% activation of PPARα, 27% activation of PPARδ and 7% activation of PPARγ at the concentration of 100 μM, PPAR-agonistic activities of the synthesized compounds were generally weak. Compounds (56, 58 and 59), that have different chain lengths of aliphatic acid unit in their side chain, exhibited almost the same agonistic activities and subtype selectivities (56; α: 14%, δ: 0%, γ: 2%, 58; α: 4%, δ: 4%, γ: 7%, 59; α: 8%, δ: 4%, γ: 5%).
On the other hand, the compounds with ether structure in the linkage between the benzene ring and aliphatic carboxylic acid showed a slightly different structure-activity relationship. Compound (60), which has an oxy-acetic acid moiety, exhibited 41% activation of PPARα (δ: 10%, γ: 6%). On the other hand, compound (61), having an oxy-butanoic acid unit, showed good PPAR γ selectivity (61; α: 0%, δ: 1%, γ: 31%).
Mechanism-based side effects have limited the therapeutic potential of PPARs modulators. Development of more selective PPARs modulators has been expected to yield safer alternatives. However, from the viewpoint of obtaining better PPARs modulators without innate side effects, it may be a better strategy to aim for an optimum balance of modulating activities for PPARs for the treatment of diabetes and metabolic syndrome.
Biological activity evaluations of compounds (56-62), which possess a carboxylic acid moiety, for glycogen phosphorylase-inhibitory activity, LXRs-modulating activity and α-glucosidase-inhibitory activity are in progress. The tetrachlorophthaloyl derivatives (29, 32, 35 and 38), which bear two OH groups at the 3”- and 4”-positions, showed good activities against all these targets. Although PPAR-modulating activities have not been evaluated yet, compounds (29, 32, 35 and 38) appear to have good potential as diabetes- or metabolic syndrome-oriented multifunctional lead compounds.
CONCLUSIONS
We prepared a series of multifunctional modulators based on the multi-template approach inspired by thalidomide. The synthesized compounds showed LXR-antagonistic activity, α-glucosidase-inhibitory activity, glycogen phosphorylase-inhibitory activity and PPARs-modulating activity, and are candidate lead compounds for development of drugs to treat diabetes, hyperlipidemia and metabolic syndrome.
Recently, the use of drug combinations, such as amlodipine besylate/atorvastatin calcium (caduet) and ezetimibe/simvastatin (Vytorin), is being seen as a good approach to improve therapeutic efficacy. In the case of Vytorin, ezetimibe and simvastatin, both of which are cholesterol reducers, are mixed to avoid the toxicity that would be associated with an increased dose of either agent alone. In clinical practice, multiple drugs may prescribed in some cases to achieve a therapeutic goal, especially for chronic diseases.
We consider that the multi-template approach is a useful strategy to find multifunctional agents, i.e., single agents with well-balanced multiple activities. Integrated multifunctional drugs possessing an appropriate balance of moderate activities might be highly efficacious for the treatment of some conditions, without the possible side effects that may be associated with highly potent single agents.
ACKNOWLEDGEMENTS
The work described in this paper was partially supported by Grants-in Aid for Scientific Research from The Ministry of Education, Culture, Sports, Science and Technology, Japan, and a grant from the Japan Society for the Promotion of Science.
