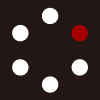
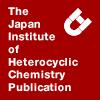
HETEROCYCLES
An International Journal for Reviews and Communications in Heterocyclic ChemistryWeb Edition ISSN: 1881-0942
Published online by The Japan Institute of Heterocyclic Chemistry
e-Journal
Full Text HTML
Received, 16th June, 2010, Accepted, 4th August, 2010, Published online, 10th August, 2010.
DOI: 10.3987/REV-10-SR(E)3
■ Development and Applications of an Oxazole-Forming Reaction
Jianmin Zhang,* Pierre-Yves Coqueron, and Marco A. Ciufolini*
Department of Chemistry, University of British Columbia, 2036 Main Mall, Vancouver B.C., V6T 1Z1, Canada
Abstract
We review an oxazole-forming reaction devised in our laboratory. The technique allows the facile assembly of 2,5-disubstituted-4-carbalkoxy oxazoles, including 5-amino and 5-alkenyl oxazoles. Applications of this chemistry in the total synthesis of muscoride A, siphonazoles, and other oxazole alkaloids are described.INTRODUCTION
Heterocyclic chemistry provides considerable opportunity for the development of new synthetic methods. Indeed, the bioactivity of many heterocycles and the noteworthy architecture of numerous heterocyclic natural products often translate into interesting challenges in the chemical domain. This is certainly the case for a group of natural products that incorporate consecutive 2,4,5-trisubstituted oxazole subunits of the type 1 (Scheme 1). Representative examples of such nitrogenous substances include muscoride A, 2,1 diazonamides, 3-4,2 and telomestatin, 5 (Scheme 2).3 It should be noted that while segments 1 are fairly rare among natural products,4 they occur frequently in medicinal agents. For instance, since the beginning of 2010, no fewer than 8 disclosures have described the therapeutic effects of chemical entities incorporating such moieties.5
We became interested in natural and non-natural substances that exhibit multiple consecutive copies of subunit 1. This induced us to ideate an iterative oxazole-forming reaction that would function as seen in Scheme 3. Thus, an appropriate sequence would elaborate a generic primary carboxamide 6 to an oxazole, 7, which displays a C-4 carbalkoxy group. Conversion of the latter into a primary amido substituent would enable a second cycle of the sequence, leading to a bis-oxazole 9, also exhibiting a C-4 CONH2 substituent. Multiple iterations of the cycle would produce a polyoxazole construct. Such a methodology was anticipated to be useful both in synthetic organic and in medicinal chemistry. In this paper, we review salient aspects of this chemistry and we discuss selected applications in natural product synthesis and in drug research.
DEVELOPMENT OF AN ITERATIVE OXAZOLE-FORMING REACTION
A number of literature precedents influenced the manner in which the surmise pictured in Scheme 3
was translated into practice. Deryckere,6 Hacksell,7 and Wipf,8 had shown that N-propargylic amides 10 (R3 = H or occasionally alkyl) undergo cycloisomerization to oxazoles 11 (Scheme 4) under Lewis acidic6 or, more commonly, basic7,8 conditions.9 However, 11 lack a requisite 4-COOR substituent. Oxazoles incorporating such a group emerge upon cyclization of alkynylglycinates 12, as demonstrated by Nagao.10 Nonetheless, the Nagao synthesis of 12 is fairly laborious. A straightforward route to 12 was described later by Williams (cf. 14 → 12).11 We not only reproduced these reactions effortlessly, but we also found that by extending the contact time to ca. 4h one could induce in situ cycloisomerization of the transient 12 to oxazoles 13, which thus become the virtually exclusive products of the reaction (50-55% yield).12 Unfortunately, the reliance of this chemistry upon alkynylstannane building blocks overshadowed its usefulness. The risk of organotin contamination of downstream products precluded the use of such organometallics for medicinal chemistry research. What we required was a way to achieve a Williams alkynylglycinate construction with acetylenic nucleophiles incorporating a nontoxic metal, with the presumption that a subsequent cycloisomerization of the resultant 12 would produce the desired oxazoles.
A few words about chloroglycinates 17 are in order at this point. These substances are readily available by the method of Ben-Ishai,13 which entails the initial combination of a primary amide (or carbamate) with ethyl glyoxylate, followed by SOCl2 treatment of the adduct 16 (Scheme 5). They are reactive, water-sensitive, and are difficult to purify. Fortunately, they emerge in a state of satisfactory purity and are best used in crude form. They are also storable for several months at –20 °C if adequately protected from moisture. Scheme 5 depicts our preferred method to prepare such chloroglycinates, but other routes are known. For instance, Williams obtained 14 by reaction of 19 with PCl5. In turn, 19 results when the adduct of a primary amide (or carbamate) with glyoxylic acid
adduct of a primary amide (or carbamate) with glyoxylic acid hydrate is treated with MeOH and catalytic H2SO4. This induces simultaneous methyl ether formation/Fischer esterification. It is worthy of note only Fischer esterification occurs when MeOH is replaced with a higher alcohol, e.g., n-butanol (cf. 94% yield of 20, R1 = Ph).12 Compounds 20 thus become accessible even if the corresponding glyoxylic ester is unavailable, and subsquent SOCl2 treatment advances them to the expected chloroglycinates (Scheme 6).
The ready availability of chloroglycinate educts induced us to study their Metcalf-type condensation14 with the trimethylsilyl derivative of terminal alkynes under the influence of AlCl3. While analogous chloro substrates combine efficiently with bis-TMS acetylene under these conditions, other 1-TMS-1-alkynes failed to react (Table 1). Equally disappointing results were obtained when we attempted to induce a Petasis-like condensation15 of 21b, 21e, or 21f with alkynylboronic esters, with or without Lewis acid promoters. A glimmer of hope initially provided by the reaction of 21g with trialkynylboranes, prepared in situ from lithium acetylides and BBr3, soon vanished when we found ourselves unable to obtain the desired 22g in greater than 20% yield. Exposure of 21 to alkali metal acetylides (Li, Na, K) triggered rapid degradation to intractable polymeric materials. Some 22 emerged in a disastrous 5% yield from the reaction of 21 with a Li acetylide in the presence of ZnCl2 or BF3OEt2.
Such a lugubrious ingemination of negative results was finally interrupted when, inspired by the work of Negishi16 and Katritzky,17 we evaluated alkynylaluminum nucleophiles. We were delighted to find that a reagent produced upon the interaction of lithiated 1-octyne and Me2AlCl in THF, at –78 °C — arguably, a dimethylaluminum acetylide — rapidly converted the foregoing chloroglycinates into alkynylglycinates in high yield (cf. entry k). Moreover, cycloisomerization of the latter to the corresponding oxazoles occurred in situ if the temperature was allowed to increase to 25 °C before workup (ca. 2-4 hrs), or if the reaction was worked up under gently basic conditions (aq. NaHCO3).
