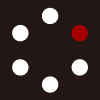
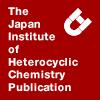
HETEROCYCLES
An International Journal for Reviews and Communications in Heterocyclic ChemistryWeb Edition ISSN: 1881-0942
Published online by The Japan Institute of Heterocyclic Chemistry
e-Journal
Full Text HTML
Received, 2nd July, 2010, Accepted, 12th August, 2010, Published online, 13th August, 2010.
DOI: 10.3987/COM-10-S(E)93
■ A Cyclic Acetal Tethered Intramolecular Diels-Alder Cycloaddition. Studies Directed toward a Total Synthesis of (±)-Fusidilactone C
Sunil K. Ghosh, Yonggang Wei, Aleksey I. Gerasyuto, John B. Feltenberger, Jiashi Wang,* and Richard P. Hsung*
Department of Chemistry, Division of Pharmaceutical Sciences, University of Wisconsin, 777 Highland Aenue, Madison, WI 53705-2222, U.S.A.
Abstract
Efforts toward a synthesis of (±)-fusidilactone C is described here featuring a novel cyclic acetal tethered intramolecular Diels-Alder strategy. This unique and facile IMDA turned out to be highly endo-selective [endo-I and endo-II], as assessed from our mechanistic analyses. When using protic solvents or Lewis acids, the endo-I selectivity was greatly enhanced. Thus, it proved to be a real challenge to circumvent this excellent stereochemical outcome, which is undesired for the total synthesis, as an exo-II selectivity is desired. Progress was made to use the endo-II cycloadduct and to access the desired trans-2-oxadecalin motif in (±)-fusidilactone C.INTRODUCTION
(+)-Fusidilactone C [3], a highly-oxygenated polycyclic lactone, along with simpler family members fusidilactones A and B [1 and 2 in Scheme 1], were isolated from the culture broth of fusidium sp.,1 a fungal endophyte found in the leaves of Mentha arvensis in Germany. Organic extracts from this culture broth reveal potent antifungal activities against Eurotium repens and Fusarium oxysporum, and they also possess moderate antimicrobial activities against E. coli, Bacillus megaterium and Chlorella fusca.1 While fusidilactones A and B contain six-membered oxygen heterocycle fused to a γ-lactone with the only difference being the oxidation state at the ring junction and side chain, what drew our attention was the unique structural motif of fusidilactone C, which poses a tremendous amount of challenge.
Foremost, (+)-fusidilactone C [3] features a rare oxo-adamantane skeleton comprised of an unusual ether-bridged bis-hemiacetal motif.2 The only other known natural product containing an oxo-adamantane motif is 5,15-oxidolycopodane,3 although tetrodotoxin possesses a dioxo-admantane core.4,5 In addition, 3 embodies a bridged γ-lactone F-ring, a highly substituted cyclohexane C-ring, a trans-fused 2-oxadecalin as the BC-ring, and a spiroketal as the AB-ring but with severe 1,3-diaxial interaction from axial substituents at C4a and C6. Last, but not the least, (+)-fusidilactone C [3] also has 11 stereogenic centers with configuration at C2” remaining unassigned. To date, besides Snider’s synthesis of B [see 2],6 and our efforts toward 3,7 there has not been any other reports in this family of natural products. We report here our progress toward a total synthesis of (±)-fusidilactone C.
RESULTS AND DISCUSSION
1. Retrosynthetic Analysis
Retrosynthetically, the oxo-bridge of the bis-hemiacetal in fusidilactone C [3] could be derived from hydration of C9,11-diketone 4, which could be envisioned from tetracyclic lactone 5 via a Dieckmann condensation [X = OMe] or an aldol reaction [X = H] to re-connect C3- and C11 [Scheme 2]. These analyses allowed us to simplify the C11-C1” β-diketo motif in 5 to the protected C11-C1” diol 6 because either C11 or C1” could serve as the precursor for Dieckmann or aldol, and the C10-stereochemistry can be regulated through means of epimerization.
The lactone F-ring in 6 could be constructed in two different ways: Through an esterification route connecting O1-C2, or via an iodolactonization of C9a-C9 olefin using acid 7 to connect O1-C9a, which is shown here as our first option. Acid 7 is just an oxidation state away from tricycle 8 at C5-OH, and tricycle 8, containing the 2-oxadecalinic BC ring and the spiroketal AB-ring of fusidilactone C [3], would represent a key advanced intermediate that we would like to target.
Synthesis of the advanced tricycle 8 would then feature a cyclic acetal tethered intramolecular Diels-Alder cycloaddition [IMDA] preferably in an exo-manner using cyclic acetal 9, leading to an efficient approach for completing the formation of not only both B and C rings of the 2-oxadecalinic motif but also the AB-spiroketal moiety. This cyclic acetal tethered IMDA represents a novel strategy developed in our lab. Cyclic acetal 9 could be accessed from E-E-diene 10 and dihydrofuran 11 through simple Brønsted acid promoted acetal formation.
2. The Cyclic Acetal Tethered Intramolecular Diels-Alder Cycloaddition
Cyclic acetal-tethered intramolecular Diels-Alder cycloaddition reaction [IMDA]8 should represent a powerful strategy for constructing polycyclic spiroketals. There can be four possibilities as shown in Figure 1: Type a is the most commonly seen with both reactive partners A and B [dienes and a dienophiles] being connected at the acetal carbon C2. However, in these cases, it is clear that the acetal predominately serves as a carbonyl-protecting group. Another common scenario is Type b, and the distinction here is not as clear whether the acetal is used to protect the diol or to link together A and B, although most belong the former case. Some interesting examples are shown here for Type b. The best examples of Type b are from Craig's9 IMDA using acetal 12.
Thirdly, Type c, although uncommon, represents a more accurate concept of acetal tethered intramolecular reactions. There are only a few precedents for IMDA in this category. Notable examples of Type c would be Boeckman's acetal tethered IMDA using 13,10 Roush's dioxolanone 14,11 and Jung's12 [15] aminal tether. Chemistry of ortho- [16] or para-quinone ketals [not shown] is quite well known, but these intermediates are usually generated in situ.13 Finally, Type d can be classified as cyclic acetal tethered systems, and IMDA reactions between A and B would lead to the construction of spiroketals. Examples in this classification are virtually unknown until our work.
The lack of interest in using acetals as tethers could be due to their overwhelming reputation as protecting groups for carbonyls and alcohols and their perceived instability under hydrolytic conditions. However, we recognized that developing cyclic acetal tethered IMDA in Type d would allow us to examine the potential influence of the anomeric effect on the conformational preference for 17-ax over 17-eq [Figure 1] [~ 1.5 kcal mol-1 per anomeric effect],14,15 which could impact on both reactivity and the stereochemical outcome of an IMDA.7,16,17 In addition, pursuing Type d-IMDA should prove to be significant in bringing forth a fundamentally different approach to constructing spiroketals 18 or related natural products.18-20
3. Possible Stereochemical Outcome of the Cyclic Acetal Tethered IMDA
Given the novelty of this cyclic acetal tethered IMDA strategy, we first analyzed all possible transition states for the cycloaddition. As shown in Figure 2, there can be 4 possible endo-transition states: TS-19n1 [TS-endo-I: Boat-Boat conformation], TS-19n2 [TS-endo-II: Chair-Boat conformation], TS-20n1 [TS-endo-I: Chair-Boat conformation], and TS-20n2 [TS-endo-II: Chair-Boat conformation]. The major difference between TS-19 and TS-20 is the position of the furanyl oxygen atom with respect to the formation of pending 3-pyranone [or the actual B-ring]: Pseudo-axial [ax] in TS-19 and pseudo-equatorial [eq] TS-20. Based on an anomeric effect worthy of ~1.5 kcal mol-1,18 and our related work demonstrating a profound anomeric control of transition state conformation,16 the furanyl oxygen would prefer a pseudo-axial position, leading to TS-19 being preferred.
Likewise, we focused on analyzing the two exo-transition states, TS-19x1 [TS-exo-I: Boat-Boat] and TS-19x2 [TS-exo-II: Chair-Boat], in which furanyl oxygen is pseudo-axial. Calculations [SpartanTM: G-31G*/B3LYP] distinctly revealed that both endo-transition states TS-19n1 and TS-19n2 [for R = CH(CH2OBn)2] are favored over exo-transition states TS-19x1 and TS-19x2 by ~1.40-1.57 kcal mol-1, while the difference between TS-19n1 and TS-19n2 [and that of TS-19x1 and TS-19x2] is very small [~ 0.11 kcal mol-1] in favored TS-19n1. These mechanistic assessments were not overtly surprising because one still expects the endo-rule to be comparably significant in IMDA reactions as in intermolecular ones, although exo-transition state is well known to play a prominent role in IMDAs. In addition, it is conceivable that both TS-19n1 and TS-19n2 have an advantage over TS-19x1 or TS-19x2 because of the steric interaction between the diene and the R group [on the dienophile] in the exo-transition state. However, we had gauged TS-19n1 to be less favorable than TS-19n2 due to the unfavorable dipole between the carbonyl group and C-O bond in the furan ring, and that TS-19n2 pertains a Chair-Boat reactive conformation. On the other hand, it is conceivable that the repulsion between the lone-pair electrons on the pending 3-pyanone oxygen atom and the carbonyl oxygen atom, as well as 1,3-diaxial interaction between the R group and the furan could lead a significant destabilization of TS-19n2.
From these analyses, the most desired pathway TS-19x2 appeared to be far reaching, but that did not deter our efforts toward a total synthesis of fusidilactone C. While the TS-19x1 pathway would lead to a real dead end in terms of a total synthesis of fusidilactone C, as all three key stereocenters in the cycloadduct 21-exo-I are incorrect, IMDA cycloadducts 21-endo-I and 21-endo-II attained through TS-19n1 or TS-19n2, respectively, could still represent viable entries to the trans-2-oxadecalin spiroketal of fusidilactone C [3] via appropriate stereochemical adjustments. However, given the preconceived notion that the steric interaction between the diene motif and the R group [CH(CH2OBn)2] in the dienophiles is destabilizing the two exo transitions states, we first pursued the following model studies using substrates in which R = H or Me.
4. Model Studies on the Cyclic Acetal Tethered IMDA
(E,E)-Diene 25 that will be employed in model studies as well as in the total synthesis could be readily prepared in 5 steps from known aldehyde 22 in good overall yield and high level stereochemical control with regards to the olefin geometry [Scheme 3]. For the first model study, ketone 28 was synthesized from dihydrofuran [DHF] in 34% overall yield in 6 steps, featuring addition of lithiated dihydrofuran [Li-DHF] to acetaldehyde and cyclic acetal formation using PPTS and diene 25 that gave the key cyclic acetal 27 anchored with the diene motif. The minor sidetrack here is that we had to remove both TBS protecting groups and re-protect the resulting primary alcohol.
Befitting with the theme of this special issue of Heterocycles dedicated to Professor Albert Eschenmoser, the ensuing enone formation from ketone 28 involved the addition of Eschenmoser's salt21 to the lithium enolate generated from 28 and subsequent β-elimination in MeOH at rt. The use of Eschenmoser's salt is a key to constructing all the cycloaddition precursors here. Intriguing, the cyclic acetal tethered intramolecular Diels-Alder cycloaddition had already occurred under the alpha-olefination conditions to give 30-endo-I cycloadduct as a single diastereomer in 28% overall yield from 28. Enone 29 was never isolated, and while not characterized, an equal amount of the side product resulting from the addition of MeOH to 29 was found. It is noteworthy that this novel cyclic acetal tethered IMDA is unusually fast, and such rate enhancement is likely associated with protic solvent.22,23
We then quickly assembled ketone 32 in an analogous manner but without the confusion of TBS protecting groups by using the acetyl capped LiDHF addition product 26’ [Scheme 4]. The exact same scenario unfolded when ketone 32 was subjected to alpha-methylenation using Eschenmoser’s salt: We could not isolate enone 33, but we obtained the IMDA cycloadduct 34-endo-I as a single isomer in much higher overall yield. Desilylation of 34-endo-I followed by p-bromobenzoyl ester formation gave 36, which afforded a single crystal X-ray structure [Figure 3] that allowed us to unambiguously assign the endo-I stereochemical outcome. This assessment lent support to the favorable TS-20 pathway in our mechanistic analysis, and its manifestation in protic solvent, as proton from MeOH can chelate to both carbonyl and furanyl oxygens.
To demonstrate that we could at least access the trans-2-oxadecalin spiroketal motif required in fusidilactone C [3], epimerization of C4a in 30-endo-I was carried out using K2CO3 and MeOH at rt [Scheme 5]. Tricycle 30-exo-II was cleanly attained in 82% yield, thereby suggesting that while the respective TS-exo-II pathway [see TS-19x2 in Figure 2] may not be a favorable one, the exo-II cycloadduct containing the trans-2-oxadecalin spiroketal is thermodynamically more stable than that of endo cycloadducts. The relative stereochemistry 30-exo-II was assigned using NOE experiments as well as coupling constants.
5. Progress Toward Fusidilactone C
Continuing to explore this novel IMDA and identify possible pathways en route to the endo-II or even exo cycloadducts, the key cycloaddition precursor enone 42 was found to be isolable. As shown in Scheme 6, the synthesis of enone 42 was achieved in 7 steps commencing from alkene 37. It is noteworthy that we were able to isolate the cycloaddition precursor for the first time, suggesting a much slower cycloaddition course for enone 42 due to the bulky R group.