References
1. Y. Hashimoto, Arch. Pharm. Chem. Life Sci., 2008, 341, 536. CrossRef
2. Y. Hashimoto, Bioorg. Med. Chem., 2002, 10, 461. CrossRef
3. J. B. Bartlett, K. Dredge, and A. G. Dalgleish, Nat. Rev. Cancer, 2004, 4, 314. CrossRef
4. Y. Hashimoto, Curr. Med. Chem., 1998, 5, 163.
5. Y. Hashimoto, Mini–Rev. Med. Chem., 2002, 2, 543. CrossRef
6. Y. Hashimoto, A. Tanatani, K. Nagasawa, and H. Miyachi, Drugs Future, 2004, 29, 383. CrossRef
7. K. Motoshima, T. Noguchi-Yachide, K. Sugita, Y. Hashimoto, and M. Ishikawa, Bioorg. Med. Chem., 2009, 17, 5001. CrossRef
8. T. Noguchi-Yachide, A. Aoyama, M. Makishima, H. Miyachi, and Y. Hashimoto, Bioorg. Med. Chem. Lett., 2007, 17, 3957. CrossRef
9. T. Noguchi-Yachide, H. Miyachi, H. Aoyama, A. Aoyama, M. Makishma, and Y. Hashimoto, Chem. Pharm. Bull., 2007, 55, 1750. CrossRef
10. S. Sou, S. Mayumi, H. Takahashim, R. Yamasaki, S. Kadoya, M. Sodeoka, and Y. Hashimoto, Bioorg. Med. Chem. Lett., 2000, 10, 1081. CrossRef
11. S. Sou, H. Takahashi, R. Yamasaki, H. Kagechika, Y. Endo, and Y. Hashimoto, Chem. Pharm. Bull., 2001, 49, 791. CrossRef
12. H. Takahashi, S. Sou, R. Yamasaki, M. Sodeoka, and Y. Hashimoto, Chem. Pharm. Bull., 2000, 48, 1494.
13. K. Motoshima, M. Ishikawa, K. Sugita, and Y. Hashimoto, Biol. Pharm. Bull., 2009, 32, 1618. CrossRef
14. E. V. Koonin, Y. I. Wolf, and G. P. Karev, Nature, 2002, 420, 218. CrossRef
15. N. V. Grishin, J. Struct. Biol., 2001, 134, 167. CrossRef
16. M. A. Koch, L.-O. Wittenberg, S. Basu, D. A. Jeyaraj, E. Gourzoulidou, K. Reinecke, A. Odermatt, and H. Waldmann, Proc. Natl. Acad. Sci. USA, 2004, 101, 16721. CrossRef
17. P. H. Jones, Am. J. Cardiol., 2008, 102(suppl), 41L. CrossRef
18. H. Gronemeyer, J.-A. Gustafsson, and V. Laudet, Nat. Rev. Drug Discovery, 2004, 3, 950. CrossRef
19. C. Zhao and K. Dahlman-Wright, J. Endcrinol., 2010, 204, 233. CrossRef
20. T. M. Stulnig, K. R. Steffensen, H. Gao, M. Reimers, K. Dahlman-Wright, G. U. Schuster, and J.-Å. Gustafsson, Mol. Pharmacol., 2002, 62, 1299. CrossRef
21. S. M. Ulven, K. T. Dalen, J.-Å. Gustafsson, and H. I. Nebb, J. Lipid Res., 2004, 45, 2052. CrossRef
22. A. Grefhorst, T. H. van Dijk, A. Hammer, F. H. van der Sluijs, R. Havinga, L. M. Havekes, J. A. Romijn, P. H. Groot, D.-J. Reijngoud, and F. Kuipers, Am. J. Physiol. Endocrinol. Metab., 2005, 289, E829. CrossRef
23. B. A. Laffitte, L. C. Chao, J. Li, R. Walczak, S. Hummasti, S. B. Joseph, A. Castrillo, D. C. Wilpitz, D. J. Mangelsdorf, J. L. Collins, E. Saez, and P. Tontonoz, Proc. Natl. Acad. Sci. USA, 2003, 100, 5419. CrossRef
