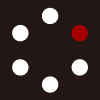
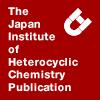
HETEROCYCLES
An International Journal for Reviews and Communications in Heterocyclic ChemistryWeb Edition ISSN: 1881-0942
Published online by The Japan Institute of Heterocyclic Chemistry
e-Journal
Full Text HTML
Received, 15th May, 2010, Accepted, 19th August, 2010, Published online, 20th August, 2010.
DOI: 10.3987/COM-10-S(E)31
■ Autocatalysis and Organocatalysis with Kemp’s Triacid Compounds
Dariush Ajami, Seiji Kamioka, Aaron C. Sather, Richard J. Hooley, and Julius Rebek, Jr.*
Department of Chemistry, The Skaggs Institute for Chemical Biology, The Scripps Research Institute, 10550 North Torrey Pines Road, La Jolla, California 92037, U.S.A.
Abstract
Synthetic structures capable of autocatalysis based on molecular recognition – self-replication – were introduced nearly 20 years ago. These systems involved neither informational oligomers such as nucleic acids nor conditions that are generally regarded as prebiotic, but they revealed how self-complementary molecules could act as templates for their own formation and helped define the structure of minimalist replicators. Recent expansions in the field of organocatalysis raise the possibility that a synthetic structure might behave as an autocatalyst and function as a chemical catalyst for other reactions. We show here that these properties can be engineered into a single synthetic structure: the compound can selectively accelerate its own formation and catalyzes hydrogenation reactions of nitrostyrene. It is likely that a wide variety of synthetic structures can be modified for autocatalysis and organocatalysis.INTRODUCTION
The unusual structure of Kemp’s triacid1 1a (Figure 1) offers advantages in chemical studies of molecular recognition2 and replication.3 The initial synthetic compound that showed autocatalysis based on recognition4 was the derivative 2, a structure that comprises adenine covalently bound to a receptor for adenine. This self-complementary feature allowed 2 to act as a template for its own formation by recognizing both components from which it is made as in 3.5
Earlier, in 1986 a hexameric, self-complementary DNA strand was shown to self-replicate6 and the same behavior was engineered into an RNA molecule 15 years later.7 The catalytic activity of RNA in other reactions had already emerged, discoveries which led to the postulation of an “RNA world”,8 where ribozymes functioned both as carriers of genetic information and performed the catalysis and regulation of reactions required in metabolism. Studies of the replication of simple organic molecules led to the many types of self-replicating structures9 presently known, including peptides.10 Yet these explorations did not lead to systems that were informational in the sense of containing a sequence that could be evolved, or to compounds that catalyzed reactions other than their own formation (or that of very closely related structures11). This latter shortcoming prompted the present research – the search for synthetic molecules that act as both catalysts and autocatalysts. While we make no claims that such compounds or conditions we use are conventionally prebiotic, we intend to show, through chemistry, that “autocatalytic reproduction of catalytic capabilities”12 as proposed by Eschenmoser is a attainable goal, and one that should be included in a definition of the chemistry at life’s origins. Recently we provided evidence that synthetic structures based on a xanthene skeleton act as both autocatalysts and organocatalysts when imidazolidinones13 or thiourea14 functions are embedded within them. Here we report the adaptation of a replicator based on Kemp’s triacid and its characterization as an autocatalyst and an organocatalyst.
RESULTS AND DISCUSSION
We chose to make modifications to a replicator 4 (Figure 2) that showed modest sigmoidal kineticbehavior15 and bearing a biphenyl spacer between the recognition sites (the imide and adenine). The minor intrusions on the structure leading to compound 5 – an amide linkage of the biaryl to the Kemp’s triacid, the substitution of a thiourea for the amide and the introduction of a nitro group to enhance the acidity of the thiourea – were not expected to interfere with the recognition elements involved in replication. The peripheral propyl groups of the Kemp’s triacid are there merely to improve its solubility in organic solvents.
The synthesis was uneventful and is outlined in Figure 3. Isothiocyanate 9a was generated from aniline 8 and proved to be very reactive to amine nucleophiles, a feature that posed some challenges for its characterization as a replicator. Specifically, the adenosine amine 10 gave the thiourea 5a on brief exposure (a few minutes) to 9a at ambient temperature. Following this reaction in real time by conventional kinetic methods using NMR or HPLC was not possible, so we considered competition experiments instead. In these experiments nucleophiles and electrophiles without recognition elements compete with those involved in the intended autocatalysis reaction. The product ratios were determined by HPLC based on integration of corresponding adsorption signals at 254 nm.