An oxazole-forming reaction proceeding through the union of a primary amide, a glyoxylic ester, and a terminal acetylene, had thus materialized.18 Table 2 provides a summary of representative oxazoles obtained by this technique. It should be noted that the listed yields refer to final products purified by column chromatography. Because chloroglycinates 23 were employed in crude form, such yields reflect substantially the overall efficiency of the conversion of primary amides 15 into the corresponding oxazoles.
The following key points define the scope and the known limitations of the process. Most primary amides — whether aromatic, aliphatic, or aminoacid-derived — may be utilized in the reaction. Higher esters of the glyoxylic portion (cf. n-Bu vs. Et) tend to afford better yields. Aminoacid-derived substrates participate in the reaction with no erosion of sterochemical integrity, as determined by the scrutiny of 1H and 13C NMR spectra of the (R)-α-methylbenzylamide of oxazoles 24j-k.19 A phthalimide protecting group (cf. 24j) is well tolerated. However, chloroglycinates derived from α,β-unsaturated amides are poor substrates, affording oxazoles in substantially diminished yields. Thioether functionalities are tolerated (cf. 24n). Conversely, no oxazole was detected among the products originating from chloroglycinate substrates in which group R1 was equal to ClCH2 or (MeO)2P(O)CH2.
The dimethylaluminum derivative of methyl propargy ether reacted with 25 to provide a mixture of the expected 5-(2-methoxylethyl) oxazole plus the corresponding 5-vinyloxazole. For example, the reaction of 25 with (3-methoxyprop-1-ynyl)dimethylaluminum returned 26 (51% yield) and 27 (21% yield; Scheme 7). Similar behavior was observed in the reaction of entry j of Table 2. The formation of 27 is clearly attributable to a formal loss of methanol from the product; however, experiment revealed that such an eliminative process is unlikely to evolve from 26. Indeed, exposure of 5-(2-methoxylethyl) oxazole of the type 26 to the action of strong bases (LDA, KHMDS) failed to produce any vinyl counterpart.20 Evidently, loss of MeOH occurs prior to oxazole formation. The work of Wipf8 suggests the mechanistic picture outlined in Scheme 8 for the origin of 27 and for the overall conversion of alkynylglycinates into oxazoles. The initial product of halogen substitution, 28, is reversibly deprotonated under the basic conditions of the reaction. Protonation of transient anion 29 at the distal position affords cumulene 30 (never detected in our reaction). Disrotatory electrocyclization of such a 6-electron systems and prototropic tautomerism afford 32. We believe this to be the mechanism by which all alkynylglycinates isomerize to oxazoles.
On the other hand, the departure of the propargylic nucleofuge (MeO in the case of 29) results in formation of cumulene 31 (also not detected), which then advances to vinyloxazole 33 by an analogous electrocyclization/tautomerization sequence.
Curiously, the 5-alkenyl-2-alkyl-4-carbalkoxy oxazole motif embodied in 33 has been scantily documented. Generic 5-alkenyloxazoles have been prepared by cycloisomerization of appropriate N-propargylamides,8 Pd-mediated coupling reactions,21 dehydration of alcohols obtained by reduction of 5-acyloxazoles,22 "long range Pummerer cyclization" of certain sulfur-substituted propargylamides,23 olefin metathesis of preformed 5-alkenyloxazoles,24 and Wittig reaction of 5-formyloxazoles.25 The 5-vinyloxazole system is particularly rare, a mere 17 examples of it having been recorded in the CAS database as of this writing.26 On the other hand, 5-alkenyloxazoles in general, and especially compounds 33, are clearly valuable building blocks in heterocyclic chemistry. In addition, they appear as subunits of particular β-lactam antibiotics27 and antiviral agents.28 This induced us to establish improved routes to the 5-alkenyl series based on the new oxazole-forming process.
When the chemistry of Scheme 7 was implemented by using a dimethylalkynyl aluminum reagent derived from phenyl propargyl ether, the result was the virtually exclusive formation of 5-vinyl oxazoles, albeit in moderate yield.29 Representative examples are listed in Table 3, which underscores the fact that chloroglycinates derived from α,β-unstaurated amides react poorly in this transformation (entry g). Evidently, the greater nucleofugal character of PhO(–) relative to MeO(–) favored the eliminative pathway leading from 29 to 31, and thence to 33.30 We note also that presumed aluminum reagents produced from lithiated propargyl chloride afforded mixtures of products containing no oxazole.
The facile access to 5-trimethylsilylmethyl oxazoles provided by the chemistry of Table 2 (entries c and g-n) permitted the development of a Peterson avenue to more complex 5-alkenyl oxazoles as
delineated in Scheme 9.31 Deprotonation of oxazoles 35 afforded a benzylogous silicon-stabilized enolate, 36, which was relatively unreactive, even toward aldehydes. Accordingly, the addition of 36 to aldehydes was carried out in the presence of TiCl4. Experiment revealed that substrates in which R1
is an aromatic group reacted best when lithiation was effected with LDA, while LHMDS was often the base of choice if R1 was a small alkyl such as a methyl group. On the other hand, while the strongly Lewis acidic TiCl4 favored carbonyl addition, it retarded the (formal) elimination of TMS-OH from the adducts,32 and promoted the formation of mixtures of 37 and 38. Complete conversion of 37 into 38 was achieved by treatment of the crude mixture with TsOH.33 The ultimate products 38 were obtained as mixtures of E and Z geometric isomers, the ratio of which appeared to depend on the nature of the aldehyde. Fortunately, nearly complete (> 95:5) isomerization to the E-alkene was observed upon refluxing the mixture of olefin isomers in toluene containing a catalytic amount of I2.34 Representative examples are listed in Table 4.
The facile formation of oxazole 24n provided us with an opportunity to revisit a long-standing problem: the Julia olefination35 of carbonyl compounds with the corresponding sulfone (Scheme 10). This seemingly trivial transformation has eluded the chemical community for decades, and this for a number of reasons.36 In particular, the anion of 2-sulfonylmethyloxazole-4-carboxylates (cf. 24n) is an infamously poor nucleophile, failing to add even to aldehydes.37 A solution to this problem was anticipated to have favorable ramifications in drug research. Indeed, 2-styryloxazoles of the type 41, R1 = aryl, appear as subunits of a number of bioactive molecules, including kinase inhibitors,38 anti-HIV agents,39 PPAR agonists,40 MCP-1 receptor antagonists,41 antiobesity/antidiabetic agents,42 and anthelmintic substances.43 A route to such motifs from 24n via oxazoles 40 would surely be a welcome addition to the synthetic armamentary of the medicinal chemist.