Isolation of the cycloaddition precursor 42 allowed us to examine this reaction in greater detail. It was quickly found that although the reaction proceeded much slower in toluene even at 110 oC [or in undecane at 200 oC], an improved formation of endo-II product was seen with the ratio of 43-endo-I to 43-endo-II being 1.0 to 1.4:1 [Scheme 7]. The cycloaddition was again found not only fast in MeOH but also afforded a 12:1 mixture in favor of 43-endo-I with an overall yield of 90%. The use of various Lewis acids [i.e., ZnCl2, Zn(OTf)2, Cu(OTf)2, MgBr2-Et2O, AlEt2Cl, Sc(OTf)3, Yb(OTF)3, SnCl2, LiClO4, Ti(O-i-Pr)4, and RhCl(Ph3P)3/AgOTf or AgBF4] in CH2Cl2 led to exclusive formation of 43-endo-I in a facile and high yielding manner at low temperature [-78 oC], thereby suggesting a metal chelation that can further favor TS-endo-I in addition to rate enhancement. Only c-hex2BCl gave 43-endo-I to 43-endo-II in 1:1 ratio.
These findings are consistent with results attained from MeOH and further underscore the significance of TS-endo-I in this unique cyclic acetal tethered IMDA. It is also noteworthy that the cyclic acetal tethering is relatively robust under these Lewis acidic conditions.
To continue our total synthesis efforts, we explored the feasibility of converting cycloadduct 43-endo-II to the desired exo-II cycloadduct through the epimerization strategy [Scheme 8]. Epimerization are required at both C8a and C3a, and we elected to pursue first at C8a. A clean separation 43-endo-I and 43-endo-II required first desilylation, which gave the isomeric mixture of free alcohol 44. While re-silylation using TBSCl gave 43-endo-I and 43-endo-II, TES-protection of 44-endo-II afforded 43’’-endo-II, which was found to be an overall better substrate than 43-endo-II in a later oxidation step.
Dihydroxylation of 43’’-endo-II employing the stoichiometric amount of OsO4 produced the desired 1,2-diol 45” as single diastereomer in excellent yield. The high stereoselectivity probably was the consequence of formation of the osmate from the convex face of the cis-oxadecalin. Desilylation of either 45 or 45” led to 45-OH, which is a crystalline material for attaining an X-ray structure as shown in Figure 4, which unambiguously assigns the relative stereochemical manifold in the endo-II cycloadduct. It is noteworthy that compound 45 or 45” would contain all the carbons in the framework of (±)-fusidilactone C. The subsequent Parikh-Doering oxidation of the secondary alcohol in 45” in the presence of the adjacent tertiary hydroxyl group provided the desired hydroxy ketone 46” in 50% yield. Nevertheless, the oxidation yields for 46” are consistently better than that of 46.
Attempts to epimerize the tertiary stereocenter α to the carbonyl by exposing hydroxy ketone 46” to either K2CO3 in MeOH, DBU in CH2Cl2 at -78 oC – 0 oC [or in toluene at 110 oC], LHMDS in THF at at -78 oC failed to provide any desired trans-2-oxadecalin 47”, which indicated the labile nature of the β-alkoxy ketone under basic condition via β-elimination. However, at -78 oC in THF, the use of LDA, followed by quenching with MeOH [or D2O], led to 1:1 mixture of 46” and 47” in 45-60% yield, thereby implying the possibility of epimerizing the C8a position, although 47” was never separable from 46”, and thus, it could not be vigorously characterized.
CONCLUSION
We have reported here our efforts toward a synthesis fusidilactone C that would feature a novel cyclic acetal tethered intramolecular Diels-Alder strategy. This unique and facile intramolecular Diels-Alder cycloaddition turned out to be highly endo-selective, as assessed from our mechanistic analyses. When using protic solvents or Lewis acids, this endo-selectivity was even more pronounced. It proved to be a real challenge to circumvent this selectivity, although progress was made to attain endo-II cycloadduct and to access the desired trans-2-oxadecalin motif through epimerization at C8a.
EXPERIMENTAL
All reactions were performed in flame-dried glassware under a nitrogen atmosphere. Solvents were distilled prior to use. Reagents were used as purchased (Aldrich, Acros), except where noted. Chromatographic separations were performed using Bodman 60 Å SiO2. 1H and 13C NMR spectra were obtained on Varian VI-300, VI-400, and VI-500 spectrometers using CDCl3 (except where noted) with TMS or residual CHCl3 in the solvent as standard. Melting points were determined using a Laboratory Devices MEL-TEMP and are uncorrected/calibrated. Infrared spectra were obtained using NaCl plates on a Bruker Equinox 55/S FT–IR Spectrophotometer, and relative intensities are expressed qualitatively as s (strong), m (medium), and w (weak). TLC analysis was performed using Aldrich 254 nm polyester-backed plates (60 Å, 250 µm) and visualized using UV and a suitable chemical stain. Low-resolution mass spectra were obtained using an Agilent-1100-HPLC/MSD and can be either APCI or ESI, or an IonSpec HiRes-MALDI FT-Mass Spectrometer. High-resolution mass spectral analyses were performed at University of Wisconsin Mass Spectrometry Laboratories. All spectral data obtained for new compounds are reported. X-Ray analyses were performed at the X-Ray facility in University of Minnesota.
Preparation of E,E-Diene 25.
Wittig Olefination. To a solution of the known aldehyde 22 (55.2 g, 293.1 mmol) in toluene (500 mL) was added (1-ethoxycarbonylethylidene)triphenylphosphorane (112.3 g, 322.4 mmol, 1.1 equiv) in several portions at rt. The resulting mixture was refluxed for 12 h, then cooled down to rt and concentrated in vacuo. To the crude product were added hexanes and the precipitated triphenylphosphine oxide was filtered. The filtrate was concentrated in vacuo to afford the crude mixture that was purified using gradient silica gel flash column chromatography (2-15% EtOAc in hexanes) to provide the desired unsaturated ester (70.0 g, 256.9 mmol) as an inseparable 20:1 (E:Z) mixture in 88% yield. The unsaturated ester intermediate: Rf = 0.72 (20% EtOAc in hexanes); 1H NMR (500MHz, CDCl3) δ 6.78 (tq, 1H, J = 1.5, 7.5 Hz), 4.19 (q, 2H, J = 7.5 Hz), 3.71 (t, 2H, J = 7.0 Hz), 2.41 (dtq, 2H, J = 1.0, 7.0, 7.0 Hz), 1.85 (dt, 3H, J = 1.0, 1.0 Hz), 1.29 (t, 3H, J = 7.5 Hz), 0.90 (s, 9H), 0.06 (s, 6H).
Dibal-H Reduction. To a solution of the aforementioned 20:1 (E:Z) mixture of unsaturated ester (34.5 g, 126.6 mmol) in anhyd CH2Cl2 (200 mL) was added slowly DIBAL-H (278.6 mL, 1.0 M solution in hexanes, 278.6 mmol, 2.2 equiv) at -78 oC. The reaction was stirred at -78 oC for 2 h and the TLC analysis indicated the complete conversion from the starting material to the product.
The reaction was quenched with MeOH (50 mL) at -78 oC carefully, and then, to the resulting mixture was added sat aq sodium potassium tartrate (200 mL) at -78 oC slowly. The resulting mixture was allowed to warm up to rt and stirred until the organic layer and the aqueous layer were completely separated. The reaction mixture was extracted with ether (3 x 100 mL) and the combined organic layers were washed with sat aq NaCl (200 mL), dried over Na2SO4, and concentrated in vacuo. The crude allylic alcohol was purified using careful gradient silica gel flash column chromatography (2-20% EtOAc in hexanes) to provide the desired E-allylic alcohol 23 (25.1 g, 108.9 mmol) as colorless oil in 86% yield. Alcohol 23: Rf = 0.34 (40% EtOAc in hexanes); 1H NMR (500MHz, CDCl3) δ 5.43 (qt, 1H, J = 1.5, 7.5 Hz), 4.02 (d, 2H, J = 5.5 Hz), 3.62 (t, 2H, J = 7.0 Hz), 2.28 (dq, 2H, J = 1.0, 7.0 Hz), 1.69 (s, 3H), 1.31 (dt, -OH, 1H, J = 1.5, 6.0 Hz) [long range coupling with one of the allylic methylene protons at 2.28 ppm], 0.90 (s, 9H), 0.06 (s, 6H); 13C NMR (125MHz, CDCl3) δ 136.7, 122.0, 68.7, 62.8, 31.4, 25.9, 18.4, 13.8, -5.3; IR (neat) cm-1 3352brs, 2954s, 2928s, 2857s, 1472s, 1387m, 1256s, 1090s, 1007s, 938ms, 836s, 776s; mass spectrum (GC-MS): m/e (% relative intensity) 173 (10) (M+-t-Bu), 155 (5), 105 (100), 89 (19), 75 (88), 57 (5).
Parikh-Doering Oxidation. To a solution of the above pure E-allylic alcohol 23 (21.6 g, 93.8 mmol) in anhyd CH2Cl2 (200 mL) were added anhyd DMSO (72.5 mL, 1.02 mol, 10.9 equiv) and anhyd Et3N (64.8 mL, 464.9 mmol, 5.0 equiv) sequentially at rt. The reaction mixture was cooled down to 0 oC before SO3.Py complex (29.8 g, 187.3 mmol, 2.0 equiv) was added in several portions. The reaction was stirred at 0 oC for 1 h, allowed to warm up to rt, and stirred for an additional 1h. TLC analysis indicated the disappearance of the starting material and the reaction was quenched with water (100 mL) and extracted with Et2O (2 x 100 mL). The combined organic layers were washed with water (2 x 100 mL) and sat aq NaCl (150 mL), dried over Na2SO4, and concentrated in vacuo. The crude α,β-unsaturated aldehyde was purified using gradient silica gel flash column chromatography (5-20% EtOAc in hexanes) to afford the desired E-enal 24 (20.4 g, 89.3 mmol) as colorless oil in 95% yield. E-Enal 24: Rf = 0.70 (20% EtOAc in hexanes); 1H NMR (500MHz, CDCl3) δ 9.42 (s, 1H), 6.56 (qt, 1H, J = 1.5, 7.0 Hz), 3.78 (t, 2H, J = 6.0 Hz), 2.58 (dt, 2H, J = 7.0, 7.0 Hz), 1.77 (s, 3H), 0.90 (s, 9H), 0.07 (s, 6H).
Wittig Olefination. To a solution of the pure E-enal 24 (20.4 g, 89.3 mmol) in toluene (200 mL) was added (carbethoxymethylene)triphenylphosphorane (36.0 g, 103.3 mmol, 1.2 equiv) at rt. The reaction was refluxed for 12 h before it was cooled down and concentrated in vacuo. The residue was dissolved in hexanes and the precipitated triphenylphosphine oxide was filtered off. The filtrate was concentrated in vacuo to afford the crude (E,E)-dienoate that was purified using gradient silica gel flash column chromatography (2-20% EtOAc in hexanes) to provide desired (E,E)-dienoate (25.6 g, 85.8 mmol) as colorless oil in 96% yield. Rf = 0.72 (10% EtOAc in hexanes); 1H NMR (500MHz, CDCl3) δ 7.33 (d, 1H, J = 15.5 Hz), 5.92 (t, 1H, J = 7.5 Hz), 5.81 (d, 1H, J = 15.5 Hz), 4.21 (q, 2H, J = 7.0 Hz), 3.68 (t, 2H, J = 7.0 Hz), 2.43 (dt, 2H, J = 7.0, 7.0 Hz), 1.79 (s, 3H), 1.30 (t, 3H, J = 7.0 Hz), 0.89 (s, 9H), 0.05 (s, 6H).
Dibal-H Reduction. To a solution of the above (E,E)-dienoate (25.6 g, 85.8 mmol) in anhyd CH2Cl2 (200 mL) was added DIBAL-H (188.8 mL, 1.0 M solution in hexanes, 188.8 mmol, 2.2 equiv) at -78 oC slowly. The reaction was stirred at -78 oC for 1 h and the TLC analysis indicated the complete conversion from the starting material to the product. The reaction was quenched with MeOH (40 mL) at -78 oC carefully then to the reaction was added sat aq sodium potassium tartrate (150 mL) at -78 oC slowly. The resulting mixture was allowed to warm up to rt and stirred until the organic layer and aqueous layer were completely separated.
The reaction mixture was extracted with Et2O (3 x 100 mL) and the combined organic layers were washed with sat aq NaCl (200 mL), dried over Na2SO4, and concentrated in vacuo. The crude product was purified using gradient silica gel flash column chromatography (5-30% EtOAc in hexanes) to afford desired (E,E)-diene 25 (20.4 g, 79.5 mmol) as colorless oil in 93% yield. (E,E)-Diene 25: Rf = 0.25 (30% EtOAc in hexanes); 1H NMR (500MHz, CDCl3) δ 6.27 (dd, 1H, J = 1.0, 15.5 Hz), 5.75 (td, 1H, J = 6.0, 15.5 Hz), 5.51 (t, 1H, J = 7.0 Hz), 4.21 (dd, 2H, J = 1.0, 6.0 Hz), 3.64 (t, 2H, J = 7.0 Hz), 2.38 (dt, 2H, J = 7.0, 7.0 Hz), 1.77 (d, 3H, J = 1.0 Hz), 0.90 (s, 9H), 0.06 (s, 6H); 13C NMR (125MHz, CDCl3) δ 136.1, 134.6, 128.8, 125.7, 63.5, 62.6, 32.1, 25.9, 18.3, 12.5, -5.3; IR (neat) cm-1 3369brs, 3020m, 2958s, 2860s, 1472s, 1388m, 1361s, 1253s, 1088s, 992s, 966s, 938s, 841s, 813s, 776s, 664m; mass spectrum (GC-MS): m/e (% relative intensity) 199 (46) (M+-t-Bu), 181 (3), 105 (100), 89 (48), 75 (70), 57 (3); m/e calcd for C14H28NaO2Si 279.1756, found 279.1755.