First, an electrophile lacking the imide recognition element was prepared to compete with 9a. This involved N-methylation of the imide function as in 9b at a site some distance from the isothiocyanate function undergoing reaction. The competition of 9a and 9b for a limited amount of adenosine amine 10 showed that the thiourea 5a was formed in greater amounts (thiourea 5a: N-methylated thiourea 5b = 62:38). When thiourea 5a was present at the beginning of the competition reaction, the preference increased by a small but measurable degree (Entry 2, 67:33, and Entry 3, 72:28, Table 1). Apparently, 5a was able to act as a template for its own formation. On other hand, when thiourea 5b, which is unable to act as a template for its own formation, was present at the beginning of the competition reaction, the product ratios did not change. In short, these results indicated that molecular recognition at the imide group was involved in the reaction and 5a enhanced its own formation, acting as an autocatalyst.
Second, competition experiments of 9a and 9b with benzyl amine 11 (a nucleophile that lacks a recognition site) show no preference between the two thioisocyanates, as shown in Table 2. No template effect is observed: products 12a and 12b are not autocatalysts.
Finally, competition experiments of adenosine amine 10 and benzyl amine 11 for the N-methyl imide thioisocyanate 9b (lacking a recognition site) showed a preference for nucleophilic attack by amine 10 (5b:12b = 62:38; c.f. Entry 1 in Table 3 5a:12a = 70:30). Recognition notwithstanding, adenosine amine 10 is the better nucleophile in this reaction system. Taken together, these results show that autocatalysis based on molecular recognition – replication – takes place during the formation of thiourea compound 5a, albeit to a modest extent.
ORGANOCATALYSIS
The solvents and temperatures that favor hydrogen bonding between molecules are essential for the recognition involved in self-replication and these conditions are also typical for organocatalysis by thioureas. There is a subtle difference in the two applications: The kinetics of autocatalytic reactions of the sort discussed above are often seen to obey the “square root law”.16 Since the product (i.e. catalyst) is to varying degrees a hydrogen-bonded dimer, the rate enhancements are proportional to the concentration of the free monomer, a value related to the square root of the total concentration. The dimers are generally not autocatalysts – and product inhibition is inevitable – but the dimers can be organocatalysts if the participating functional groups are exposed to the reagents undergoing change.
There is evidence that 5a is highly dimerized at the low temperatures of the replication experiments, and hydrogen bonding may occur between the very thioureas that are required for organocatalysis as shown in 12 (Figure 4). This hydrogen bonding still leaves an open thiourea site that could even be more activated in H-bonding catalysis than the corresponding monomer,17 but will most likely suffer from steric hindrance due to the congested environment in which it resides. Since there is no requirement that the two functions – catalysis and autocatalysis – need to be optimal, or even operational, under the same conditions, the higher temperatures more appropriate for the organocatalytic applications were used. The thiourea site in the self-replicating molecule 5a is capable of acting as a catalyst through hydrogen bonding to the transition state of various reactions such as cycloadditions,18 condensations,19 hydrocyanations,20 Strecker,21 and Mannich22 reactions, especially in asymmetric settings.23 The acidity of the thiourea is of paramount importance to the rate acceleration; electron-withdrawing groups adjacent to the thiourea function are known to enhance its hydrogen donor ability and consequently its catalytic activity.23 The nitro group was inserted into the biphenyl structure for just this reason. Three reactions were studied to assess the organocatalytic activity of the thiourea function in 5a: Michael additions, Friedel-Crafts alkylation, and a hydrogen transfer reaction. The results of the organocatalytic experiments are reported in percent yield after a constant time for each reaction discussed.
The organocatalytic activity of 5a was demonstrated in Michael additions using 1-heptanethiol 13 and 2,4-pentanedione 14 as nucleophiles. Compound 5a was an effective catalyst for the addition of 1-heptanethiol 13 to nitrostyrene 15 and gave the adduct 16 in 85% yield (Figure 5). Control experiments were performed to evaluate the organocatalytic activity of other functional groups embedded in 5a such as the imide group and the heterocycles of the adenosine. Because Michael additions are known to be catalyzed by bases, we used the adenosine alcohol 17 as a control instead of amine 10. Catalytic amounts of 17 gave a 35% yield of product 16 under the same conditions. Thioisocyanate 9a was also used as a control and gave only 12% product. While these results show that the catalytic activity of 5a is not exclusive to the thiourea function, it is certainly the dominant factor for catalysis.