With these objectives in mind, oxazole 24n was desilylated and oxidized to the corresponding sulfone 42 (Scheme 11). Not unexpectedly, the anion of 42 failed to add to carbonyl compounds. However, smooth Knoevenagel condensation of 42 with aldehydes and even with ketones occurred in the presence of TiCl4.44 Aromatic aldehydes reacted best at room temperature in THF-CH2Cl2 in the presence of Et3N. Products 44 formed mostly or exclusively as the E-isomers, consistent with the relative steric demand of an aryl, phenylsulfonyl, and 2-oxazolyl substituents.45 Several examples appear in Table 5.
Reactions involving aliphatic aldehydes proceeded best when the carbonyl substrate and TiCl4 were added to a cold (–78 °C) solution of lithiated 42, prepared in advance by treatment with LHMDS,
followed by gradual warming to room temperature. Enolizable aldehydes afforded mixtures of 45 (Table 6, major product, E-isomer only) and its deconjugated isomer 46 (E-isomer only), except propionaldehyde (entry b), which delivered only a product of the type 45. Isomers 45 and 46 were readily separable by silica gel chromatography and were characterized independently. They were also prone to vinylsulfone-allylsulfone isomerization,46 and care had to be taken to avoid this facile transposition. Identical operating conditions proved to be optimal for the reaction of ketones as well, except that no deconjugated products were observed with these substrates. Even unreactive acetophenone afforded the expected product in a respectable 44% yield. On the other hand, unsymmetrical ketones afforded mixtures of unassigned geometric isomers (Table 7; entries b and d).47
Whereas sulfones 44-47 may be valuable intermediates on their own right, a true olefination sequence required a simple, mild technique for their desulfonylation.48 Happily, aldehyde-derived 44-45 underwent facile reductive desulfonylation by treatment with metallic Zn and aqueous NH4Cl in refluxing THF (Scheme 12).44 The use of Zn/NH4Cl for the desulfonylation of compounds such as
44-45 was undocumented prior to our own work. No vinylsulfone-allylsulfone isomerization was detected during the reduction of 45 under such mild conditions.
The primary products of such reductive processes apparently formed with retention of olefin geometry; i.e. as the Z-isomers. Prolonged contact times (ca. 6 h) caused virtually complete isomerization of materials derived from aromatic aldehydes to the E-isomers. Substances emanating from aliphatic aldehydes isomerized more slowly, and were obtained as ca. 3:1 mixtures of Z (major) and E isomers. Table 8 summarizes the results of representive such desulfonylation-isomerization experiments. As for vinylsulfones 47, the Zn/NH4Cl system was effective only for the desulfonylation of products in which an aryl substituent was present on the olefin. For instance, 47d afforded 50 in high yield and as the E-isomer only (Scheme 13). By contrast, the desulfonylation of other ketone-derived products required the more vigorous reductant, Na/Hg amalgam, which is an established reagent for such transformations.48
These reactions were best conducted at –15 °C for a short time (40 min.) to avoid overreduction. Yields were sensibly lower than in the previous case, as evident from Table 9. We presume that the difference in reactivity between 47d and 47a-c reflects relative LUMO energies: lower for 47d thanks to phenyl conjugation; higher for 47a-c due to the electron-releasing effect of alkyl substituents R1 and R2. Electron transfer into the less energetic LUMO is efficient with a weaker reductant such as metallic Zn; electron transfer into the more energetic LUMO requires a stronger reducing agent such as Na/Hg amalgam.
The iterative mode of oxazole assembly was demonstrated as shown in Schemes 14 and 15. Thus, 24a and 26 were converted into the corresponding primary amides. These proved to be perfectly competent in a second round of the oxazole-forming reaction, leading to the production of bis-oxazoles 54 and 57 in 65-80% yield. Additional examples of bis-oxazole construction will be shown in the section dealing with applications in natural product synthesis. We emphasize that in the course of experiments involving aminoacid-derived materials, great care was exercised to verify that no erosion of stereochemical integrity had taken place during the various transformations. Typically, this entailed
the condensation (EDCI/HOBt) of oxazole carboxylic acids with (R)-methylbenzylamine, and careful scrutiny (1H and 13C NMR) of the resulting amide for diasteromeric homogeneity. No loss of optical purity was ever detected.
The favorable outcome of the foregoing investigations, as well as the work of Deyrup,49 induced us to examine the reaction of chloroglycinates with isonitriles and cyanide ion, leading to the formation of 5-aminooxazoles (Scheme 16). Of course, the use of isonitriles as building blocks in oxazole synthesis
is well documented,50 albeit not in the manner of Scheme 16. Accordingly, this chemistry would nicely complement existing alternatives.51 Initial experiments determined that chloroglycinates do not combine with isonitriles in the absence of Lewis acid promoters. Among the latter, AlCl3 and ZnI2 were ineffective, ZnCl2 was moderately efficacious,12 and Me2AlCl proved to be outstanding, inducing the formation of the desired 5-alkylaminooxazoles in good to excellent yield (Table 10).52
The bioactivity of 5-aminooxazoles and their derivatives induced us to explore an automated synthesis using a robot such as the Chemspeed® system. For such a purpose, we elected to employ of the safer, if less efficacious, ZnCl2 in lieu of the more effective, but pyrophoric, Me2AlCl promoter. The Chemspeed® instrument, essentially a dispenser of liquids, would combine THF solutions of ZnCl2, isonitriles, and chloroglycinates, then perform an extractive workup of the various reactions and purify the crude product by HPLC- MS.53 We emphasize that neither the size of the library nor the yield of the final oxazole were of significant import at this juncture. Our objective was to demonstrate an automated route to oxazoles 60 of sufficient purity to permit screening.
We thus chose to count as successful those reactions that afforded an oxazole of greater than 85% purity (HPLC-MS) in greater than 10% yield. The results of a parallel synthesis involving 5 chloroglycinates and 5 isonitriles are summarized in Table 11. A check mark indicates a successful reaction according to the foregoing criteria; a dash, that the reaction failed. It is apparent that two thirds of these automated syntheses were successful.12
Turning now to the formation of 5-aminooxazoles, we found that exposure of chloroglycinates to the action of Zn(CN)2, KCN, LiCN, ZnCl2/KCN; ZnCl2/TMSCN; Et3N/KCN in THF, in a range of temperatures between 25 °C and reflux, failed to give any of the target heterocycles.
However, efficient formation of the desired products ensued upon treatment with 3 equivalents of Et2AlCN (Nagata reagent)54 in THF, at rt. Table 12 provides representative examples of this transformation. An application of this chemistry was demonstrated through a synthesis of 66 (Scheme 17), a moderately potent inhibitor of Raf kinase,55 and a possible point of departure for the development of new antitumor drugs.