Preparation of Ketone 28.
Lithiated 2,3-DHF Addition. To a solution of 2,3-dihydrofuran (4.54 mL, 60.0 mmol) in THF (30 mL) was added a solution of t-BuLi (1.7 M in pentane, 35.3 mL, 60.0 mmol) at -78 °C dropwise. The resulting bright yellow solution was allowed to warm up to 0 °C slowly and stirred at 0 °C for an additional 1 h. The reaction mixture was re-cooled down to -78 °C, before a solution of acetaldehyde (3.70 mL, 65.9 mmol, 1.09 equiv) in THF (30 mL) was added via a cannula at -78 oC. After 1 h, TLC analysis indicated the disappearance of the starting material. The reaction was quenched by water at -78 oC dropwise then allowed to warm up to room temperature and extracted with Et2O (3 x 50 mL). The combined organic layers were washed with sat aq NaCl, dried over Na2SO4, and concentrated in vacuo to afford the corresponding alcohol, which was used in the next step without further purification: Rf = 0.30 (40% EtOAc in hexanes); 1H NMR (300MHz, CDCl3) δ 4.86 (dt, 1H, J = 1.2, 2.4 Hz), 4.40 (t, 2H, J = 9.6 Hz), 4.43-4.34 (m, 1H), 2.67 (ddt, 2H, J = 1.5, 2.4, 9.6 Hz), 1.39 (d, 3H, J = 6.6 Hz).
TBS-Protection: To a solution of the above alcohol in CH2Cl2 (100 mL) were added imidazole (8.17 g, 120.0 mmol, 2.0 equiv) and TBSCl (9.04 g, 60.0 mmol, 1.0 equiv) sequentially at 0 oC. The resulting mixture was allowed to warm up to room temperature and stirred for an additional 3 h. TLC analysis indicated the disappearance of the starting material. The reaction mixture was quenched by water and extracted with CH2Cl2 (3x60 mL). The combined organic layers were washed with sat aq NaCl, dried over Na2SO4, and concentrated in vacuo. The crude product was purified using gradient silica gel flash column chromatography (2-10% EtOAc in hexanes) to afford desired dihydro-furan 26: (8.21 g, 36.0 mmol) as colorless oil in 60% yield over two steps. Rf = 0.68 (10% EtOAc in hexanes); 1H NMR (300MHz, CDCl3) δ 4.81 (dt, 1H, J = 1.2, 2.4 Hz), 4.36 (t, 2H, J = 9.3 Hz), 4.38-4.30 (m, 1H), 2.63 (ddt, 2H, J = 1.5, 2.4, 9.3 Hz), 1.32 (d, 3H, J = 6.3 Hz).
Cyclic Acetal Formation. To a solution of dihydrofuran 26 (510.2 mg, 2.24 mmol) and diene 25 (686.2 mg, 2.68 mmol, 1.2 equiv) in CH2Cl2 (10 mL) was added PPTS (28.2 mg, 0.112 mmol, 0.05 equiv) at 0 oC. The resulting solution was allowed to warm up to room temperature slowly and stirred for 12 h. The reaction was quenched by sat aq NaHCO3 and extracted with CH2Cl2 (3x30 mL). The combined organic layers were washed by sat aq NaCl, dried over Na2SO4, and concentrated in vacuo. The crude product was purified using gradient silica gel flash column chromatography (2%-10% EtOAc in hexanes) to afford desired cyclic acetal 27 (858.2 mg, 1.77 mmol) as a 1:1 inseparable mixture in 79% yield: Rf = 0.70 (10% EtOAc in hexanes); 1H NMR (500MHz, CDCl3) δ 6.23 (d, 1H, J = 15.5 Hz), 5.68-5.59 (m, 1H), 5.47-5.42 (m, 1H), 4.15-4.09 (m, 1H), 4.00-3.94 (m, 3H), 3.90-3.84 (m, 1H), 3.62 (t, 1H, J = 7.0 Hz), 2.36 (q, 2H, J = 7.0 Hz), 2.17 (ddd, 1H, J = 9.0, 9.0, 12.5 Hz), 2.02-1.93 (m, 2H), 1.89-1.80 (m, 1H), 1.75 (s, 3H), 1.17-1.15 (m, 3H), 0.90 (s, 9H), 0.88 (s, 9H), 0.05 (s, 12H).
Desilylation-Silylation-Oxidation Sequence. To a solution of cyclic acetal 27 (858.2 mg, 1.77 mmol) in THF (10 mL) was added a solution of TBAF (1.0 M in THF, 5.3 mmol, 3.0 equiv) at 0 oC. The resulting mixture was allowed to warm up to room temperature and stirred for 48 h. The reaction mixture was partitioned between water and Et2O (3 x 60 mL). The combined organic layers were washed by saturated NaCl, dried over Na2SO4, and concentrated in vacuo. The crude product was purified using gradient silica gel flash column chromatography (10-60% EtOAc in hexanes) to afford desired diol (389.2 mg, 1.52 mmol) as a 1:1 inseparable mixture in 86% yield.
To a solution of above diol (389.2 mg, 1.52 mmol) in CH2Cl2 (5 mL) was added imidazole (217.1 mg, 3.19 mmol, 2.1 equiv) and TBSCl (240.0 mg, 1.59 mmol, 1.05 equiv) sequentially at 0 oC. The resulting mixture was allowed to warm up to room temperature and stirred for 2 h. After TLC analysis indicated the disappearance of the starting material, the reaction was quenched by water and extracted with CH2Cl2 (3x40 mL). The combined organic layers were washed by sat aq NaCl, dried over Na2SO4, and concentrated in vacuo. The crude product was purified using gradient silica gel flash column chromatography (5-30% EtOAc in hexanes) to afford desired mono-TBS protected alcohol (502.3 mg, 1.36 mmol) as a 1:1 inseparable mixture in 89% yield.
To a solution of aforementioned mono-TBS protected alcohol (652.2 mg, 1.76 mmol) in CH2Cl2 (8 mL) was added powdered 4Å molecular sieves (0.80 g), NMO (309.5 mg, 2.60 mmol, 1.5 equiv), and TPAP (89.6 mg, 0.093 mmol, 0.05 equiv) sequentially at room temperature. The resulting mixture was stirred for 2 h and TLC showed the complete conversion from the alcohol to the corresponding ketone. The reaction mixture was filtered through a pad of silica gel to remove the molecular sieves and the inorganic salt. The filtrate was concentrated in vacuo to afford the crude product, which was purified using gradient silica gel flash column chromatography (2-20% EtOAc in hexanes) to afford the desired ketone 28 (601.2 mg, 1.63 mmol) as colorless oil in 93% yield.
Ketone 28: Rf = 0.62 (20% EtOAc in hexanes); 1H NMR (500MHz, CDCl3) δ 6.25 (d, 1H, J = 15.5 Hz), 5.64 (dt, 1H, J = 6.0, 15.5 Hz), 5.49 (t, 1H, J = 7.5 Hz), 4.10-4.04 (m, 3H), 3.89 (ddd, 1H, J = 1.0, 6.5, 12.0 Hz), 3.62 (t, 2H, J = 7.0 Hz), 2.36 (q, 2H, J = 7.5 Hz), 2.25 (s, 3H), 2.15-2.08 (m, 2H), 2.01-1.93 (m, 2H), 1.75 (s, 3H), 0.89 (s, 9H), 0.05 (s, 6H); 13C NMR (125MHz, CDCl3) δ 205.5, 137.5, 134.4, 129.2, 122.7, 109.0, 69.0, 64.3, 62.5, 34.4, 32.0, 25.9, 25.6, 24.2, 18.2, 12.4, -5.3; IR (neat) cm-1 3034w, 2953s, 2929s, 2886s, 2857s, 1730s, 1471m, 1463m, 1386m, 1335m, 1225s, 1186m, 1099s, 966m, 939m, 835s, 777s; mass spectrum (ESI): m/e (% relative intensity) 391.2 (100) (M+Na)+; m/e calcd for C20H36NaO4Si 391.2281, found 391.2288.
The Use of Eschenmoser's Salt in Alpha-Methylenation and Cyclic Acetal Tethered IMDA.
Eschenmoser's Alpha-Methylenation. To a solution of ketone 28 (140.0 mg, 0.38 mmol) in THF (3 mL) was added a solution of LiHMDS (1.0 M in THF, 0.53 mL, 0.53 mmol, 1.4 equiv) at -78 °C. The resulting mixture was warmed up to 0 °C and stirred for 1 h. The reaction mixture was re-cooled down to -78 °C before a suspension of Eschenmoser's salt (84.4 mg, 0.46 mmol, 1.2 equiv) in THF (3 mL) was added via a cannula. The reaction was allowed to warm up to room temperature slowly and stirred for an additional 1 h. After TLC analysis indicated that the reaction was complete, the reaction was quenched with sat aq NaHCO3 (10 mL) and extracted with CH2Cl2 (5x10 mL). The combined organic layers were washed with sat aq NaCl (40 mL), dried over Na2SO4, and concentrated in vacuo. The crude product was purified using gradient silica gel flash column chromatography (5-80% MeOH in EtOAc) to afford the desired β-dimethylamino ketone intermediate (98.7 mg, 0.23 mmol) in 61% yield.
To a solution of the above β-dimethylamino ketone (30.0 mg, 0.070 mmol) in MeOH (3 mL) were added Na2CO3 (37.0 mg, 0.35 mmol, 5.0 equiv) and MeI (0.040 mL, 0.70 mmol, 10.0 equiv) sequentially at room temperature. The reaction was stirred for 12 h and TLC indicated the disappearance of the starting material. The reaction was quenched by water (5 mL) and extracted with CH2Cl2 (5x5 mL). The combined organic layers were washed with saturated NaCl (20 mL), dried over Na2SO4, and concentrated in vacuo. The crude product was purified using gradient silica gel flash column chromatography (0-5% EtOAc in hexanes) to afford the Diels-Alder cycloadduct 30-endo-I (12.0 mg, 0.032 mmol) as colorless oil in 45% yield overall. 30-endo-I: Rf = 0.71 (10% EtOAc in hexanes); 1H NMR (500MHz, CDCl3) δ 5.26 (ddd, 1H, J = 1.5, 1.5, 5.0 Hz), 4.01 (ddd, 1H, J = 6.0, 8.0, 8.0 Hz), 3.96-3.91 (m, 2H), 3.69 (ddd, 1H, J = 5.0, 8.0, 10.0 Hz), 3.64 (ddd, 1H, J = 7.0, 7.0, 10.0 Hz), 3.55 (ddd, 1H, J = 1.0, 5.0, 12.0 Hz), 2.85-2.79 (m, 1H), 2.64 (ddd, 1H, J = 5.0, 5.0, 13.5 Hz), 2.60 (ddd, 1H, J = 9.0, 9.0, 13.0 Hz), 2.28-2.20 (m, 1H), 2.04-1.90 (m, 4H), 1.81-1.73 (m, 2H), 1.70 (s, 3H), 1.36 (dddd, 1H, J = 5.0, 7.0, 10.0, 17.0 Hz), 0.89 (s, 9H), 0.05 (s, 6H); 13C NMR (125MHz, CDCl3) δ 205.5, 140.5, 119.9, 107.0, 69.6, 61.5, 60.8, 46.8, 38.2, 36.4, 35.8, 32.8, 28.5, 26.0, 24.4, 21.6, 18.3, -5.2, -5.3; IR (neat) cm-1 2952s, 2884s, 2857s, 1723s, 1255m, 1151m, 1093s, 1027s, 837s, 776s; mass spectrum (ESI): m/e (% relative intensity) 403.2 (100) (M+Na)+; m/e calcd for C21H36NaO4Si 403.2281, found 403.2280.
The experimental details for the reaction sequence leading to cyclic acetal 31 and ketone 32 are the same as those described for 40 and 41 below and the Eschenmoser's alpha-methylenation of ketone 32 and the ensuing facile IMDA in MeOH are the same as described above for ketone 28.
Cyclic Acetal 31: Rf = 0.41 (30 % EtOAc in hexanes); 1H NMR (500 MHz, CDCl3) δ 0.04 (s, 6H), 0.88 (s, 9H), 1.02-1.05 (m, 3H), 1.32-1.44 (m, 2H), 1.74 (s, 3H), 1.77-2.08 (m, 4H), 2.35 (q, 2H, J = 6.5 Hz), 3.61 (t, 2H, J = 7.0 Hz), 3.69 (dddd, 1H, J = 3.0, 3.0, 10.0, 14.0 Hz) 3.89-3.99 (m, 3H), 4.09-4.15 (m, 1H), 5.46 (t, 1H, J = 6.5 Hz), 5.56-5.66 (m, 1H), 6.24 (t, 1H, J = 15.5 Hz); 13C NMR (125 MHz, CDCl3): δ -5.13, 11.2, 12.6, 18.45, 24.3, 24.5, 24.9, 30.3, 31.9, 32.1, 62.2, 62.7, 68.7, 68.9, 72.6, 74.8, 110.6, 111.1, 123.6, 123.7, 128.7, 128.8, 134.6, 136.6, 137.7; IR (neat) cm-1 3488br, 2956s, 2932s, 2859s, 1463m, 1101s.