Additional experimentation indicated that the organocatalytic ability of 5a is decreased by its dimerization (see Figure 4) under these conditions. N-methylated thiourea 5b, which is unable to dimerize, showed higher organocatalytic ability (Figure 6, purple points vs. red points). The combination of thioisocyanate 9a and aminoadenosine 10 showed the same activity as thiourea 5a, illustrating that the thiourea formation is much faster than the thiol addition.
Compound 5a was tested as a catalyst in another Michael reaction. The addition of 2,4-pentanedione 14 to nitrostyrene 18 as catalyzed by 5a gave 19 in 63% yield (Figure 6). However, the same product was observed with the amine 10 (42% yield) and even with the isothiocyanate 9a (45% yield). Apparently, a thiourea is not required for catalysis of this reaction, and what little organocatalytic activity this function shows in 5a must be decreased through its dimerization to 12. The n-methyl imide 5b showed, again, the best activity (Figure 6; compare the purple and red data points), while the combination of thioisocyanate 9a and aminoadenosine 10 showed the same activity as thiourea 5a.
Finally, the organocatalytic activity of 5a was demonstrated in transfer hydrogenation using the Hantzsch ester 20 as the hydride source. Trans-β-nitrostyrene 15 was reduced in high yield (80% of 21) at 35 °C using 5a (Figure 7). In the absence of 5a only a small amount of reduction was observed (14% of 21). The yields with the thioisocyanate 9a (28%), the amine 10 (47%) and alcohol 17 (29%) showed that other functional groups do participate in this reaction. The imide of the Kemp substructure can catalyze the reduction in an estimated 14% yield after 6 days (28%-14% = 14%) and the adenosine group can do likewise in about 15% yield (29%-14% = 15%). From these estimates, the organocatalytic activity of the thiourea group 5a accounts for about 51% [80%-(14%+15%)=51%] of the product. As in the other reactions, dimerization of 5a decreases its organocatalytic activity (Figure 7; compare purple and red data points), and the combination of thioisocyanate 9a and aminoadenosine 10 shows activity indistinguishable from the intact thiourea 5a.
CONCLUSION
The new synthetic compound 5a shows the earmarks of a self-replicating system: recognition-based autocatalysis. This was established through competition experiments with structurally related compounds lacking the recognition features (hydrogen bonding sites). The self-replicator, however, showed only modest organocatalytic activity in all three reactions examined. Its tendency to form a dimeric structure in organic media is held responsible for this shortcoming, since the n-methylated thiourea 5b, which is unable to dimerize, consistently showed higher activity as an organocatalyst. In the future, one way of enhancing catalytic action could be to introduce complementary functions that compete for the hydrogen-bonding sites into the substrates of the reaction. For example, appropriate placement of a purine nucleus of adenine on either partner of the Michael addition reaction should promote catalysis by 5a, and could introduce selectivity into the process. For the present, we suggest that molecules such as 5a provide capabilities of replication and chemical catalysis as minimalist vehicles of “genetics” and “metabolism”, respectively. These functions are widely acknowledged24 as required for molecules nearer to the origin of life than, say, RNA. As for the distant past, the potential precursors to the RNA world are shrouded in mystery but new candidates are emerging from chemical synthesis.25,26 The search is expanding as even water is no longer regarded as a required solvent.27
EXPERIMENTAL SECTION
Aniline 8a: Acyl chloride 6 (280 mg, 0.819 mmol), 3-nitro-4,4’diaminobiphenyl 7 (209 mg, 0.901 mmol), and a catalytic amount of DMAP (10 mg) were added to a 50 mL round bottom flask equipped with a reflux condenser and a stir bar. The reaction was placed under an atmosphere of argon and 30 mL of dry pyridine was added. The reaction mixture was heated to 90 °C for 15 h. The reaction mixture was then cooled to room temperature and the solvent was removed under reduced pressure. The residue was purified using column chromatography eluting with CH2Cl2 and a gradient of EtOAc to a final mixture of 5% EtOAc in CH2Cl2 giving a red solid. Yield 302 mg (68%). 1H NMR (600 MHz, CDCl3) δ 7.77 (s, 1H), 7.43 (d, J = 8.4 Hz, 2H), 7.28 (s, 1H), 7.16 (d, J = 8.4 Hz, 2H), 7.11 (d, J = 8.4 Hz, 1H), 7.08 (d, J = 2.3 Hz, 1H), 6.83 (d, J = 8.4 Hz, 1H), 4.03 (s, 2H), 2.59 (d, J = 14.3 Hz, 2H), 2.22 (d, J = 13.2 Hz, 1H), 2.01 – 1.92 (m, 1H), 1.50 (m, 2H), 1.42 – 1.19 (m, 12H), 0.94 (t, J = 7.1 Hz, 6H), 0.86 (t, J = 7.2 Hz, 3H); 13C NMR (151 MHz, CDCl3) δ 176.3, 171.4, 149.8, 146.7, 136.6, 134.2, 132.8, 128.7, 125.4, 121.1, 118.7, 109.8, 48.3, 46.5, 43.3, 43.2, 40.2, 37.8, 17.2, 17.1, 14.7, 14.5; HRMS (MALDI-FTMS: MH+) calcd. for C30H38N4O5+ 535.2915, found 535.2911.