OXAZOLE FORMATION USING ALKYNYL GRIGNARDS: AN EXCEPTIONAL CASE
In one case uncovered thus far, an alkynyl Grignard reagent outperformed the corrsponding organoalane in the oxazole-forming reaction. Ongoing studies toward telomestatin require compound 71 (Scheme 19) as the appropriate carrier of the bis-5-methyloxazole segment of the natural product.
Experiments aiming to fashion the material in question from protected serine 6756 (Scheme 18) revealed that the reaction of chloroglycinate 68 with the dimethylaluminum derivative of TMS-acetylene was inefficient, affording the desired 69 in only 23% yield. The yield of this step doubled when the reaction was carried out with the corresponding acetylenic Grignard reagent. Notice how the LiOH workup of the oxazole-forming step serves to induce three distinct reactions: the complete cycloisomerization of the intermediate alkynylglycinate, the protiodesilylation of the primary product, and the saponification of the ester. Compound 69 was then elaborated to the requisite 71 as detailed in Scheme 19,57 which demonstrates yet another iterative application of the oxazole-forming reaction.
APPLICATIONS IN NATURAL PRODUCT SYNTHESIS
An initial application of the new chemistry was demonstrated in the context of a total synthesis of the weak antibiotic, muscoride A, 2.18 This effort commenced (Scheme 20) with the elaboration of
(L)-proline to amide 15k as a prelude to the execution of the first oxazole-forming sequence, which culminated with LiOH treatment of the nascent 24k, providing acid 73 via simultaneous ester saponification/protiodesilylation. The acid was then converted into amide 74, which was processed through a second oxazole iteration, as per Scheme 21. This surrendered acid 76, which was esterified with prenyl alcohol, as required for the natural product. Finally, the Troc group was released under neutral conditions by the use of Cd/Pb couple,58 and the synthesis was completed by acylation of 78 with activated ester 79 as described earlier by Wipf.1
A more recent effort has centered on the total synthesis of siphonazoles, 80-81, which are structurally unusual bis-oxazole substances displaying a two-carbon bridge between the heterocyclic moieties.59 Prior to their discovery, only compounds featuring directly linked oxazoles,60 or oxazoles bridged by a single carbon atom,61 were known among natural products. The structure of 80-81 evokes a possible iterative approach, wherein two copies of synthon 82 would merge with aldehyde 83 (or its congener 84) and amine 85 according to the format of Scheme 22. Clearly, sulfone 42 is an ideal carrier of synthon 82. Consequently, bond a would be formed in a Julia-like mode as seen earlier, whereas bond b could be efficiently created by means of a Fujita acylation62/desulfonylation sequence.
The foregoing logic led to the identification of acids 90 and 91 (Scheme 23) as the first major subgoals of the synthesis. The construction of these segments unfolded uneventfully and in high overall yield. The practical nature of this sequence is apparent: no expensive reagents, strong bases or careful control of inert atmospheres are necessary. Acids 90 and 91 were then subjected to Fujita acylation/desulfonylation.
The crucial C–C bond forming step (cf. 90 → 92) was found to afford superior results when a mixed anhydride was employed as the acylating agent in lieu of a more traditional acid chloride or acyl imidazole. The resultant ketosulfones 92 – 93 were best advanced to the following step in crude form, given their polar nature and C-acidity. Reductive desulfonylation to afford ketones 94 – 95 was achieved most efficiently with metallic Zn and aq. NH4Cl as described by Holton.63 The synthesis of siphonazole A was completed from 94 as illustrated in Scheme 24. Release of the benzyl ether and saponification of the ester afforded acid 96, which upon coupling with amine 8564 delivered 80 in high overall yield. The synthesis of siphonazole B proceeded in a like fashion from 95 (Scheme 25).65
We conclude this section by noting that synthetic siphonazoles obtained by the above routes were assayed for biological activity against various bacterial pathogens and tumor cell lines. What prompted these studies was the lack of information concering the biological properties of these natural products. At a test concentration of 10 µg/mL (NIH standard protocol; equivalent to 2.2•10–5 M for 80 and 2.1•10–5 M for 81), both siphonazoles were moderately cytotoxic to human breast carcinoma (HTB-129), and, especially, human acute T-cell leukemia (TIB-152) cells, but they were inactive against hepatocellular carcinoma (hepG2), prostate cancer (PC3), colon adenocarcinoma (Caco-2) and Chinese hamster ovarian cancer (CHO) cell lines at the same concentrations. Siphonazole A is more active than its congener 81. It induced cell death in human TIB-152 leukemia and mouse lymphoma cells with an IC50 value equal to 16 µg/mL (3.5•10–5 M), and in breast carcinoma NTB-129 with an IC50 of 20 µg/mL (4.2•10–5 M). Furthermore, it exhibited a good dose-response curve against human acute T cell leukemia TIB-152 and it was also cytotoxic against the mouse lymphoma cell line.44
CONCLUSION
The reactions described herein facilitate the construction of substituted oxazoles of interest in both natural products chemistry and drug research. We believe that in many cases the techniques described herein are superior to known alternatives for the synthesis of the target heterocycle. It is our hope that practitioners of synthetic heterocyclic chemistry in both industry and academia will make increasingly greater use of these methods.
ACKNOWLEDGMENT
We are grateful to the University of British Columbia, the Canada Research Chair program, NSERC, CIHR, and Merck Frosst Canada for support of our current research activities. Portions of this review focus on work that was carried out in France with support from Bayer CropScience, S. A., the CNRS, the MRT, and the Région Rhône-Alpes.