Ketone 32: Rf = 0.62 (30 % EtOAc in hexanes); 1H NMR (500 MHz, CDCl3) δ 0.01 (s, 6H), 0.85 (s, 9H), 1.02-1.05 (m, 3H), 1.71 (s, 3H), 1.89-1.93 (m, 2H), 2.07-2.10 (m, 2H), 2.32 (q, 2H, J = 7.0 Hz), 2.56-2.61 (m, 2H), 3.59 (t, 2H, J = 7.5 Hz), 3.84 (dd, 1H, J = 7.0, 12.5 Hz), 3.99-4.03 (m, 3H), 5.45 (t, 1H, J = 7.5 Hz), 5.60 (td, 1H, J = 6.5, 15.5 Hz), 6.20 (d, 1H, J = 16.0 Hz); 13C NMR (125 MHz, CDCl3): δ -5.2, 7.4, 12.4, 18.0, 24.1, 25.9, 31.0, 31.1, 34.8, 62.6, 64.4, 69.0, 109.1, 122.8, 129.1, 134.5, 137.4, 208.4; IR (neat) cm-1 2932s, 2858s, 1730s, 1462m, 1254m.
Diels-Alder Cycloadduct 34-Endo-I: Rf = 0.30 (20 % EtOAc in hexanes); 1H NMR (500 MHz, CDCl3) δ 0.02 (s, 6H), 0.08 (s, 9H), 1.08 (s, 3H), 1.32-1.38 (m, 2H), 1.66 (s, 3H), 1.72 (ddd, 1H, J = 5.0, 8.5, 17.5 Hz), 1.83-1.96 (m, 3H), 2.07 (t, 1H, J = 12.5 Hz), 2.15-2.25 (m, 1H), 2.42-2.45 (m, 1H), 2.55 (dt, 1H, J = 9.5, 12.5 Hz), 3.54 (ddd, 1H, J = 1.0, 5.0, 12.0 Hz), 3.57-3.67 (m, 2H), 3.86-3.99 (m, 3H), 5.21 (m, 1H); 13C NMR (125 MHz, CDCl3): δ -5.3, 18.2, 20.1, 22.6, 25.9, 32.6, 33.1, 33.6, 35.5, 44.1, 45.4, 60.5, 62.5, 69.5, 107.0, 119.4, 138.6, 207.3; IR (neat) cm-1 2954s, 2936s, 2883s, 2859s, 1720s, 1459m, 1254m; mass spectrum (LCMS) for C22H38O4Si: m/e (% relative intensity) 395 (100) (M+H)+, 377 (61), 263 (26); m/e calcd for C22H38NaO4Si 417.2437, found 417.2430.
Desilylation of Cycloadduct 34-Endo-I: It was carried using TBAF according to the procedure described for cyclic acetal 27. Free Alcohol 35: Rf = 0.40 (50 % EtOAc in hexanes); 1H NMR (500 MHz, CDCl3) δ 1.07 (s, 3H), 1.32 (ddd, 1H, J = 1.0, 5.5, 13.0 Hz), 1.37-1.44 (m, 1H), 1.66 (s, 3H), 1.70 (ddd, 1H, J = 5.0, 8.5, 18 Hz), 1.86-2.21 (m, 5H), 2.41- 2.45 (m, 1H), 2.53 (dt, 1H, J = 8.5, 13.0 Hz), 3.54 (dd, 1H, J = 5.0, 12.0 Hz), 3.58-3.68 (m, 2H), 3.84-3.97 (m, 3H), 5.21-5.22 (m, 1H); 13C NMR (125 MHz, CDCl3): δ 20.0, 21.4, 24.3, 32.7, 33.1, 33.6, 35.3, 44.0, 45.4, 60.2, 62.5, 69.6, 107.0, 119.7, 138.3, 207.4; IR (neat) cm-1 3418br, 2943s, 2882s, 1718s, 1454m, 1156m, 1096m, 1034s; mass spectrum (LCMS) for C16H24O4: m/e (% relative intensity) 281 (10) (M+H)+, 263 (100), 245 (6).
Para-Bromobenzoyl Ester 36 Formation: This was accomplished using standard acylation procedures. Ester 36: Rf = 0.42 (20 % EtOAc in hexanes); mp: 105-107 0C; 1H NMR (500 MHz, CDCl3) δ 1.12 (s, 3H), 1.40-1.43 (m, 1H), 1.71 (s, 3H), 1.67-1.76 (m, 2H), 1.86-2.05 (m, 2H), 2.16-2.25 (m, 3H), 2.47-2.49 (m, 1H), 2.54-2.61 (m, 1H), 3.58 (dd, 1H, J = 4.5, 12.0 Hz), 3.84-3.96 (m, 3H), 4.28-4.33 (m, 1H), 4.36-4.41 (m, 1H), 5.29 (dd, 1H, J = 1.5, 3.0 Hz), 7.54-7.57 (m, 2H), 7.86-7.88 (m, 2H); 13C NMR (125 MHz, CDCl3): δ 20.0, 21.4, 24.3, 31.2, 33.0, 33.3, 33.6, 44.1, 45.3, 62.4, 63.0, 69.6, 107.0, 120.5, 128.0, 129.1, 131.1, 131.6, 137.4, 165.7, 206.9; IR (neat) cm-1 2963s, 2928m, 2884m, 1721s, 1591m, 1271s; mass spectrum (LCMS) for C23H27BrO5: m/e (% relative intensity) 464 (24) (M+/Br81), 462 (24) (M+/Br79), 445 (23), 263 (100); m/e calcd for C23H27BrNaO5 485.0940, found 485.0945.
Epimerization of Diels-Alder Cycloadduct 30-Endo-I.
To a solution of the Diels-Alder cycloadduct 30-Endo-I (12.0 mg, 0.032 mmol) in MeOH (2 mL) was added K2CO3 (36.3 mg, 0.26 mmol, 8.2 equiv) at rt. The reaction mixture was stirred at rt for 12 h. The reaction was diluted with water (4 mL) and extracted with CH2Cl2 (3 x 10 mL). The combined organic layers were washed with sat aq NaCl (10 mL), dried over Na2SO4, and concentrated in vacuo. The crude product was purified using gradient silica gel flash column chromatography (0-5% EtOAc in hexanes) to afford 30-Exo-II with trans-ring junction (10.0 mg, 0.026 mmol) as colorless oil in 82% yield.
30-Exo-II: Rf = 0.71 (10% EtOAc in hexanes); 1H NMR (500MHz, CDCl3) δ 5.16 (sextet, 1H, J = 1.5 Hz), 4.03 (ddd, 1H, J = 6.5, 8.0, 8.0 Hz), 3.95-3.90 (m, 1H), 3.86 (dd, 1H, J = 11.0, 12.0 Hz), 3.68 (dd, 1H, J = 4.0, 11.0 Hz), 3.68-3.60 (m, 2H), 2.75 (ddd, 1H, J = 2.0, 12.0, 12.0 Hz), 2.60 (ddd, 1H, J = 8.0, 9.0, 13.0 Hz), 2.51 (dddq, 1H, J = 2.0, 4.0, 12.0, 12.0 Hz), 2.22-2.17 (m, 1H), 2.08-1.86 (m, 3H), 1.83-1.76 (m, 1H), 1.75 (ddd, 1H, J = 5.5, 8.0, 13.5 Hz), 1.70 (s, 3H), 1.50 (dddd, 1H, J = 1.0, 6.0, 12.0, 13.5 Hz), 1.35 (dddd, 1H, J = 4.0, 5.5, 10.0, 14.0 Hz), 0.89 (s, 9H), 0.05 (s, 6H); mass spectrum (ESI): m/e (% relative intensity) 403 (100) (M+Na)+; m/e calcd for C21H36NaO4Si 403.2281, found 403.2275.
Preparation of 37 From Diethyl Allyl Malonate. (i) LAH Reduction. To a solution of LAH (10.0 g, 263.5 mmol, 2.1 equiv) in anhyd Et2O (250 mL) was added via a cannula a solution of known diethyl allylmalonate (25.0 mL, 126.8 mmol) in anhyd Et2O (150 mL) at 0 oC. The reaction was allowed to warm up to rt and stirred for 12 h. TLC analysis indicated the disappearance of the starting material and the reaction was quenched with dropwise addition of water. The precipitated white aluminum salt was filtered off and the aluminum salt was washed thoroughly with Et2O (3 x 150 mL). The filtrate was washed with sat aq NaCl (100 mL), dried over Na2SO4, and concentrated in vacuo. The crude product was purified using gradient silica gel flash column chromatography (10-80% EtOAc in hexanes) to afford desired 1,3-diol (12.3 g, 105.9 mmol) as colorless oil in 84% yield.
1,3-Diol Intermediate: Rf = 0.18 (60% EtOAc in hexanes); 1H NMR (500MHz, CDCl3) δ 5.81 (ddt, 1H, J = 7.0, 10.0, 17.0 Hz), 5.08 (ddt, 1H, J = 1.5, 1.5, 17.0 Hz), 5.06-5.03 (m, 1H), 3.82 (ddd, 2H, J = 4.0, 5.5, 10.5 Hz), 3.68 (ddd, 2H, J = 5.0, 7.0, 11.0 Hz), 2.22-2.17 (m, 2H), 2.08 (dddd, 2H, J = 1.0, 1.0, 7.0, 7.0 Hz), 1.92-1.84 (m, 1H).
(ii) Di-Benzylation. To a solution of the above 1,3-diol (16.3 g, 140.3 mmol) in anhyd THF (300 mL) and DMF (60 mL) was added the NaH (12.0 g, 60% suspension in mineral oil, 300.0 mmol, 2.1 equiv) in several portions at 0 oC. The resulting white suspension was allowed to warm up to rt and stirred for 45 min. The resulting mixture was cooled back down to 0 oC before a solution of benzyl bromide (51.0 g, 298.2 mmol, 2.1 equiv) in anhyd THF (100 mL) was added via a cannula. The reaction was allowed to warm up to rt and stirred for 12 h. The reaction was quenched with water (100 mL) and extracted with Et2O (2 x 100 mL). The combined organic layers were washed with sat aq NaCl (200 mL), dried over Na2SO4, and concentrated in vacuo. The crude product was purified using gradient silica gel flash column chromatography (2-20% EtOAc in hexanes) to afford desired alkene 37 (41.6 g, 140.3 mmol) as colorless oil in quantitative yield.
Alkene 37: Rf = 0.73 (20% EtOAc in hexanes); 1H NMR (500MHz, CDCl3) δ 7.36-7.27 (m, 10H), 5.79 (ddt, 1H, J = 7.0, 10.0, 17.0 Hz), 5.03 (ddt, 1H, J = 2.0, 2.0, 17.0 Hz), 5.00 (m, 1H), 4.50 (s, 4H), 3.51 (dd, 2H, J = 6.0, 9.0 Hz), 3.48 (dd, 2H, J = 5.0, 9.0 Hz), 2.21 (dd, 2H, J = 7.0, 7.0 Hz), 2.04 (septet, 1H, J = 6.0 Hz); 13C NMR (125MHz, CDCl3) δ 138.8, 136.7, 128.4, 127.6, 127.5, 116.4, 73.2, 70.6, 39.4, 33.3; IR (neat) cm-1 3064m, 3030m, 2902s, 2857s, 1495m, 1453s, 1364s, 1099s, 1028m, 996m, 913m, 736s, 698s; mass spectrum (GC-MS): m/e (% relative intensity) 205 (66) (M+-Bn), 91 (100), 81 (4), 63 (2).
Dihydroxylation. To a solution of alkene 37 (41.6 g, 140.3 mmol) in acetone (270 mL) and water (30 mL) were added N-methyl morpholine N-oxide (16.4 g, 140.3 mmol, 1.0 equiv) and K2OsO4.2H2O (103.4 mg, 0.28 mmol, 0.5mol%) sequentially at rt. The reaction was stirred at rt for 12 h and TLC analysis indicated the disappearance of the starting material. The reaction was quenched with sat aq sodium metabisulfite (250 mL) and extracted with EtOAc (3 x 100 mL). The combined organic layers were washed with sat aq NaCl (200 mL), dried over Na2SO4, and concentrated in vacuo. The crude product was purified using gradient silica gel flash column chromatography (5-100% EtOAc in hexanes) to afford desired 1,2-diol (44.5 g, 134.7 mmol) as colorless oil in 96% yield.
1,2-Diol Intermediate: Rf = 0.20 (EtOAc); 1H NMR (500MHz, CDCl3) δ 7.37-7.26 (m, 10H), 4.52 (s, 2H), 4.50 (s, 2H), 3.78 (ddd, 1H, J = 3.5, 7.0, 13.0 Hz), 3.60-3.56 (m, 2H), 3.51 (dd, 1H, J = 5.5, 9.5 Hz), 3.47-3.43 (m, 3H), 2.21 (septet, 1H, J = 6.0 Hz), 1.59 (ddd, 1H, J = 2.5, 6.5, 14.0 Hz), 1.52 (ddd, 1H, J = 6.0, 9.0, 14.0 Hz).