Thioisocyanate 9a: Aniline 8a (152 mg, 0.280 mmol) was added to a 100 mL round bottom flask and dissolved in 20 mL CH2Cl2. A saturated aqueous solution of sodium bicarbonate (20 mL) was added and the biphasic mixture was cooled to 0 °C in an ice bath. Thiophosgene (327 mg, 2.80 mmol) was added dropwise to the vigorously stirred mixture. The reaction mixture was then allowed to warm to room temperature for 1 h. The organic phase was separated and concentrated under reduced pressure. The yellow residue was purified using column chromatography eluting with CH2Cl2 giving a yellow solid. Yield 90 mg (55 %). 1H NMR (600 MHz, CDCl3) δ 7.67 (d, J = 2.0 Hz, 1H), 7.56 (s, 1H), 7.51 (d, J = 8.2 Hz, 2H), 7.45 – 7.39 (m, 2H), 7.24 (s, 1H), 7.22 (d, J = 8.4 Hz, 2H), 2.58 (d, J = 14.2 Hz, 2H), 2.23 (d, J = 13.2 Hz, 1H), 1.97 (td, J = 4.3, 13.2 Hz, 1H), 1.54 – 1.48 (m, 2H), 1.44 – 1.22 (m, 12H), 0.95 (t, J = 7.1 Hz, 6H), 0.87 (t, J = 7.2 Hz, 3H); 13C NMR (151 MHz, CDCl3) δ 176.2, 171.5, 149.5, 139.7, 137.8, 134.5, 133.3, 132.3, 131.8, 129.3, 128.6, 121.3, 121.2, 48.2, 46.6, 43.3, 43.2, 40.2, 37.8, 17.2, 17.1, 14.7, 14.5; HRMS (MALDI-FTMS: MH+) calcd. for C31H36N4O5S+ 577.2479, found 577.2474.
General procedure for coupling reaction of thioisocyanate 9a or 9b with adenosine-derived amine 10 or benzylamine 11: Thioisocyanate 9a or 9b (1.0 equiv.) was treated with a solution of adenosine-derived amine 10 or benzylamine 11 (1.2 equiv.) in CH2Cl2 (8.0 mM) at -78 °C. After 24 h of stirring at -78 °C, the reaction mixture was concentrated in vacuo. The residue was purified by column chromatography on silica gel, eluting with 20% acetone in chloroform to afford the thiourea.
Thiourea 9a: 1H NMR (600 MHz, DMSO-d6) δ 10.36 (s, 1H), 9.97 (s, 1H), 9.25 (s, 1H), 8.36 (s, 1H), 8.30 – 8.12 (m, 3H), 7.70 (d, J = 2.1, 8.4 Hz, 1H), 7.52 (d, J = 8.6 Hz, 2H), 7.44 (d, J = 8.4 Hz, 1H), 7.35 (s, 2H), 7.21 (d, J = 8.6 Hz, 2H), 6.20 (d, J = 2.6 Hz, 1H), 5.51 (dd, J = 2.6, 6.3 Hz, 1H), 5.09 (dd, J = 3.4, 6.3 Hz, 1H), 4.42 (td, J = 3.4, 6.9 Hz, 1H), 3.98 – 3.90 (m, 1H), 3.82 – 3.67 (m, 1H), 2.61 (d, J = 13.8 Hz, 2H), 2.02 (d, J = 12.6 Hz, 1H), 1.83 – 1.71 (m, 2H), 1.55 (s, 3H), 1.53 – 1.44 (m, 2H), 1.43 (s, 3H), 1.40 – 1.19 (m, 7H), 1.19 – 1.03 (m, 4H), 0.87 (t, J = 7.2 Hz, 6H), 0.79 (t, J = 7.3 Hz, 3H); 13C NMR (151 MHz, DMSO-d6) δ 180.9, 176.2, 172.1, 156.2, 152.8, 148.8, 148.3, 140.0, 139.5, 138.7, 131.7, 131.5, 129.7, 127.6, 126.0, 121.8, 119.2, 117.1, 113.5, 88.9, 83.7, 83.1, 81.7, 46.7, 45.7, 45.6, 42.2, 41.6, 40.1, 36.9, 27.0, 25.2, 16.5, 16.4, 14.7, 14.4; HRMS (MALDI-FTMS: MH+) calcd. for C44H54N10O8S+ 883.3919, found 883.3922.