References
1. Isolation: A. Nagatsu, H. Kajitani, and J. Sakakibara, Tetrahedron Lett., 1995, 36, 4097; CrossRef Synthesis: P. Wipf and S. Venkatraman, J. Org. Chem., 1996, 61, 6517; CrossRef J. C. Muir, G. Pattenden, and R. M. Thomas, Synthesis, 1998, 613. CrossRef
2. Isolation: N. Lindquist, W. Fenical, D. Van Duyne, and J. Clardy, J. Am. Chem. Soc., 1991, 113, 2303; CrossRef R. Fernandez, M. J. Martin, R. Rodriguez-Acebes, F. Reyes, A. Francesch, and C. Cuevas, Tetrahedron Lett., 2008, 49, 2283; CrossRef Synthesis: J. Li, S. Jeong, L. Esser, and P. G. Harran, Angew. Chem. Int. Ed., 2001, 40, 4765; CrossRef J. Li, A. W. G. Burgett, L. Esser, C. Amezcua, and P. G. Harran, Angew. Chem. Int. Ed., 2001, 40, 4770; CrossRef K. C. Nicolaou, D. Y.-K. Chen, X. Huang, T. Ling, M. Bella, and S. A. Snyder, J. Am. Chem. Soc., 2004, 126, 12888; CrossRef K. C. Nicolaou, J. Hao, M. V. Reddy, P. B. Rao, G. Rassias, S. A. Snyder, X. Huang, D. Y.-K. Chen, W. E. Brenzovich, N. Giuseppone, P. Giannakakou, and A. O’Brate, J. Am. Chem. Soc., 2004, 126, 12897. CrossRef
3. Isolation: K. Shin-ya, K. Wierzba, K. Matsuo, T. Ohtani, Y. Yamada, K. Furihata, Y. Hayakawa, and H. Seto, J. Am. Chem. Soc., 2001, 123, 1262; CrossRef Synthesis: T. Doi, M. Yoshida, K. Shin-ya, and T. Takahashi, Org. Lett. 2006, 8, 4165; CrossRef Synthetic studies: N. Endoh, K. Tsuboi, R. Kim, Y. Yonezawa, and C.-g. Shin, Heterocycles, 2003, 60, 1567; CrossRef S. K. Chattopadhyay, S. Biswas, and S. K. Ghosh, Synthesis, 2008, 1029; CrossRef S. K. Chattopadhyay, S. Biswas, and B. K. Pal, Synthesis, 2006, 1289; CrossRef C. M. Marson and M. Saadi, Org. Biomol. Chem., 2006, 4, 3892; CrossRef S. K. Chattopadhyay and S. Biswas, Tetrahedron Lett., 2006, 47, 7897; CrossRef D. Hernandez, E. Riego, A. Francesch, C. Cuevas, F. Albericio, and M. Alvarez, Tetrahedron, 2007, 63, 9862; CrossRef J. Deeley, A. Bertram, and G. Pattenden, Org. Biomol. Chem., 2008, 6, 1994; CrossRef Medicinal chemistry: S. Yamada, K. Shigeno, K. Kitagawa, S. Okajima, and T. Asao, PCT Int. Appl. WO 2002048153 (2002); M. Tera, Y. Sohtome, H. Ishizuka, T. Doi, M. Takagi, K. Shin-ya, and K. Nagasawa, Heterocycles, 2006, 69, 505; CrossRef K. Shinke, K. Nagasawa, and T. Ono, Jpn. Kokai Tokkyo Koho JP 2006316008 (2006); Y. C. Tsai, H. Qi, C.-P. Lin, R.-K. Lin, J. E. Kerrigan, S. G. Rzuczek, E. J. LaVoie, J. E. Rice, D. S. Pilch, Y. L. Lyu, and L. F. Liu, J. Biol. Chem., 2009, 284, 22535. CrossRef
4. Recent example: A. Raveh, S. Moshe, Z. Evron, E. Flescher, and S. Carmeli, Tetrahedron, 2010, 66, 2705. CrossRef
5. T. G. Gant and S. Sarshar, U.S. Pat. Appl. Publ. (2010), US 2010120861; T. G. Gant and M. Shahbaz, PCT Int. Appl. (2010), WO 2010048358 (obesity, diabetes, hypertension); N. D. Smith, C. Bonnefous, M. Kahraman, S. A. Noble, J. E. Payne, and S. P. Govek, PCT Int. Appl. (2010), WO 2010048149 (cardiovascular/metabolic disease); J. J.-Q. Wu, PCT Int. Appl. (2010), WO 2010042925 (protein kinase C antagonists); P. Gjorstrup, PCT Int. Appl. (2010), WO 2010039529 (inflammatory disease); B. N. Cook, D. Disalvo, D. R. Fandrick, C. Harcken, D. Kuzmich, T. W.-H. Lee, P. Liu, J. Lord, C. Mao, J. Neu, B. C. Raudenbush, H. Razavi, J. T. Reeves, J. J. Song, A. D. Swinamer, and Z. Tan, PCT Int. Appl. (2010), WO 2010036632 (chemotactic cytokine receptor 1 antagonists); M. F. Brown, C. F. Donovan, E. L. Ellsworth, D. W. Hoyer, T. A. Johnson, M. S. Lall, C. Limberakis, S. T. Murphy, D. A. Sherry, C. B. Taylor, and J. S. Warmus, PCT Int. Appl. (2010), WO 2010032147 (antibacterial agents); J. Li, L. J. Kennedy, Y. Shi, S. Tao, X.-Y. Ye, S. Y. Chen, Y. Wang, A. S. Hernandez, W. Wang, P. V. Devasthale, S. Chen, Z. Lai, H. Zhang, S. Wu, R. A. Smirk, S. A. Bolton, D. E. Ryono, H. Zhang, N.-K. Lim, B.-C. Chen, K. T. Locke, K. M. O'Malley, L. Zhang, R. A. Srivastava, B. Miao, D. S. Meyers, H. Monshizadegan, D. Search, D. Grimm, R. Zhang, T. Harrity, L. K. Kunselman, M. Cap, P. Kadiyala, V. Hosagrahara, L. Zhang, C. Xu, Y.-X. Li, J. K. Muckelbauer, C. Chang, Y. An, S. R. Krystek, M. A. Blanar, R. Zahler, R. Mukherjee, P. T. W. Cheng, and J. A. Tino, J. Med. Chem., 2010, 53, 2854 (atherosclerosis). CrossRef
6. F. Eloy and A. Deryckere, Chim. Thér., 1973, 4, 437.
7. B. M. Nilsson and U. Hacksell, J. Heterocycl. Chem., 1989, 26, 269; CrossRef B. M. Nilsson, H. M. Vargas, B. Ringdahl, and U. Hacksell, J. Med. Chem., 1992, 35, 285. CrossRef
8. P. Wipf, L. T. Rahman, and S. R. Rector, J. Org. Chem., 1998, 63, 7132; CrossRef P. Wipf, Y. Aoyama, and T. E. Benedum, Org. Lett., 2004, 6, 3593. CrossRef
9. More recently, transition metal catalysis has been applied effectively to the cyclization of such propargylic amides: A. S. K. Hashmi, J. P. Weyrauch, W. Frey, and J. W. Bats, Org. Lett., 2004, 6, 4391; CrossRef M. D. Milton, Y. Inada, Y. Nishibayashi, S. Uemura, Chem. Commun., 2004, 2712; CrossRef A. S. K. Hashmi, M. Rudolph, S. Schymura, J. Visus, and W. Frey, Eur. J. Org. Chem., 2006, 4905; CrossRef J. E. Kang, H. B. Kim, J.-W. Lee, and S. Shin, Org. Lett., 2006, 8, 3537; CrossRef E. Merkul, O. Grotkopp, and T. J. J. Müller, Synthesis, 2009, 502. Once again, these methods furnish oxazoles that lack a 4-COOR substituent. CrossRef
10. Y. Nagao, K. Kweon, S. Sano, H. Kakegawa, W. S. Lee, H. Shimizu, M. Shiro, and N. Katunuma, Tetrahedron Lett., 1996, 37, 861; CrossRef S. Sano, H. Shimizu, K. Kim, W. S. Lee, M. Shiro, and Y. Nagao, Chem. Pharm. Bull., 2006, 54, 196. CrossRef