24. S. R. Commerford, L. Vargas, S. E. Dorfman, N. Mitro, E. C. Rocheford, P. A. Mak, X. Li, P. Kennedy, T. L. Mullarkey, and E. Saez, Mol. Endocrinol., 2007, 21, 3002. CrossRef
25. K. T. Dalen, S. M. Ulven, K. Bamberg, J.- Å. Gustafsson, and H. I. Nebb, J. Biol. Chem., 2003, 278, 48283. CrossRef
26. S. Frenández-Veledo, I. Nieto-Vazquez, C. M. Rondinone, and M. Lorenzo, Diabetologia, 2006, 49, 3038. CrossRef
27. B. A. Janowski, M. J. Grogan, S. A. Jones, G. B. Wisely, S. A. Kliewer, E. J. Corey, and D. J. Mangelsdorf, Proc. Natl. Acad. Sci. USA, 1999, 96, 266. CrossRef
28. J. R. Schultz, H. Tu, A. Luk, J. J. Repa, J. C. Medina, L. Li, S. Schwendner, S. Wang, M. Thoolen, D. J. Mangelsdorf, K. D. Lustig, and B. Shan, Genes Dev., 2000, 14, 2831. CrossRef
29. J. L. Collins, A. M. Fivush, M. A. Watson, C. M. Galardi, M. C. Lewis, L. B. Moore, D. J. Parks, J. G. Wilson, T. K. Tippin, J. G. Binz, K. D. Plunket, D. G. Morgan, E. J. Beaudet, K. D. Whitney, S. A. Kliewer, and T. M. Willson, J. Med. Chem., 2002, 45, 1963. CrossRef
30. J. D. Horton, J. L. Goldstein, and M. S. Brown, J. Clin. Investig., 2002, 109, 1125. CrossRef
31. S. B. Joseph, B. A. Laffitte, P. H. Patel, Michael. A. Watson, K. E. Matsukuma, R. Walczak, J. L. Collins, T. F. Osborne, and P. Tontonoz, J. Biol. Chem., 2002, 277, 11019. CrossRef
32. K. Iizuka and Y. Horikawa, Endocrine J., 2008, 55, 617. CrossRef
33. R. Dentin, F. Benhamed, I. Hainault, V. Fauveau, F. Foufelle, J. R. B. Dyck, J. Girard, and C. Postic, Diabetes, 2006, 55, 2159. CrossRef
34. J. J. Repa and D. J. Mangelsdorf, Annu. Rev. Cell Dev. Biol., 2000, 16, 459. CrossRef
35. S. Alberti, K. R. Steffensen, and J.-Å. Gustafsson, Gene, 2000, 243, 93. CrossRef
36. S. Williams, R. K. Bledsoe, J. L. Collins, S. Boggs, M. H. Lambert, A. B. Miller, J. Moore, D. D. McKee, L. Moore, J. Nichols, D. Parks M. Watson, B. Wisely, and T. M. Willson, J. Biol. Chem., 2003, 278, 27138. CrossRef
37. B. Hu, E. Quinet, R. Unwalla, M. Collini, J. Jetter, R. Dooley, D. Andraka, L. Nogle, D. Savio, A. Halpern, A. Goos-Nilsson, A. Wihelmsson, P. Nambi, and J. Wrobel, Bioorg. Med. Chem. Lett., 2008, 18, 54. CrossRef
38. M. R. Stam, E. G. J. Danchin, C. Rancurel, P. M. Coutinho, and B. Henrissat, Protein Eng., Des. Sel., 2006, 19, 555. CrossRef
39. C. Yu, A. M. Lee, B. L. Bassler, and S. Roseman, J. Biol. Chem., 1991, 266, 24260.
40. G. Legler, Adv. Carbohydr. Chem. Biochem., 1990, 48, 319. CrossRef
41. M. yoshikawa, H. Shimada, N. Nishida, Y. Li, I. Toguchida, J. Yamahara, and H. Matsuda, Chem. Pharm. Bull., 1998, 46, 113.