NaIO4-Oxidative Cleavage. To a solution of the above 1,2-diol intermediate (44.5 g, 134.7 mmol) in THF (180 mL) and water (90 mL) was added the NaIO4 (30.0 g, 140.3 mmol, 1.0 equiv) at 0 oC. The resulting mixture was allowed to warm up to rt and stirred for 3h. After TLC analysis showed that the reaction was complete, the reaction was diluted with water (200 mL) and extracted with Et2O (3 x 150 mL). The combined organic layers were washed with sat aq NaCl (200 mL), dried over Na2SO4, and concentrated in vacuo. The crude product was purified using gradient silica gel flash column chromatography (2-30% EtOAc in hexanes) to afford desired aldehyde 38 (36.2 g, 121.3 mmol) as colorless oil in 90% yield.
Aldehyde 38: Rf = 0.65 (20% EtOAc in hexanes); 1H NMR (500MHz, CDCl3) δ 9.77 (t, 1H, J = 2.0 Hz), 7.37-7.26 (m, 10H), 4.48 (s, 4H), 3.55 (dd, 2H, J = 5.0, 9.0 Hz), 3.45 (dd, 2H, J = 6.0, 9.0 Hz), 2.63 (septet, 1H, J = 6.0 Hz), 2.54 (dd, 2H, J = 2.0, 6.0 Hz); 13C NMR (125MHz, CDCl3) δ 201.9, 138.4, 128.5, 128.4, 127.7, 127.6, 73.2, 70.7, 44.0, 35.1; IR (neat) cm-1 3087m, 3063m, 3029s, 2858s, 2725m, 1720s, 1495s, 1478m, 1453s, 1408m, 1389m, 1364s, 1205s, 1099s, 1028s, 741s, 699s; mass spectrum (ESI): m/e (% relative intensity) 353.2 (100) (M+MeOH+Na)+, 321.2 (18) (M+Na)+; m/e calcd for C20H26NaO4 353.1729, found 353.1720.
Preparation of Alcohol 40.
Lithiated 2,3-DHF Addition. To a solution of 2,3-dihydrofuran (4.11 mL, 54.3 mmol, 1.2 equiv) in anhyd THF (30 mL) was added t-BuLi (32.0 mL, 1.7 M in Pentane, 54.4 mmol, 1.2 equiv) at -78 °C dropwise. The resulting bright yellow solution was allowed to warm up to 0 °C slowly and stirred at 0 °C for an additional 1 h. The reaction mixture was re-cooled down to -78 °C, and a solution of aldehyde 38 (13.5 g, 45.3 mmol, 1.0 equiv) in anhyd THF (30 mL) was added via a cannula at -78 °C. After 1 h, TLC analysis indicated the disappearance of the starting material. The reaction was quenched with water (12 mL) at -78 oC dropwise, allowed to warm up to rt, and extracted with Et2O (3 x 50 mL). The combined organic layers were washed with sat aq NaCl (100 mL), dried over Na2SO4, and concentrated in vacuo. The crude furanyl alcohol (16.7 g, 45.3 mmol) was sufficiently pure judging by 1H NMR and used the next step without further purification. The hydroxyl group of the above furanyl alcohol was capped using Ac2O, DMAP, and pyridine in CH2Cl2 at rt for 12 h following standard conditions to afford dihydrofuran 39 in 70% yield over two steps from 38.
Furanyl alcohol: Rf = 0.33 (20% EtOAc in hexanes); 1H NMR (500MHz, CDCl3) δ 7.36-7.27 (m, 10H), 4.85 (t, 1H, J = 2.5 Hz), 4.51 (s, 4H), 4.34 (ddd, 2H, J = 2.5, 9.0, 9.0 Hz), 4.31-4.27 (m, 1H), 3.57-3.53 (m, 3H), 3.46 (dd, 2H, J = 6.0, 9.0 Hz), 2.62 (dddd, 2H, J = 2.0, 2.0, 9.5, 9.5 Hz), 2.24-2.18 (m, 1H), 1.83 (ddd, 1H, J = 4.0, 7.0, 14.5 Hz), 1.75 (ddd, 1H, J = 6.0, 8.5, 14.5 Hz).
Dihydrofuran 39: 1H NMR (500 MHz, CDCl3) δ 7.33 (m, 10H), 5.54 (t, 1H, J = 6.5 Hz), 4.90 (t, 1H, J = 2.5 Hz), 4.50 (d, 4H, J = 4.5 Hz), 4.34 (t, 2H, J = 9.5 Hz), 3.52 (m, 4H), 2.61 (td, 2H, J = 2.5, 9.5 Hz), 2.06 (s, 3H), 2.02 (sept, 1H, J = 6.5 Hz), 1.91 (ddd, 1H, J = 6.5, 6.5, 13.5 Hz), 1.86 (ddd, 1H, J = 6.5, 6.5, 13.5 Hz); 13C NMR (125 MHz, CDCl3) δ 170.2, 155.7, 138.6, 138.6, 128.4, 128.3, 128.3, 127.6, 127.6, 127.5, 127.5, 97.9, 73.2, 73.1, 70.8, 70.3, 70.1, 67.6, 36.0, 31.0, 29.8, 21.2, 14.2; IR (neat) cm-1 3030m, 2860s, 1740s, 1668w, 1453m, 1368m, 1237s, 1094m; mass spectrum (APCI): m/e (% relative intensity) 351.2 (100) (M++OAc), 243.1 (30).
Cyclic Acetal Formation. To a solution of dihydrofuran 39 (10.6 g, 25.9 mmol) and (E,E)-Diene 25 (7.96 g, 31.0 mmol, 1.2 equiv) in anhyd CH2Cl2 (100 mL) was added the pyridinium p-toluenesulfonate (325.0 mg, 1.29 mmol, 5 mol%) at 0 oC. The resulting solution was allowed to warm up to rt slowly and stirred for 12 h. After TLC analysis indicated the disappearance of the starting material, the reaction was quenched with sat aq NaHCO3 (100 mL) and extracted with CH2Cl2 (3 x 60 mL). The combined organic layers were washed with sat aq NaCl (150 mL), dried over Na2SO4, and concentrated in vacuo. The crude product was purified using gradient silica gel flash column chromatography (2%-10% EtOAc in hexanes) to afford the acylated cyclic acetal intermediate (13.6 g, 20.4 mmol) as a 1.5:1 mixture in 79% yield. The pure major isomer was isolated after a second gradient flash chromatography for characterization.
Acylated Cyclic Acetal Intermediate: 1H NMR (500 MHz, CDCl3) δ 7.30 (m, 10H), 6.29 (d, 1H, J = 16.0 Hz), 6.15 (d, 1H, J = 16.0 Hz), 5.62 (dt, 1H, J = 6.0, 16.0 Hz), 5.58 (dt, 1H, J = 6.0, 16.0 Hz), 5.52 (d, 1H, J = 12.0 Hz), 5.46 (d, 1H, J = 7.0 Hz), 5.42 (t, 1H, J = 7.0 Hz), 5.37 (dd, 1H, J = 2.0, 10.0 Hz), 4.51 (m, 4H), 4.18 (m, 1H), 4.09 (d, 1H, J = 6.5 Hz), 3.95 (m, 2H), 3.93 (m, 1H), 3.50-3.62 (m, 6H), 3.48 (m, 1H), 2.38 (p, 2H, J = 6.5 Hz), 2.06 (s, 3H), 2.05 (s, 3H), 1.92-2.00 (m, 3H), 1.80-1.90 (m, 2H), 1.78 (s, 3H), 1.73 (s, 3H), 0.91 (s, 9H), 0.08 (s, 6H); 13C NMR (125 MHz, CDCl3) δ 170.5, 170.4, 138.8, 138.7, 138.6, 136.7, 136.6, 134.8, 134.7, 128.7, 128.6, 128.4, 128.3, 128.3, 128.3, 127.6, 127.6, 127.5, 127.5, 127.5, 127.4, 126.7, 123.9, 123.6, 112.1, 109.5, 108.9, 81.2, 71.8, 71.6, 71.4, 69.9, 69.3, 69.1, 68.7, 63.0, 62.7, 62.7, 62.5, 62.2, 36.1, 36.1, 34.7, 33.3, 32.2, 31.7, 31.6, 31.3, 28.9, 28.8, 26.0, 25.3, 24.5, 24.2, 22.7, 21.2, 21.1, 20.6, 18.4, 14.2, 12.6, 12.5, -5.2; IR (neat) cm-1 2953s, 2859s, 1745s, 1455m, 1368m, 1239s, 1098s; mass spectrum (APCI): m/e (% relative intensity) 411.2 (35) (M+-diene), 351.2 (100), 303.1 (30), 261.2 (40), 243.1 (15).
The above acylated cyclic acetal intermediate was deacylated using K2CO3 in MeOH at rt following standard conditions to afford alcohol 40 in 75% yield over two steps from 39.
Alcohol 40 [major isomer]: Rf = 0.35 (40% EtOAc in hexanes); 1H NMR (500MHz, CDCl3) δ 7.35-7.26 (m, 10H), 6.15 (d, 1H, J = 16.0 Hz), 5.55 (dt, 1H, J = 6.0, 16.0 Hz), 5.42 (t, 1H, J = 7.0 Hz), 4.52 (s, 2H), 4.49 (s, 2H), 4.10 (dd, 1H, J = 6.5, 12.0 Hz), 4.00 (ddd, 1H, J = 2.0, 2.0, 11.0 Hz), 3.98-3.90 (m, 3H), 3.61 (t, 2H, J = 7.0 Hz), 3.60-3.53 (m, 3H), 3.50 (dd, 1H, J = 6.0, 9.0 Hz), 2.62 (d, 1H, J = 1.0 Hz), 2.35 (q, 2H, J = 7.0 Hz), 2.28-2.23 (m, 1H), 2.12-2.01 (m, 2H), 1.93-1.76 (m, 3H), 1.72 (s, 3H), 1.42 (ddd, 1H, J = 5.0, 10.5, 14.0 Hz), 0.90 (s, 9H), 0.05 (s, 6H); 13C NMR (125MHz, CDCl3) δ 138.5, 136.6, 134.7, 128.6, 128.3, 127.6, 127.5, 127.4, 123.8, 111.1, 73.2, 73.1, 71.9, 70.7, 69.3, 68.6, 62.7, 62.1, 36.8, 32.2, 31.3, 30.3, 26.0, 24.5, 18.4, 12.5, -5.2; IR (neat) cm-1 3463brs, 3030m, 2952s, 2927s, 2856s, 1470m, 1362m, 1253m, 1098s, 1026s, 965m, 835s, 777m, 735m, 698m; mass spectrum (ESI): m/e (% relative intensity) 1271.8 (16) (2xM++Na+), 647.4 (100) (M+Na)+; m/e calcd for C37H56NaO6Si 647.3744, found 647.3748.
Preparation of Ketone 41.
To a solution of alcohol 40 (3.20 g, 5.10 mmol) in anhyd CH2Cl2 (25 mL) was added the molecular sieves (2.50 g), N-methyl morpholine N-oxide (0.90 g, 7.60 mmol, 1.5 equiv), and tetra-n-propyl ammonium perruthenate (89.6 mg, 0.26 mmol, 0.05 equiv) sequentially at rt. The resulting mixture was stirred for 2 h at rt and TLC showed the complete conversion from the alcohol to the corresponding ketone. The reaction mixture was filtered through a pad of silica gel to remove the molecular sieves and the inorganic salt. The filtrate was concentrated in vacuo to afford the crude product that was purified using gradient silica gel flash column chromatography (2-20% EtOAc in hexanes) to afford desired ketone 41 (2.90 g, 4.60 mmol) as colorless oil in 91% yield.
Ketone 41: Rf = 0.75 (40% EtOAc in hexanes); 1H NMR (500MHz, CDCl3) δ 7.35-7.24 (m, 10H), 6.21 (d, 1H, J = 16.0 Hz), 5.61 (dt, 1H, J = 6.5, 16.0 Hz), 5.45 (t, 1H, J = 7.0 Hz), 4.47 (s, 2H), 4.46 (s, 2H), 4.07-4.00 (m, 3H), 3.84 (dd, 1H, J = 6.0, 12.0 Hz), 3.61 (t, 2H, J = 7.0 Hz), 3.53 (dd, 1H, J = 5.0, 9.0 Hz), 3.52 (dd, 1H, J = 4.5, 9.0 Hz), 3.48 (dd, 2H, J = 5.5, 9.0 Hz), 2.76 (dd, 1H, J = 7.0, 18.5 Hz), 2.71 (dd, 1H, J = 6.0, 18.5 Hz), 2.67-2.61 (m, 1H), 2.35 (q, 2H, J = 7.0 Hz), 2.10-2.00 (m, 2H), 1.95-1.84 (m, 2H), 1.73 (d, 3H, J = 1.0 Hz), 0.89 (s, 9H), 0.05 (s, 6H); 13C NMR (125MHz, CDCl3) δ 206.8, 138.5, 137.5, 134.6, 129.1, 128.3, 127.6, 127.5, 123.0, 109.2, 73.0, 70.6, 70.3, 69.1, 64.5, 62.7, 37.0, 34.9, 34.6, 32.2, 26.0, 24.2, 18.4, 12.6, -5.2; IR (neat) cm-1 3032w, 2953s, 2929s, 2857s, 1727s, 1455m, 1362m, 1254m, 1098s, 1036s, 966m, 835s, 777m, 736m, 698m; mass spectrum (ESI): m/e (% relative intensity) 645.4 (100) (M+Na)+, 1267.8 (10) (2xM++Na); m/e calcd for C37H54NaO6Si 645.3587, found 645.3587.