11. R. M. Williams, D. J. Aldous, and S. C. Aldous, J. Org. Chem., 1990, 55, 4657. CrossRef
12. P. Y. Coqueron, Dissertation, Université Claude Bernard Lyon 1 (France), 2002.
13. U. Zoller and D. Ben-Ishai, Tetrahedron, 1975, 31, 863; CrossRef D. Ben-Ishai, I Sataty, and Z. Bernstein, Tetrahedron, 1976, 32, 1571; CrossRef Z. Bernstein and D. Ben-Ishai, Tetrahedron, 1977, 33, 881. CrossRef
14. B. Metcalf and P. Casara, Tetrahedron Lett., 1978, 19, 1581. CrossRef
15. N. A. Petasis and I. Akritopoulou, Tetrahedron Lett., 1993, 34, 583; CrossRef N. A. Petasis and I. A. Zavialov, J. Am. Chem. Soc., 1997, 119, 7703. CrossRef
16. E. Negishi and S. Baba, J. Am. Chem. Soc., 1975, 97, 7385. CrossRef
17. A. Katritzky, D. C. Oniciu, and N. M. Yoon, J. Org. Chem., 1999, 64, 488. CrossRef
18. P. Y. Coqueron, C. Didier, and M. A. Ciufolini, Angew. Chem. Int. Ed., 2003, 42, 1411. CrossRef
19. repared by LiOH saponification of 24j-k and coupling of the resultant acid with (R)-α-methylbenzylamine in the presence of EDCI.
20. The COOR substituent activates the CH2 group at position 5 of the oxazole toward deprotonation: P. Cornwall, C. P. Dell, and D. W. Knight, Chem. Soc., Perkin Trans. 1, 1991, 2417. CrossRef
21. T. Sakamoto, H. Nagata, Y. Kondo, M. Shiraiwa, and H. Yamanaka, Chem. Pharm. Bull., 1987, 35, 823; S. Jeong, X. Chen, and P. G. Harran, J. Org. Chem., 1998, 63, 8640; CrossRef K. J. Hodgetts, and M. T. Kershaw, Org. Lett., 2002, 4, 2905; CrossRef K. J. Hodgetts and M. T. Kershaw, Org. Lett., 2003, 5, 2911; CrossRef J. E. M. Booker, A. Boto, G. H. Churchill, C. P. Green, M. Ling, G. Meek, J. Prabhakaran, D. Sinclair, A. J. Blake, and G. Pattenden, Org. Biomol. Chem., 2006, 4, 4193. CrossRef
22. T. Lechel, D. Lentz, and H.-U. Reissig, Chem. Eur. J., 2009, 15, 5432; CrossRef See also: A. S. K. Hashmi, J. P. Weyrauch, E. Kurpejovic, T. M. Frost, B. Michlich, W. Frey, and J. W. Bats, Chem. Eur. J., 2006, 12, 5806. CrossRef
23. G. Xu, K. Chen, and H. Zhou, Synthesis, 2009, 3565. CrossRef
24. T. J. Hoffmann, J. H. Rigby, S. Arseniyadis, and J. Cossy, J. Org. Chem., 2008, 73, 2400; CrossRef F. Besselièvre, S. Piguel, F. Mahuteau-Betzer, and D. S. Grierson, Org. Lett., 2008, 10, 4029; CrossRef C. Verrier, C. Hoarau, and F. Marsais, Org. Biomol. Chem., 2009, 7, 647, and literature cited therein. CrossRef
25. S. Chandrasekhar and A. Sudhakar, Org. Lett., 2010, 12, 236. CrossRef
26. In addition to refs. 8 and 21-25, see: (a) A. Maquestiau, R. Flammang, and F. B. Ben Abdelouahab, Heterocycles, 1989, 29, 103; CrossRef G. Emeric, S. Gary, V. Gerusz, N. Gourlaouen, B. Hartmann, N. Huser, H. Lachaise, L. Le Hir De Fallois, J. Perez, and T. Wegmann, T. PCT Int. Appl. WO 2001002385 (2001); E. Bacque, J. C. Barriere, and G. Puchault, Fr. Demande FR 2001-16856 20011226 (2003); H. U. Reissig and T. Lechel, Ger. Offen. DE 102008049431 (2010).
27. I. Bennett, N. P. J. Broom, G. Bruton, S. Calvert, B. P. Clarke, K. Coleman, R. Edmonson, P. Edwards, D. Jones, N. F. Osborne, and G. Walker, J. Antibiot., 1991, 44, 331.
28. F. Nan, J. Zuo, W. Wang, G. Wang, H. Chen, and P. He, PCT Int. Appl. WO 2006097030 (2006).
29. J. Zhang and M. A. Ciufolini, Tetrahedron Lett., 2010, 51, In Press. CrossRef
30. This is in accord with: E. E. Schweitzer and R. D. Bach, Org. Synth. Coll. Vol. V, 1145, and refs. cited therein.
31. J. Chau, J. Zhang, and M. A. Ciufolini, Tetrahedron Lett., 2009, 50, 6163. CrossRef
32. F. Sato, H. Uchiyama, K. Iida, Y. Kobayashi, and M. Sato, J. Chem. Soc., Chem. Commun., 1983, 921; CrossRef M. T. Reetz, R. Steinbach, J. Westermann, and R. Peter, Chem. Ber., 1985, 118, 1441. CrossRef
33. M. Koreeda and M. A. Ciufolini, J. Am. Chem. Soc., 1982, 104, 2308. CrossRef
34. R. G. Dickinson and H. Lotzkar, J. Am. Chem. Soc., 1937, 59, 472, and references cited therein. CrossRef
35. M. Julia and J. M. Paris, Tetrahedron Lett., 1973, 14, 4833; CrossRef Reviews: S. E. Kelly, in Comprehensive Organic Synthesis, B. M. Trost, I. Fleming, Eds; Pergamon Press: Oxford, UK, 1991, Vol. 1, pp. 729–817; P. R. Blakemore, J. Chem. Soc., Perkin Trans. 1, 2002, 2563. CrossRef
36. For a thorough discussion see ref. 44.
37. P. Wolbers, A. M. Misske, and H. M. R. Hoffmann, Tetrahedron Lett., 1999, 40, 4527; CrossRef P. Wolbers, M. Misske, and H. M. R. Hoffmann, Synlett, 1999, 1808. CrossRef
38. A. Tasaka, T. Taniguchi, S. Tsujimoto, and K. Naito, Jpn. Kokai Tokkyo Koho JP 2005035944 (2005).
39. C. J. Aquino, H. Dickson, and A. J. Peat, PCT Int. Appl. WO 2008157273 (2008).
40. P. Bernardelli, S. Keil, M. Urmann, H. Matter, W. Wendler, M. Glien, K. Chandross, J. Lee, C. Terrier, and H. Minoux, PCT Int. Appl. WO 2007039172 (2007).