42. Y. Moritoh, K. Takeuchi, and M. Hazama, J. Pharmacol. Exp. Ther., 2009, 329, 669. CrossRef
43. D. Breitmeier, S. Günther, and H. Heymann, Arch. Biochem. Biophys., 1997, 346, 7. CrossRef
44. K. Dodo, A. Aoyama, T. Noguchi-Yachide, M. Makishima, H. Miyachi, and Y. Hashimoto, Bioorg. Med. Chem., 2008, 16, 4272. CrossRef
45. M. Roden and E. Bernroider, Best Pract. Res. Clin. Endocrinol. Metab., 2003, 17 365. CrossRef
46. R. Kurukulasuriya, J. T. Link, D. J. Madar, Z. Pei, J. J. Rohde, S. J. Richards, A. J. Souers, and B. G. Szczepankiewicz, Curr. Med. Chem., 2003, 10, 99.
47. P. Staehr, O. Hother-Nielsen, and H. Beck-Nielsen, Diabetes Obes. Metab., 2002, 4 215. CrossRef
48. L. N. Johnson, FASEB J., 1992, 6, 2274.
49. N. G. Oikonomakos and L. Somsák, Curr. Opin. Investig. Drugs, 2008, 9, 379.
50. C. J. F. Bichard, E. P. Mitchell, M. R. Wormald, K. A. Watson, L. N. Johnson, S. E. Zographos, D. D. Koutra, N. G. Oikonomakos, and G. W. J. Fleet, Tetrahedron Lett., 1995, 54, 2145. CrossRef
51. P. Jakobsen, J. M. Lundbeck, M. Kristiansen, J. Breinholt, H. Demuth, J. Pawlas, M. P. T. Candela, B. Andersen, N. Westergaard, K. Lundgren, and N. Asano, Bioorg. Med. Chem., 2001, 9, 733. CrossRef
52. K. Fosgerau, N. Westergaard, B. Quistorff, N. Grunnet, M. Kristiansen, and K. Lundgren, Arch. Biochem. Biophys., 2000, 380, 274. CrossRef
53. B. Andersen and N. Westergaard, Biochem. J., 2002, 367, 443. CrossRef
54. N. G. Oikonomakos, C. Tiraidis, D. D. Leonidas, S. E. Zographos, M. Kristiansen, C. U. Jessen, L. Nørskov-Lauritsen, and L. Agius, J. Med. Chem., 2006, 49, 5687. CrossRef
55. J. N. Feige, L. Gelman, L. Michalik, B. Desvergne, and W. Wahli, Prog. Lipid Res., 2006, 45, 120. CrossRef
56. L. Michalik, J. Auwerx, J. P. Berger, V. K. Chatterjee, C. K. Glass, F. J. Gonzalez, P. A. Grimaldi, T. Kadowaki, M. A. Lazar, S. O'Rahilly, C. N. A. Palmer, J. Plutzky, J. K. Reddy, B. M. Spiegelman, B, Staels, and W. Wahli, Pharmacol Rev., 2006, 58, 726. CrossRef
57. H. A. Hostetler, A. D. Petrescu, A. B. Kier, and F. Schroeder, J. Biol. Chem., 2005, 280, 18667. CrossRef
58. H. A. Hostetler, A. B. Kier, and F. Schroeder, Biochemistry, 2006, 45, 7669. CrossRef
59. H. Keller, C. Dreyer, J. Medin, A. Mahfoudi, K. Ozato, and W. Wahli, Proc. Natl. Acad. Sci. U S A, 1993, 90, 2160. CrossRef
60. C. N. Palmer, M. H. Hsu, H. J. Griffin, and E. F. Johnson, J. Biol. Chem., 1995, 270, 16114. CrossRef
61. S. S. Lee, T. Pineau, J. Drago, E. J. Lee, J. W. Owens, D. L. Kroetz, P. M. Fernandez-Salguero, H. Westphal, and F. J. Gonzalez, Mol. Cell. Biol., 1995, 15, 3012.