Eschenmoser's Salt in Alpha-Methylenation.
To a solution of ketone 41 (140.0 mg, 0.22 mmol) in anhyd THF (2 mL) was added lithium bis(trimethylsilyl) amide (0.30 mL, 1.0 M solution in THF, 0.30 mmol, 1.4 equiv) at -78 °C dropwise. The resulting mixture was warmed up to 0 oC and stirred for 1 h. The reaction mixture was re-cooled down to -78 °C before a suspension of Eschenmoser’s salt (50.0 mg, 0.27 mmol, 1.2 equiv) in anhyd THF (2 mL) was added via a cannula. The reaction was allowed to warm up to rt slowly and stirred at rt for an additional 1 h. After TLC analysis indicated that the reaction was complete, the reaction was quenched with sat aq NaHCO3 (10 mL) and extracted with CH2Cl2 (5 x 10 mL). The combined organic layers were washed with sat aq NaCl (40 mL), dried over Na2SO4, and concentrated in vacuo. The crude product was purified using gradient silica gel flash column chromatography (5-100% EtOAc in hexanes) to afford desired major β-dimethylaminoketone (69.0 mg, 0.10 mmol) and minor β-dimethylaminoketone (31.0 mg, 0.046 mmol) in 46% and 21% yields, respectively.
Major Isomer: Rf = 0.15 (EtOAc); 1H NMR (500MHz, CDCl3) δ 7.35-7.25 (m, 10H), 6.23 (d, 1H, J = 16.0 Hz), 5.66 (dt, 1H, J = 6.5, 16.0 Hz), 5.45 (t, 1H, J = 7.5 Hz), 4.50 (d, 1H, J = 12.0 Hz), 4.47 (s, 2H), 4.45 (d, 1H, J = 12.0 Hz), 4.05 (dd, 1H, J = 5.5, 7.5 Hz), 4.04-4.02 (m, 1H), 4.00-3.95 (m, 2H), 3.65-3.53 (m, 5H), 3.62 (t, 2H, J = 7.0 Hz), 2.60 (dd, 1H, J = 8.0, 12.0 Hz), 2.42 (quintet, 1H, J = 6.0 Hz), 2.36 (q, 2H, J = 7.5 Hz), 2.32 (dd, 1H, J = 6.0, 12.0 Hz), 2.16 (s, 6H), 2.20-1.96 (m, 3H), 1.86-1.78 (m, 1H), 1.74 (s, 3H), 0.90 (s, 9H), 0.06 (s, 6H); 13C NMR (125MHz, CDCl3) δ 209.8, 138.7, 138.6, 137.1, 134.8, 128.7, 128.3, 128.2, 127.6, 127.5, 127.4, 123.5, 109.6, 73.0, 72.8, 69.3, 69.1, 68.6, 64.3, 62.7, 58.6, 45.8, 43.2, 40.1, 34.0, 32.1, 26.0, 24.3, 18.4, 12.5, -5.2; mass spectrum (ESI): m/e (% relative intensity) 680.5 (100) (M+H)+.
Minor Isomer: Rf = 0.12 (EtOAc); 1H NMR (500MHz, CDCl3) δ 7.34-7.25 (m, 10H), 6.20 (d, 1H, J = 15.5 Hz), 5.64 (dt, 1H, J = 6.0, 15.5 Hz), 5.45 (t, 1H, J = 7.5 Hz), 4.56 (d, 1H, J = 12.0 Hz), 4.47 (d, 1H, J = 12.0 Hz), 4.46 (d, 1H, J = 12.0 Hz), 4.44 (d, 1H, J = 12.0 Hz), 4.05-3.95 (m, 3H), 3.91 (dd, 1H, J = 6.5, 12.0 Hz), 3.65 (dd, 1H, J = 6.0, 10.0 Hz), 3.62 (t, 2H, J = 7.0 Hz), 3.60 (dd, 1H, J = 5.0, 9.0 Hz), 3.59 (dd, 1H, J = 7.0, 9.5 Hz), 3.47 (ddd, 1H, J = 4.0, 4.0, 10.0 Hz), 3.44 (dd, 1H, J = 6.5, 9.5 Hz), 2.72 (dd, 1H, J = 10.0, 12.0 Hz), 2.47-2.41 (m, 1H), 2.36 (q, 2H, J = 7.0 Hz), 2.27-2.21 (m, 2H), 2.13 (s, 6H), 2.16-2.04 (m, 1H), 1.97 (ddd, 1H, J = 4.5, 8.5, 13.0 Hz), 1.92-1.84 (m, 1H), 1.74 (s, 3H), 0.90 (s, 9H), 0.06 (s, 6H); 13C NMR (125MHz, CDCl3) δ 209.2, 138.7, 138.6, 137.1, 134.8, 128.6, 128.3, 127.5, 127.4, 123.5, 109.9, 73.0, 72.7, 69.4, 69.3, 68.0, 64.4, 62.7, 58.1, 45.9, 43.4, 39.2, 34.3, 32.1, 26.0, 24.6, 18.4, 12.5, -5.2; mass spectrum (ESI): m/e (% relative intensity) 680.4 (100) (M+H)+.
To a solution of the above β-dimethylaminoketone (91.0 mg, 0.13 mmol) in MeOH (3 mL) was added Na2CO3 (74.0 mg, 0.70 mmol, 5.4 equiv) and MeI (0.080 mL, 1.30 mmol, 10.0 equiv) sequentially at rt. The reaction was stirred for 12 h and TLC indicated the disappearance of the starting material. The reaction was quenched with water (5 mL) and extracted with CH2Cl2 (5 x 10 mL). The combined organic layers were washed with sat aq NaCl (40 mL), dried over Na2SO4, and concentrated in vacuo. The crude product was purified using gradient silica gel flash column chromatography (2-20% EtOAc in hexanes) to afford the Diels-Alder endo adducts 43 (13.0 mg, 0.020 mmol) as a 12:1 mixture in favoring 43-endo-I, and the desired cycloaddition precursor 42 (50.0 mg, 0.079 mmol) as colorless oils in 15% and 61% yields, respectively.
Cycloaddition Precursor 42: Rf = 0.69 (20% EtOAc in hexanes); 1H NMR (500MHz, CDCl3) δ 7.33-7.26 (m, 10H), 6.69 (s, 1H), 6.17 (d, 1H, J = 16.0 Hz), 6.02 (s, 1H), 5.56 (dt, 1H, J = 6.5, 16.0 Hz), 5.43 (t, 1H, J = 7.5 Hz), 4.48 (s, 4H), 4.08 (dd, 1H, J = 6.0, 12.0 Hz), 4.04-4.00 (m, 2H), 3.81 (dd, 1H, J = 7.0, 13.0 Hz), 3.66-3.59 (m, 4H), 3.61 (t, 2H, J = 7.0 Hz), 3.44-3.40 (m, 1H), 2.35 (q, 2H, J = 7.0 Hz), 2.19-2.16 (m, 1H), 2.11-2.04 (m, 2H), 1.89-1.82 (m, 1H), 1.72 (d, 3H, J = 1.0 Hz), 0.89 (s, 9H), 0.05 (s, 6H); 13C NMR (125MHz, CDCl3) δ 197.4, 143.8, 138.4, 137.5, 134.6, 129.1, 128.7, 128.3, 127.6, 127.5, 127.4, 122.7, 109.4, 73.0, 70.4, 70.2, 68.2, 64.6, 62.7, 40.8, 36.8, 32.2, 26.0, 24.2, 18.4, 12.5, -5.2; IR (neat) cm-1 3030w, 2953s, 2929s, 2859s, 1727m, 1687s, 1455s, 1362s, 1255s, 1100s, 1029s, 966s, 835s, 777m, 736m, 698m; mass spectrum (ESI): m/e (% relative intensity) 657.4 (100) (M+Na)+; m/e calcd for C38H54NaO6Si 657.3587, found 657.3676.
Cyclic Acetal Tethered IMDA Using 42 and De-Silylation.
A solution of 42 (27.0 mg, 0.042 mmol) in anhyd toluene (10 mL) was cannulated into a sealed tube and was refluxed for 48 h. The TLC analysis indicated the disappearance of the starting material. The reaction mixture was concentrated in vacuo to afford the crude product that was purified using gradient silica gel flash column chromatography (2-10% EtOAc in hexanes) to afford the inseparable Diels-Alder endo adducts 43-endo-I and 43-endo-II (24.0 mg, 0.038 mmol) as a 1:1 inseparable mixture in 90% yield.
To a solution of 1:1 mixture of the Diels-Alder endo adducts (24.0 mg, 0.038 mmol) in THF (1 mL) was added TBAF (0.10 mL, 1.0 M solution in THF, 0.1 mmol, 2.6 equiv) at rt. The reaction was stirred for 1 h at rt before it was diluted with water (1 mL) and extracted with CH2Cl2 (5 x 5 mL).
The combined organic layers were washed with sat aq NaCl (10 mL), dried over Na2SO4, and concentrated in vacuo. The crude product was purified using careful gradient silica gel flash column chromatography (5-30% EtOAc in hexanes) to afford pure 44-endo-I adduct (8.3 mg, 0.016 mmol) and 44-endo-II adduct (7.3 mg, 0.014 mmol) in 42% yield and 37% yields, respectively.
44-endo-I: Rf = 0.20 (30% EtOAc in hexanes); 1H NMR (500MHz, CDCl3) δ 7.35-7.26 (m, 10H), 5.21 (ddd, 1H, J = 2.0, 2.0, 5.5 Hz), 4.51 (d, 1H, J = 12.0 Hz), 4.47 (d, 1H, J = 12.0 Hz), 4.43 (s, 2H), 3.98-3.84 (m, 5H), 3.63 (ddd, 1H, J = 5.0, 8.0, 10.5 Hz), 3.60 (dd, 1H, J = 5.0, 12.0 Hz), 3.57 (ddd, 1H, J = 7.0, 7.0, 10.5 Hz), 3.54-3.48 (m, 2H), 3.05-2.99 (m, 1H), 2.35 (ddd, 1H, J = 8.0, 9.0, 13.0 Hz), 2.32-2.25 (m, 1H), 2.10 (dddd, 1H, J = 4.0, 4.0, 4.0, 8.0 Hz), 2.01-1.86 (m, 3H), 1.83 (dd, 1H, J = 11.0, 14.0 Hz), 1.75 (ddd, 1H, J = 1.5, 6.0, 14.0 Hz), 1.67 (ddd, 1H, J = 5.0, 8.0, 13.0 Hz), 1.67 (s, 3H), 1.35 (dddd, 1H, J = 5.0, 7.0, 9.5, 17.0 Hz); 13C NMR (125MHz, CDCl3) δ 208.4, 138.8, 138.6, 138.2, 128.4, 128.3, 127.9, 127.8, 127.6, 119.5, 107.4, 73.3, 73.0, 70.1, 69.6, 69.0, 62.5, 60.2, 49.0, 42.8, 39.4, 35.3, 35.1, 32.7, 31.3, 25.0, 21.2; IR (neat) cm-1 3466brs, 3029w, 2945s, 2916s, 2879s, 1714s, 1453s, 1363m, 1094s, 1073s, 1028s, 738s, 699s; mass spectrum (ESI): m/e (% relative intensity) 543.3 (100) (M+Na)+; m/e calcd for C32H40NaO6 543.2723, found 543.2730.
44-endo-II: Rf = 0.23 (30% EtOAc in hexanes); 1H NMR (500MHz, CDCl3) δ 7.35-7.24 (m, 10H), 5.34-5.31 (m, 1H), 4.51 (d, 1H, J = 12.0 Hz), 4.47 (d, 1H, J = 12.0 Hz), 4.46 (d, 1H, J = 12.0 Hz), 4.42 (d, 1H, J = 12.0 Hz), 4.24 (dd, 1H, J = 5.0, 12.0 Hz), 4.01 (ddd, 1H, J = 6.0, 8.0, 8.0 Hz), 3.88 (dd, 1H, J = 5.0, 10.0 Hz), 3.84 (dd, 1H, J = 7.0, 15.0 Hz), 3.73 (dd, 1H, J = 5.5, 10.0 Hz), 3.73-3.62 (m, 2H), 3.56 (dd, 1H, J = 4.0, 10.0 Hz), 3.52 (dd, 1H, J = 6.0, 11.5 Hz), 3.42 (dd, 1H, J = 6.0, 10.0 Hz), 3.12-3.07 (m, 1H), 2.51 (dq, 1H, J = 4.0, 6.0 Hz), 2.40 (td, 1H, J = 8.5, 13.0 Hz), 2.32-2.25 (m, 1H), 2.02-1.84 (m, 3H), 1.90 (dd, 1H, J = 7.0, 14.0 Hz), 1.83 (dd, 1H, J = 7.0, 14.0 Hz), 1.78 (ddd, 1H, J = 5.0, 8.0, 13.0 Hz), 1.70 (s, 3H), 1.32-1.24 (m, 1H); 13C NMR (125MHz, CDCl3) δ 207.4, 138.7, 138.6, 138.5, 128.3, 128.2, 127.7, 127.5, 127.3, 122.9, 106.9, 72.9, 72.8, 69.6, 69.2, 68.9, 64.5, 60.9, 49.3, 42.3, 37.3, 35.1, 34.0, 33.2, 29.6, 24.6, 21.7; IR (neat) cm-1 3452brs, 3029w, 2941s, 2878s, 1718s, 1495m, 1476m, 1453s, 1364m, 1093s, 1028s, 737s, 699s; mass spectrum (ESI): m/e (% relative intensity) 543.3 (100) (M+Na)+; m/e calcd for C32H40NaO6 543.2723, found 543.2715.