41. H. Orino, T. Ishiyama, T. Tatematsu, C. Makino, Y. Ochiai, K. Kito, N. Kanetani, and K. Nishida, Jpn. Kokai Tokkyo Koho JP 2001278886 (2001).
42. Y. Momose, N. Takakura, T. Maekawa, H. Odaka, and H. Kimura, PCT Int. Appl. WO 2004024705 (2004).
43. T. Shih, S. L. Colletti, M. H. Fisher, P. T. Meinke, H. C. H. Kuo, P. K. Chakravarty, M. J. Wyvratt, S. Tyagarajan, and R. Berger, U.S. Patent 6586452 (2003).
44. J. Zhang, E. E. Polishchuk, J. Chen, and M. A. Ciufolini, J. Org. Chem., 2009, 74, 9140. CrossRef
45. As reflected by their respective A-values: 2.9 kcal/mol for a PhSO2 group (E. Juaristi, V. Labastida, and S. Antunez, J. Org. Chem., 2000, 65, 969); CrossRef 2.8 kcal/mol for a Ph group (E. L. Eliel and S. Wilen, Stereochemistry of Organic Compounds; Wiley: New York, NY, 1994, p. 697); approximately 1.3 kcal/mol for a 4-carbomethoxy-5-methyl-2-oxazolyl group [estimated (Hyperchem® package) by MM+ structural optimization of the axial and equatorial conformer of the corresponding 2-cyclohexyl derivative].
46. An excellent discussion of vinylsulfone – allylsulfone isomerization appears in: A. El-Awa, M. N. Noshi, X. Mollat du Jourdin, and P. L. Fuchs, Chem. Rev., 2009, 109, 2315, and literature cited therein. CrossRef
47. We presume that the major product was the E-isomer, by analogy with the previous cases.
48. Reviews on desulfonylation: N. Simpkins, Tetrahedron, 1990, 46, 6951; CrossRef C. Najera and M. Yus, Tetrahedron, 1999, 55, 10547. CrossRef
49. J. A. Deyrup and K. K. Killion, J. Heterocycl. Chem., 1972, 9, 1045. CrossRef
50. X. Sun, J. Janvier, H. Bienaymé, and J. Zhu, Org. Lett., 2001, 3, 877; CrossRef P. Janvier, X. Sun, H. Bienaymé, and J. Zhu, J. Am. Chem. Soc., 2002, 124, 2560; CrossRef P. Janvier, M. Bois-Choussy, H. Bienaymé, and J. Zhu, Angew. Chem. Int. Ed., 2003, 42, 811; CrossRef S.-X. Wang, M.-X. Wang, D.-X. Wang, and J. Zhu, Org. Lett., 2007, 9, 3615; CrossRef T. Yue, M. X. Wang, D X. Wang, G. Masson, and J. Zhu, Angew. Chem. Int. Ed., 2009, 48, 6717; CrossRef T. Yue, M. X. Wang, D. X. Wang, G. Masson, and J. Zhu, J. Org. Chem., 2009, 74, 8396; CrossRef Related reactions: T. Pirali, G. C. Tron, and J. Zhu, Org. Lett., 2006, 8, 4145; CrossRef D. Bonne, M. Dekhane, and J. Zhu, Angew. Chem. Int. Ed., 2007, 46, 2485; CrossRef J. Wu, W. Chen, M. Hu, H. Zou, and Y. Yu, Org. Lett., 2010, 11, 616; CrossRef M. Daniel, R. K. Rizvi, and J. J. Kaminski, Heterocycles, 1987, 26, 651. CrossRef
51. Routes to 5-aminooxazoles that do not rely upon isonitrile building blocks may proceed via the cyclization of α-amidonitriles [A. H. Cook and G. D. Hunter, J. Chem. Soc., 1952, 3789; CrossRef S. Mandal, W. T. Li, Y. Bai, O. V. Shablykin, V. S. Brovarets, and B. S. Drach, Russ. J. Gen. Chem., 2007, 77, 1308; CrossRef M. C. Carreiras, A. Eleuterio, C. Dias, M. A. Brito, D. Brites, J. Marco-Contelles, and E. Gomez-Sanchez, Heterocycles, 2007, 71, 2249; CrossRef J. D. Robertus and S. M. Kerwin, Beilst. J. Org. Chem., 2008, 4, http://www.beilstein-journals.org/bjoc/content/provisional/1860-5397-4-26.pdf] and related reactions; [A. V. Golovchenko, S. G. Pilyo, V. S. Brovarets, A. N. Chernega, and B. S. Drach, Russ. J. Gen. Chem., 2005, 75, 425; CrossRef A. V. Golovchenko, S. G. Pilyo, V. S. Brovarets, A. N. Chernega, and B. S. Drach, Synthesis, 2003, 2851; CrossRef B. S. Drach, G. N. Mis'kevich, and A. P. Martynyuk, Zh. Org. Khim., 1978, 14, 508]; the cyclodehydration of α-amido-amides [M. Falorni, G. Giacomelli, A. Porcheddu, and G. Dettori, Eur. J. Org. Chem., 2000, 3217; CrossRef G. I. Elliott, J. R. Fuchs, B. S. J. Blagg, H. Ishikawa, H. Tao, Z.-Q. Yuan, and D. L. Boger, J. Am. Chem. Soc., 2006, 128, 10589]; CrossRef as well as Cornforth rearrangements [I. J. Turchi and C. A. Maryanoff, Synthesis, 1983, 837; CrossRef M. B. Nolt, M. A. Smiley, S. L. Varga, R. T. McClain, S. E. Wolkenberg, and C. W. Lindsley, Tetrahedron, 2006, 62, 4698]. CrossRef
52. Unpublished results from this laboratory (J. Zhang, P.-Y. Coqueron, J.-P. Vors, and M. A. Ciufolini, Org. Lett., 2010, 12, ASAP).
53. The robot could also generate the chloroglycinates via the reaction of an amide with a glyoxylic ester, followed by treatment of the adduct with SOCl2. However, safety and corrosion issues associated with the use of SOCl2 dissuaded us from automating these reactions.