62. P. Lefebvre, G. Chinetti, J.-C. Fruchart, and B. Staels, J. Clin. Invest., 2006, 116, 571. CrossRef
63. P. R. Devchand, H. Keller, J. M. Peters, M. Vazquez, F. J. Gonzalez, and W. Wahli, Nature, 1996, 384, 39. CrossRef
64. P. Howroyd, C. Swanson, C. Dunn, R. C. Cattley, and J. C. Corton, Toxicol. Pathol., 2004, 32, 591. CrossRef
65. O. Braissant, F. Foufelle, C. Scotto, M. Dauça, and W. Wahli, Endocrinology, 1996, 137, 354. CrossRef
66. Y. Barak, D. Liao, W. He, E. S. Ong, M. C. Nelson, J. M. Olefsky, R. Boland, and R. M. Evans, Proc. Natl. Acad. Sci. U S A, 2002, 99. 303. CrossRef
67. Y. Barak and S. Kim, PPAR Res., 2007, 12781.
68. M. L. Sznaidman, C. D. Haffner, P. R. Maloney, A. Fivush, E. Chao, D. Goreham, M. L. Sierra, C. LeGrumelec, H. E. Xu, V. G. Montana, M. H. Lambert, T. M. Willson, W. R. Oliver, Jr., and D. D. Sternbach, Bioorg. Med. Chem. Lett., 2003, 13, 1517. CrossRef
69. W. R. Jr. Oliver, J. L. Shenk, M. R. Snaith, C. S. Russell, K. D. Plunket, N. L. Bodkin, M. C. Lewis, D. A. Winegar, M. L. Sznaidman, M. H. Lambert, H. E. Xu, D. D. Sternbach, S. A. Kliewer, B. C. Hansen, and T. M. Willson, Proc. Natl. Acad. Sci. U S A, 2001, 98, 5306. CrossRef
70. T. Tanaka, J. Yamamoto, S. Iwasaki, H. Asaba, H. Hamura, Y. Ikeda, M. Watanabe, K. Magoori, R. X. Ioka, K. Tachibana, Y. Watanabe, Y. Uchiyama, K. Sumi, H. Iguchi, S. Ito, T. Doi, T. Hamakubo, M. Naito, J. Auwerx, M. Yanagisawa, T. Kodama, and J. Sakai, Proc Natl. Acad. Sci. U S A, 2003, 100, 15924. CrossRef
71. Y. X. Wang, C.-H. Lee, S. Tiep, R. T. Yu, J. Ham, H. Kang, and R. M. Evans, Cell, 2003, 113, 159. CrossRef
72. C.-H. Lee, P. Olson, A. Hevener, I. Mehl, L.-W. Chong, J. M.Olefsky, F. J. Gonzalez, J. Ham, H. Kang, J. M. Peters, and R. M. Evans, Proc. Natl. Acad. Sci. U S A, 2006, 103, 3444. CrossRef
73. W. He, Y. Barak, A. Hevener, P. Olson, D. Liao, J. Le, M. Nelson, E. Ong, J. M. Olefsky, and R. M. Evans, Proc. Natl. Acad. Sci. U S A, 2003, 100, 15712. CrossRef
74. L. Fajas, J. C. Fruchart, and J. Auwerx, FEBS Lett., 1998, 438, 55. CrossRef
75. C. Christodoulides and A. Vidal-Puig, Mol. Cell. Endocrinol., 2010, 318, 61. CrossRef
76. S. Z. Duan, C. Y. Ivaschenko, S. E. Whitesall, L. G. D’Alecy, D. C. Duquaine, F. C. Brosius III, F. J. Gonzalez, C. Vinson, M. A. Pierre, D. S. Milstone, and R. M. Mortensen, J. Clin. Invest., 2007, 117 , 812. CrossRef
77. M. Agostini, E. Schoenmakers, C. Mitchell, I. Szatmari, D. Savage, A. Smith, O. Rajanayagam, R. Semple, J. Luan, L. Bath, A. Zalin, M. Labib, S. Kumar, H. Simpson, D. Blom, D. Marais, J. Schwabe, I. Barroso, R. Trembath, N. Wareham, L. Nagy, M. Gurnell, S. O'Rahilly, and K. Chatterjee, Cell Metab., 2006, 4, 303 CrossRef