Re-Silylations of 44-endo-I and 44-endo-II, and Characterization 43-endo-I and 43-endo-II.
To a solution of the pure 44-endo-I (8.3 mg, 0.016 mmol) in anhyd CH2Cl2 (1 mL) was added imidazole (3.00 mg, 0.044 mmol, 2.8 equiv) and TBSCl (5.0 mg, 0.033 mmol, 2.1 equiv) sequentially at 0 oC. The resulting mixture was allowed to warm up to rt and stirred for an additional 1 h. After completion as indicated by TLC analysis, the reaction mixture was quenched with water (1 mL) and extracted with CH2Cl2 (3 x 5 mL). The combined organic layers were washed with sat aq NaCl (10 mL), dried over Na2SO4 and concentrated in vacuo. The crude product was purified using gradient silica gel flash column chromatography (2-10% EtOAc in hexanes) to afford 43-endo-I (7.50 mg, 0.012 mmol) as colorless oil in 75% yield.
DA Cycloadduct 43-endo-I: Rf = 0.76 (10% EtOAc in hexanes); 1H NMR (500MHz, CDCl3) δ 7.34-7.24 (m, 10H), 5.21 (ddd, 1H, J = 1.5, 1.5, 5.5 Hz), 4.50 (d, 1H, J = 11.5 Hz), 4.44 (d, 1H, J = 11.5 Hz), 4.42 (s, 2H), 3.96 (ddd, 1H, J = 6.5, 8.0, 8.0 Hz), 3.94-3.90 (m, 3H), 3.87 (dd, 1H, J = 11.5, 11.5 Hz), 3.65-3.55 (m, 4H), 3.54 (dd, 1H, J = 3.0, 10.0 Hz), 3.06-2.99 (m, 1H), 2.35 (ddd, 1H, J = 8.0, 9.0, 13.0 Hz), 2.31-2.23 (m, 1H), 2.14-2.09 (m, 1H), 1.99-1.86 (m, 3H), 1.84 (dd, 1H, J = 11.0, 14.0 Hz), 1.75 (ddd, 1H, J = 1.5, 6.0, 14.0 Hz), 1.67 (ddd, 1H, J = 5.0, 8.0, 13.0 Hz), 1.66 (s, 3H), 1.32 (dddd, 1H, J = 5.0, 7.0, 9.5, 17.0 Hz), 0.90 (s, 9H), 0.06 (s, 3H), 0.05 (s, 3H); 13C NMR (125MHz, CDCl3) δ 208.5, 139.1, 138.7, 138.3, 128.3, 128.2, 127.9, 127.8, 127.6, 127.5, 119.2, 107.5, 73.3, 73.1, 70.0, 69.5, 68.8, 62.6, 60.7, 49.0, 42.6, 39.2, 35.9, 35.2, 33.0, 31.4, 26.0, 25.0, 21.3, 18.3, -5.2; IR (neat) cm-1 3027w, 2953s, 2930s, 2882s, 2858s, 1716s, 1454s, 1362m, 1255s, 1095s, 1029s, 837s, 777s, 737s, 698s; mass spectrum (ESI): m/e (% relative intensity) 657.4 (100) (M+Na)+; m/e calcd for C38H54NaO6Si 657.3587, found 657.3576.
To a solution of the pure 44-endo-II (7.3 mg, 0.014 mmol) in anhyd CH2Cl2 (1 mL) was added imidazole (2.40 mg, 0.035 mmol, 2.5 equiv) and TBSCl (4.40 mg, 0.029 mmol, 2.1 equiv) sequentially at 0 oC. The resulting mixture was allowed to warm up to rt and stirred for an additional 1 h. After completion as indicated using TLC analysis, the reaction mixture was quenched with water (1 mL) and extracted with CH2Cl2 (3 x 5 mL). The combined organic layers were washed with sat aq NaCl (10 mL), dried over Na2SO4 and concentrated in vacuo. The crude product was purified using gradient silica gel flash column chromatography (2-10% EtOAc in hexanes) to afford desired 43-endo-II (7.20 mg, 0.011 mmol) as colorless oil in 81% yield.
DA Cycloadduct 43-endo-II. Rf = 0.76 (10% EtOAc in hexanes); 1H NMR (500MHz, CDCl3) δ 7.34-7.24 (m, 10H), 5.33-5.31 (m, 1H), 4.51 (d, 1H, J = 12.0 Hz), 4.48 (d, 1H, J = 12.0 Hz), 4.46 (d, 1H, J = 12.0 Hz), 4.43 (d, 1H, J = 12.0 Hz), 4.24 (dd, 1H, J = 5.0, 11.5 Hz), 4.02 (dt, 1H, J = 6.0, 8.0 Hz), 3.86-3.81 (m, 2H), 3.78 (dd, 1H, J = 7.0, 10.0 Hz), 3.72-3.61 (m, 2H), 3.58 (dd, 1H, J = 4.0, 10.0 Hz), 3.53 (dd, 1H, J = 7.0, 11.5 Hz), 3.48 (dd, 1H, J = 5.0, 10.0 Hz), 3.18-3.13 (m, 1H), 2.53-2.48 (m, 1H), 2.41 (td, 1H, J = 8.5, 12.5 Hz), 2.31-2.24 (m, 1H), 1.97 (tdd, 1H, J = 8.0, 12.0, 15.0 Hz), 1.89-1.78 (m, 4H), 1.77 (ddd, 1H, J = 5.0, 8.0, 13.0 Hz), 1.70 (s, 3H), 1.32-1.24 (m, 1H), 0.90 (s, 9H), 0.06 (s, 6H); 13C NMR (125MHz, CDCl3) δ 206.9, 138.8, 138.6, 128.3, 128.2, 127.6, 127.5, 127.4, 127.2, 122.6, 112.3, 106.9, 73.0, 72.7, 69.7, 69.1, 68.8, 64.6, 61.0, 49.2, 42.1, 36.8, 35.5, 33.8, 33.2, 29.7, 26.0, 24.6, 21.8, 18.3, -5.2, -5.3; IR (neat) cm-1 3027w, 2949s, 2928s, 2855s, 1719s, 1453s, 1362m, 1252s, 1096s, 1028s, 835s, 776s, 735s, 697s; mass spectrum (ESI): m/e (% relative intensity) 657.4 (100) (M+Na)+; m/e calcd for C38H54NaO6Si 657.3587, found 657.3625.
Preparation of Hydroxy Ketone 46.
Dihydroxylation. To a solution of 43-endo-II (86.7 mg, 0.137 mmol) in pyridine (8 mL) was added OsO4 (42.0 mg, 0.164 mmol, 1.2 equiv) at -10 oC and the resulting solution turned dark brown slowly. After 1 h, the reaction was quenched by sat aq sodium metabisulfite then allowed to warm up to room temperature and stirred for an additional 1 h. The heterogeneous mixture was partitioned between EtOAc and H2O. The combined organic layers were washed by sat aq NaCl, dried over Na2SO4, and concentrated in vacuo to afford the crude product which was purified by gradient flash column chromatography (10%-40% EtOAc in hexanes) to provide desired diol 45 (83.7 mg, 0.125 mmol) as colorless oil in 91% yield as single diastereomer.
Diol 45 [For P = TBS]: Rf = 0.35 (50% EtOAc in hexanes); 1H NMR (500MHz, CDCl3) δ 7.35-7.24 (m, 10H), 4.51 (d, 1H, J = 12.0 Hz), 4.47 (d, 1H, J = 12.0 Hz), 4.44 (d, 1H, J = 12.0 Hz), 4.43 (dd, 1H, J = 2.0, 12.0 Hz), 4.37 (d, 1H, J = 12.0 Hz), 4.02 (dt, 1H, J = 5.5, 8.0 Hz), 3.99 (dd, 1H, J = 2.0, 11.0 Hz), 3.89 (dd, 1H, J = 7.0, 15.0 Hz), 3.83 (dd, 1H, J = 5.0, 10.0 Hz), 3.78 (dd, 1H, J = 3.0, 10.0 Hz), 3.72-3.68 (m, 1H), 3.52 (dt, 1H, J = 4.5, 9.5 Hz), 3.40 (dd, 1H, J = 8.0, 11.0 Hz), 3.35 (dd, 1H, J = 8.0, 10.0 Hz), 3.32 (dd, 1H, J = 4.0, 10.0 Hz), 3.06-3.01 (m, 1H), 2.92 (brs, 1H), 2.54 (dt, 1H, J = 8.5, 13.0 Hz), 2.45-2.41 (m, 1H), 2.43 (brs, 1H), 2.04-1.95 (m, 1H), 1.93-1.85 (m, 2H), 1.84-1.79 (m, 1H), 1.79 (ddd, 1H, J = 4.5, 8.0, 13.0 Hz), 1.70 (dd, 1H, J = 4.5, 14.5 Hz), 1.56 (dddd, 1H, J = 5.5, 5.5, 8.5, 14.0 Hz), 1.20 (s, 3H), 1.01 (dddd, 1H, J = 4.5, 4.5, 9.0, 14.0 Hz), 0.90 (s, 9H), 0.06 (s, 6H); 13C NMR (125MHz, CDCl3) δ 205.8, 138.6, 138.2, 128.3, 128.2, 127.8, 127.6, 127.5, 127.4, 107.6, 73.3, 73.0, 72.7, 71.4, 69.8, 68.4, 67.7, 62.2, 57.4, 51.7, 42.5, 41.2, 40.8, 33.9, 32.3, 26.0, 24.3, 23.7, 18.3, -5.3, -5.4; mass spectrum (ESI): m/e (% relative intensity) 691 (100) (M+Na)+; m/e calcd for C38H56NaO8Si 691.3642, found 691.3650.
Parikh-Doering Oxidation. To a solution of diol 45 (34.7 mg, 0.052 mmol) in CH2Cl2 (1.5 mL) was added DMSO (0.1 mL) and Et3N (0.1 mL) sequentially at rt. The reaction mixture was cooled down to 0 oC before SO3·Py complex (41.3 mg, 0.259 mmol, 5.0 equiv) was added in one portion. The reaction was stirred at 0 oC for 1 h then allowed to warm up to rt. After 5 h, additional SO3-Py (41.3 mg, 0.259 mmol, 5.0 equiv) was added and the solution was stirred overnight. The reaction was quenched by H2O and extracted with EtOAc (3 x 10 mL). The combined organic layers were washed by sat aq NaCl, dried over Na2SO4 and concentrated in vacuo to afford the crude product which was purified by gradient flash column chromatography (10-30% EtOAc in hexanes) to afford the desired hydroxy ketone 46 (10.4 mg, 0.0156 mmol) as colorless oil in 30% yield.
Preparation of Diol 45” from 43”. Same as that described for 45 from 43.
Diol 45” [For P = TES]: Rf = 0.30 (50% EtOAc in hexanes); 1H NMR (500 MHz, CDCl3) δ 7.25-7.33 (m, 10H), 4.52 (d, 1H, J = 5.0 Hz), 4.49 (d, 1H, J = 5.0 Hz), 4.44 (d, 2H, J = 11.5 Hz), 4.37 (d, 1H, J = 12.0 Hz), 4.02 (m, 2H), 3.90 (q, 1H, J = 7.0 Hz), 3.83 (dd, 1H, J = 1.0, 5.5 Hz), 3.78 (dd, 1H, J = 1.0, 6.5 Hz), 3.72 (p, 1H, J = 5.0 Hz), 3.51 (sextet, 1H, 5.0 Hz), 3.40 (m, 1H), 3.33 (m, 2H), 3.05 (m, 1H), 2.64 (brs, 1H), 2.55 (dt, 1H, J = 8.5, 12.5 Hz), 2.45 (d, 1H, J = 11.5 Hz), 2.00 (m, 1H), 1.75-1.95 (m, 4H), 1.70 (dd, 1H, J = 4.0, 14.0 Hz), 1.59 (td, 1H, J = 6.0, 14.0 Hz), 1.18 (s, 3H), 1.02 (m, 1H), 0.95 (t, 9H, J = 7.5 Hz), 0.60 (q, 6H, J = 7.5 Hz); 13C NMR (125 MHz, CDCl3) δ 205.8, 138.6, 138.2, 128.3, 128.3, 127.8, 127.6, 127.6, 127.4, 107.6, 73.2, 73.0, 72.7, 71.3, 69.8, 68.5, 67.7, 61.8, 57.4, 51.8, 42.5, 41.2, 40.8, 33.9, 32.4, 24.8, 24.3, 24.2, 23.7, 6.8, 4.6, 4.3, 4.2, 4.1; IR (neat) cm-1 3343brs, 3065w, 2955s, 2876s, 1715s, 1455s, 1366w, 1239w, 1095s; mass spectrum (APCI): m/e (% relative intensity) 668.0 (35) (M+), 650.9 (10), 560.9 (90), 542.9 (100), 524.9 (10), 428.9 (25); m/e calcd for C38H56O8Si 668.3745, found 668.3746.
Preparation of Hydroxy Ketone 46”. Same as that described for 46 from 45.