54. W. Nagata and M. Yoshioka, Tetrahedron Lett., 1966, 18, 1913; CrossRef W. Nagata and M. Yoshioka, Org. React., 1984, 25, 255.
55. R. A. Smith, J. Barbosa, C. L. Blum, M. A. Bobko, Y. V. Caringal, R. Dally, J. S. Johnson, M. E. Katz, N. Kennure, J. Kingery-Wood, W. Lee, T. B. Lowinger, J. Lyons, V. Marsh, D. H. Rogers, S. Swartz, T. Walling, and H. Wild, Bioorg. Med. Chem. Lett., 2001, 11, 2775. CrossRef
56. N. Xi and M. A. Ciufolini, Tetrahedron Lett., 1995, 36, 6595. CrossRef
57. Unpublished results from this laboratory (Dr. M. El-Qacemi).
58. Q. Dong, C. E. Anderson, and M. A. Ciufolini, Tetrahedron Lett., 1995, 36, 5681. CrossRef
59. Isolation: M. Nett, Ö. Erol, S. Kehrhaus, M. Köck, A. Krick, E. Eguereva, E. Neu, and G. M. König, Angew. Chem. Int. Ed., 2006, 45, 3863; CrossRef Synthesis: J. Linder and C. J. Moody, Chem. Commun., 2007, 1508; CrossRef J. Linder, A. J. Blake, and C. J. Moody, Org. Biomol. Chem., 2008, 6, 3908. CrossRef
60. Bis-oxazoles of this type besides muscoride A (ref. 1) and telomestatin (ref. 3) include, e.g., hennoxazole (isolation: T. Ichiba, W. Y. Yoshida, P. J. Scheuer, T. Higa, and D. G. Gravalos, J. Am. Chem. Soc., 1991, 113, 3173; CrossRef T. Higa, J. Tanaka, A. Kitamura, T. Koyama, M. Takahashi, and T. Uchida, Pure Appl. Chem., 1994, 66, 2227; CrossRef Synthesis: P. Wipf and S. Lim, J. Am. Chem. Soc., 1995, 117, 558; CrossRef P. Wipf and S. Lim, Chimia, 1996, 50, 157; D. R. Williams, D. A. Brooks, and M. A. Berliner, J. Am. Chem. Soc., 1999, 121, 4924; CrossRef F. Yokokawa, T. Asano, and T. Shioiri, Tetrahedron, 2001, 57, 6311; CrossRef Synthetic studies: A. G. M. Barrett and J. T. Kohrt, Synlett, 1995, 415); CrossRef For higher polyoxazoles of this type see the following leading references: D. S. Dalisay, E. W. Rogers, A. S. Edison, and T. F. Molinski, J. Nat. Prod., 2009, 72, 732; CrossRef J. Deeley, A. Bertram, and G. Pattenden, Org. Biomol. Chem., 2008, 6, 1994; CrossRef D. Hernandez, G. Vilar, E. Riego, L. M. Canedo, C. Cuevas, F. Albericio, and M. Alvarez, Org. Lett., 2007, 9, 809; CrossRef C. Petchprayoon, Y. Asato, T. Higa, L. F. Garcia-Fernandez, S. Pedpradab, G. Marriott, K. Suwanborirux, and J. Tanaka, Heterocycles, 2006, 69, 447; CrossRef Z. Jin, Nat. Prod. Rep., 2006, 23, 464; CrossRef V. S. C. Yeh, Tetrahedron, 2004, 60, 11995; CrossRef S. Matsunaga, N. Fusetani, and K. Hashimoto, J. Am. Chem. Soc., 1986, 108, 84760. CrossRef
61. This motif is found in the bengazoles, a group of about 20 marine alkaloids with remarkable bioactivity. Isolation: M. Adamczeski, E. Quinoa, and P. Crews, J. Am. Chem. Soc., 1988, 110, 1598; CrossRef J. Rodriguez, R. M. Nieto, and P. Crews, J. Nat. Prod., 1993, 56, 2034; CrossRef A. Rudi, Y. Kashman, Y. Benayahu, and M. Schleyer, J. Nat. Prod., 1994, 57, 829; CrossRef A. Groweiss, J. J. Newcomer, B. R. O'Keefe, A. Blackman, and M. R. Boyd, J. Nat. Prod., 1999, 62, 1691; CrossRef R. Fernandez, M. Dherbomez, Y. Letourneux, M. Nabil, J. F. Verbist, and J. F. Biard, J. Nat. Prod., 1999, 62, 678; CrossRef P. A. Searle, R. K. Richter, and T. F. Molinski, J. Org. Chem., 1996, 61, 4073; CrossRef Total syntheses: R. J. Mulder, C. M. Shafer, T. F. Molinski, J. Org. Chem., 1999, 64, 4995; CrossRef J. A. Bull, E. P. Balskus, R. A. J. Horan, M. Langner, and S. V. Ley, Angew. Chem. Int. Ed., 2006, 45, 6714; CrossRef J. A. Bull, E. P. Balskus, R. A. J. Horan, M. Langner, and S. V. Ley, Chem. Eur. J., 2007, 13, 5515; CrossRef Synthetic studies: R. J. Mulder, C. M. Shafer, and T. F. Molinski, J. Org. Chem., 1999, 64, 4995; CrossRef C. Shafer and T. F. Molinski, Tetrahedron Lett., 1998, 39, 2903; CrossRef C. M. Shafer and T. F. Molinski, Heterocycles, 2000, 53, 1167; CrossRef J. C. Hodges, W. C. Patt, and C. J. Connolly, J. Org. Chem., 1991, 56, 449; CrossRef E. Vedejs, and S. D. Monahan, J. Org. Chem., 1996, 61, 5192; CrossRef P. Chittari, Y. Hamada, and T. Shioiri, Synlett, 1998, 1022; CrossRef P. Chittari, Y. Hamada, and T. Shioiri, Heterocycles, 2003, 59, 465. CrossRef
62. Y. Nagao, S. Yamada, and E. Fujita, Tetrahedron Lett., 1983, 24, 2287; CrossRef Y. Nagao, S. Yamada, and E. Fujita, Tetrahedron Lett., 1983, 24, 2291. CrossRef
63. R. A. Holton, D. J. Crouse, A. D. Williams, and R. M. Kennedy, J. Org. Chem., 1987, 52, 2317. CrossRef
64. P. A. Grieco, P. Galatsis, and R. F. Spohn, Tetrahedron, 1986, 42, 2847. CrossRef
65. See ref. 44 as well as J. Zhang and M. A. Ciufolini, Org. Lett., 2009, 11, 2389 CrossRef