Hydroxy Ketone 46” [For P = TES]: Rf = 0.45 (50% EtOAc in hexanes); 1H NMR (500 MHz, CDCl3) δ 7.25-7.35 (m, 10H), 4.45 (m, 2H), 4.38 (s, 2H), 4.34 (dd, 1H, J = 5.0, 12.5 Hz), 4.00 (dd, 2H, J = 1.5, 12.0 Hz), 3.98 (dd, 1H, J = 6.0, 7.5 Hz), 3.93 (q, 1H, J = 7.5 Hz), 3.72 (ddd, 2H, J = 5.5, 10.5, 15.5 Hz), 3.62 (dd, 2H, J = 6.0, 6.5 Hz), 3.45-3.49 (m, 2H), 3.39 (dd, 1H, J = 3.5, 10.0 Hz), 2.92 (m, 1H), 2.38 (dt, 1H, J = 9.0, 12.5 Hz), 1.84-2.12 (m, 7H), 1.60 (s, 1H), 1.25 (m, 1H), 1.16 (s, 3H), 0.94 (t, 9H, J = 7.5 Hz), 0.58 (q, 6H, J = 7.5 Hz); 13C NMR (125 MHz, CDCl3) δ 213.7, 205.3, 164.8, 138.1, 138.0, 128.4, 128.3, 127.8, 127.7, 127.7, 127.6, 117.2, 107.0, 80.4, 76.6, 73.1, 73.0, 69.7, 69.0, 68.1, 61.3, 58.8, 51.0, 49.4, 43.6, 38.9, 35.5, 32.6, 30.2, 24.4, 19.1, 6.9, 4.4; mass spectrum (APCI): m/e (% relative intensity) 666.0 (10) (M+), 650.2 (10), 541.0 (100), 523.3 (20), 427.0 (35); m/e calcd for C38H54O8Si 666.3588, found 666.3578.
Hydroxy Ketone 47’’: A fresh solution of LDA was added dropwise to a solution of 46’’ in THF at -78 °C to afford an inseperable 1:1 mixture of 46’’ and 47’’. Characterization of 47’’ proved difficult, with many of the 1H NMR signals overlapping slightly with 46’’; however, the clean doubling of signal at 2.38 (dt, 1H, J = 9.0, 12.5 Hz) with a second signal at 2.30 (dt, 1H, J = 9.0, 12.5 Hz), as well as doubling of singlet at 1.16 (s, 3H) with a singlet at 1.18 (s, 3H) provided some direct evidence, in addition to 13C NMR showing doubling of most signals. 13C NMR (125 MHz, CDCl3) δ 213.7, 212.0, 205.3, 164.8, 138.2, 138.1, 138.0, 128.4, 128.3, 128.3, 128.2, 127.9, 127.8, 127.7, 127.7, 127.6, 127.6, 127.5, 127.4, 117.2, 107.2, 107.0, 104.8, 80.4, 76.8, 76.6, 76.6, 73.9, 73.1, 73.1, 72.9, 69.9, 69.7, 69.0, 69.0, 68.1, 67.8, 61.5, 61.3, 59.0, 58.8, 52.6, 51.0, 50.7, 49.4, 45.3, 43.6, 40.0, 38.9, 35.5, 35.4, 32.8, 32.6, 30.3, 30.1, 24.4, 24.0, 20.5, 19.1, 6.8, 4.4.
ACKNOWLEDGEMENTS
RPH thanks University of Wisconsin for financial support, and JW thanks University of Minnesota for a prestigious Lester C. and Joan Krogh Graduate Dissertation Fellowship. We thank Dr. William W. Brennessel and Dr. Victor G. Young, Jr. of University of Minnesota for providing X-ray structural analysis.
References
1. K. Krohn, C. Biele, K.-H. Drogies, K. Steingröver, H.-J. Aust, S. Draeger, and B. Schulz, Eur. J. Org. Chem., 2002, 2331. CrossRef
2. For another rare example, see: R. B. Woodward, T. Fukunaga, and R. C. Kelly, J. Am. Chem. Soc., 1964, 86, 3162. CrossRef
3. W. A. Ayer, L. M. Browne, A. W. Singer, and P. Elgersma, Can. J. Chem., 1990, 68, 1300. CrossRef
4. For isolation and biological activities, see: (a) C. Y. Kao and S. R. Levinson, Eds. ‘Tetrodotoxin, Saxitoxin, and the Molecular Biology of the Sodium Channel.’ New York Academy of Sciences: New York, 1986; Vol. 479; (b) T. Goto, Y. Kishi, S. Takahashi, and Y. Hirata, Tetrahedron, 1965, 21, 2059; CrossRef (c) H. S. Mosher, F. A. Fuhrman, H. D. Buchwald, and H. G. Fischer, Science, 1964, 144, 1100; CrossRef (d) K. Tsuda, S. Ikuma, M. Kawamura, R. Tachikawa, K. Sakai, C. Tamura, and O. Amakasu, Chem. Pharm. Bull., 1964, 12, 1357; (d) R. B. Woodward, Pure Appl. Chem., 1964, 9, 49; CrossRef (e) T. Narahashi, J. Toxicol., Toxin Rev., 2001, 20, 67. CrossRef
5. For total syntheses and synthetic efforts, see: (a) S. Akai, H. Seki, N. Sugita, T. Kogure, N. Nishizawa, K. Suzuki, Y. Nakamura, Y. Kajihara, J. Yoshimura, and K.-I. Sato, Bull. Chem. Soc. Jpn., 2010, 83, 279; CrossRef (b) D. Urabe, T. Nishikawa, and M. Isobe, Chem. Asian J., 2006, 1, 125; CrossRef (c) T. Umezawa, T. Hayashi, H. Sakai, H. Teramoto, T. Yoshikawa, M. Izumida, Y. Tamatani, T. Hirose, Y. Ohfune, and T. Shinada, Org. Lett., 2006, 8, 4971; CrossRef (d) T. Nishikawa, D. Urabe, and M. Isobe, Angew. Chem. Int. Ed., 2004, 43, 4782; CrossRef (e) A. Hinman and J. Du Bois, J. Am. Chem. Soc., 2003, 125, 11510; CrossRef (f) N. Ohyabu, T. Nishikawa, and M. Isobe, J. Am. Chem. Soc., 2003, 125, 8798; CrossRef (g) T. Nishikawa, D. Urabe, K. Yoshida, T. Iwabuchi, M. Asai, and M. Isobe, Pure Appl. Chem., 2003, 75, 251; CrossRef (h) Y. Kishi, M. Aratani, T. Fukuyama, F. Nakatsubo, T. Goto, S. Inoue, H. Tanino, S. Sugiura, and H. Kakoi, J. Am. Chem. Soc., 1972, 94, 9217; CrossRef (i) Y. Kishi, T. Fukuyama, M. Aratani, F. Nakatsubo, T. Goto, S. Inoue, H. Tanino, S. Sugiura, and H. Kakoi, J. Am. Chem. Soc., 1972, 94, 9219; CrossRef (j) T. Itoh, M. Watanabe, and T. Fukuyama, Synlett, 2002, 1323; CrossRef (k) K. Sato, S. Akai, N. Sugita, T. Ohsawa, T. Kogure, H. Shoji, and J. Yoshimura, J. Org. Chem., 2005, 70, 7496; CrossRef (l) K. Sato, S. Akai, H. Shoji, N. Sugita, S. Yoshida, Y. Nagai, K. Suzuki, Y. Nakamura, Y. Kajihara, M. Funabashi, and J. Yoshimura, J. Org. Chem., 2008, 73, 1234. CrossRef
6. X. Gao, and B. S. Snider, J. Org. Chem., 2004, 69, 5517. CrossRef
7. J. Wang, R. P. Hsung, and S. K. Ghosh, Org. Lett., 2004, 6, 1939. CrossRef
8. For reviews, see: (a) B. R. Bear, S. M. Sparks, and K. J. Shea, Angew. Chem. Int. Ed., 2001, 40, 820; CrossRef (b) D. Craig, ’Stereoselective Synthesis’ Vol. E21c ed. by G. Helmchen, R. W. Hoffmann, J. Mulzer, and E. Schaumann, Thieme: Stuttgardt, 1996, pp. 2872-2904; (c) W. R. Roush, in ‘Advances in Cycloaddition,’ Vol. 2 ed. by D. P. Curran, JAI: Greenwich, CT, 1990, pp. 91-146; (d) W. R. Roush, in ‘Comprehensive Organic Synthesis’ Vol. 5 ed. by B. M. Trost, JAI: Pergamon, Oxford, 1991, pp. 513-550; (e) D. Craig, Chem. Soc. Rev., 1987, 16, 187; CrossRef (f) E. Ciganek, Org. React., 1984, 32, 1; (g) A. G. Fallis, Can. J. Chem., 1984, 62, 183; CrossRef (h) D. F. Taber, in ‘Intramolecular Diels-Alder Reactions and Alder-Ene Reactions’ Springer: Berlin, 1984.
9. P. J. Ainsworth, D. Craig, A. J. P. White, and D. J. Williams, Tetrahedron, 1996, 52, 8937. CrossRef
10. (a) R. K. Boeckman Jr., and C. J. Flann, Tetrahedron Lett., 1983, 24, 1655; (b) R. K. Boeckman, Jr., K. G. Estep, S. G. Nelson, and M. A. Walters, Tetrahedron Lett., 1991, 32, 4095. CrossRef
11. W. R. Roush and D. A. Barba, Tetrahedron Lett., 1997, 38, 8781. CrossRef
12. (a) M. E. Jung and L. J. Street, J. Am. Chem. Soc., 1984, 106, 8327; CrossRef (b) M. E. Jung and L. J. Street, Heterocycles, 1988, 27, 45. CrossRef
13. For reviews, see: (a) C.-C. Liao and R. K. Peddinti, Acc. Chem. Res., 2002, 35, 856; CrossRef (b) S. Quideau and L. Pouységu, Org. Prep Proc. Int., 1999, 31, 617. CrossRef
14. For reviews, see: (a) M. H. D. Postema, Tetrahedron, 1992, 48, 8545; CrossRef (b) K. A. Parker, Pure Appl. Chem., 1994, 66, 2135; CrossRef (c) D. E. Levy and C. Tang, ‘The Chemistry of C-Glycosides.’ Vol. 13 Pergamon Press: 1995; (d) For an example, see: A. Hosomi, Y. Sakata, and H. Sakurai, Carbohydr. Res., 1987, 171, 223. CrossRef
15. (a) S. R. Shenoy, D. M. Smith, and K. A. Woerpel, J. Am. Chem. Soc., 2006, 128, 8671; CrossRef (b) D. M. Smith and K. A. Woerpel, Org. Lett., 2004, 6, 2063; CrossRef (c) J. A. C. Romero, S. A. Tabacco, and K. A. Woerpel, J. Am. Chem. Soc., 2000, 122, 168; CrossRef (d) H. Matsutani, S. Ichikawa, J. Yaruva, T. Kusumoto, and T. Hiyama, J. Am. Chem. Soc., 1997, 119, 4541; CrossRef (e) S. Hosokawa, B. Kirschbaum, and M. Isobe, Tetrahedron Lett., 1998, 39, 1917. CrossRef
16. S. K. Ghosh, R. P. Hsung, and J. Liu, J. Am. Chem. Soc., 2005, 127, 8260. CrossRef
17. (a) J. Liu and R. P. Hsung, Org. Lett., 2005, 7, 2273; CrossRef (b) J. Yang, J. Liu, and R. P. Hsung, Org. Lett., 2008, 10, 2525. CrossRef
18. For a review on chemistry of spiroketals, see: (a) J. E. Aho, P. M. Pihko, and T. K. Rissa, Chem. Rev., 2005, 105, 4406; CrossRef (b) K. T. Mead and B. N. Brewer, Curr. Org. Chem., 2003, 7, 227; CrossRef (c) M. A. Brimble and F. A. Fares, Tetrahedron, 1999, 55, 7661; CrossRef (d) M. T. Fletcher and W. Kitching, Chem. Rev., 1995, 95, 789; CrossRef (e) F. Perron and K. F. Albizati, Chem. Rev., 1989, 89, 1617. CrossRef
19. R. Figueroa, R. P. Hsung, and C. C. Guevarra, Org. Lett., 2007, 9, 4857. CrossRef
20. S. K. Ghosh, C. Ko, J. Liu, J. Wang, and R. P. Hsung, Tetrahedron, 2006, 62, 10485. CrossRef
21. J. Schreiber, H. Maag, N. Hashimoto, and A. Eschenmoser, Angew. Chem., Int. Ed. Engl., 1971, 10, 330. CrossRef
22. For a leading reference on hydrogen bonding promoted hetero Diels-Alder cycloadditions, see: Y. Huang and V. H. Rawal, J. Am. Chem. Soc., 2002, 124, 9662. CrossRef
23. For some earlier examples on effect of aqueous or protic solvents on Diels-Alder cycloadditions, see: (a) P. A. Grieco, P. Garner, and Z.-M. He, Tetrahedron Lett., 1983, 24, 1897; CrossRef (b) R. Breslow, U. Maitra, and D. Rideout, Tetrahedron Lett., 1983, 24, 1901. CrossRef
24. Intriguingly, we also examined Z,E-diene ii [prepared from 39 and (Z,E)-25] but the Diels-Alder cycloaddition was not only endo-selective but also gave 43 with an endo-I to endo-II ratio of 1:1, thereby implying isomerization of the olefin geometry took place under the reaction conditions. The use of Lewis acid was not useful in this example.