Thiourea 9b: 1H NMR (600 MHz, DMSO-d6) δ 9.98 (s, 1H), 9.21 (s, 1H), 8.36 (s, 1H), 8.29 – 8.14 (m, 3H), 7.69 (d, J = 8.3 Hz, 1H), 7.58 (d, J = 8.6 Hz, 2H), 7.45 (d, J = 8.3 Hz, 1H), 7.35 (s, 2H), 7.21 (d, J = 8.6 Hz, 2H), 6.20 (d, J = 2.5 Hz, 1H), 5.50 (dd, J = 2.5, 6.2 Hz, 1H), 5.09 (dd, J = 3.2, 6.2 Hz, 1H), 4.42 (td, J = 3.2, 6.6 Hz, 1H), 3.98 – 3.90 (m, 1H), 3.80 – 3.71 (m, 1H), 2.63 (s, 3H), 2.61 (d, J = 14.0 Hz, 2H), 2.03 (d, J = 12.8 Hz, 1H), 1.91 – 1.74 (m, 2H), 1.55 (s, 3H), 1.53 – 1.46 (m, 2H), 1.41 – 1.27 (m, 7H), 1.34 (s, 3H), 1.14 – 1.08 (m, 4H), 0.87 (t, J = 7.1 Hz, 6H), 0.78 (t, J = 7.3 Hz, 3H); 13C NMR (151 MHz, DMSO-d6) δ 180.9, 176.2, 172.1, 156.2, 152.8, 148.8, 148.3, 140.0, 139.5, 138.7, 131.7, 131.5, 129.7, 127.6, 126.0, 121.8, 119.2, 117.1, 113.5, 88.9, 83.7, 83.1, 81.7, 46.7, 45.7, 45.6, 42.2, 41.6, 40.1, 36.9, 27.0, 25.2, 16.5, 16.4, 14.7, 14.4; HRMS (MALDI-FTMS: MH+) calcd. for C45H56N10O8S+ 897.4076, found 897.4073.
General procedure of competition studies in the presence of additive for coupling reaction of adenosine derived amine 10 or 11 with thioisocyanate 9a and 9b:
Method A: Adenosine derived amine 10 (8.0 mM) or benzylamine 11 (8.0 mM) in CH2Cl2 was added very slowly to a solution of thioisocyanate 9a and 9b (8.0 mM), and additive 5a in CH2Cl2 at -78 °C. After 24 h of stirring at -78 °C, the mixture was analyzed by HPLC using the peak areas [254 nm].
Method B: Thioisocyanate 9a or 9b (8.0 mM) was added very slowly to a solution of amino adenosine 10 (8.0 mM), and benzylamine 11 (8.0 mM), and thiourea 5a (0.00 equiv, 0.25 equiv, 0.50 equiv) in CH2Cl2 at -78 °C. After 24 h of stirring at -78 °C, the mixture was analyzed by HPLC using the peak areas [254 nm].
ACKNOWLEDGEMENTS
We are grateful to the Skaggs Institute for support and Prof. A. Eschenmoser for advice and encouragement. S. K. and R. H. are Skaggs Post-doctoral Fellows and A. C. S. is a Skaggs Pre-doctoral Fellow. Dedicated to Professor Albert Eschenmoser with admiration and gratitude.
References
1. D. S. Kemp and K. S. Petrakis, J. Org. Chem., 1981, 46, 5140. CrossRef
2. J. Rebek, Jr., Science, 1987, 235, 1478. CrossRef
3. M. M. Conn, E. A. Wintner, and J. Rebek, Jr., Acc. Chem. Res., 1994, 27, 198. CrossRef
4. T. Tjivikua, P. Ballester, and J. Rebek Jr., J. Am. Chem. Soc., 1990, 112, 1249. CrossRef
5. D. N. Reinhoudt, D. M. Rudkevich, and F. de Jong, J. Am. Chem. Soc., 1996, 118, 6880. CrossRef
6. G. A von Kiedrowski, Angew. Chem., Int. Ed. Engl., 1986, 25, 932.
7. N. Paul and G. F. Joyce, Proc. Natl. Acad. Sci. USA, 2002, 99, 12733. CrossRef
8. W. Gilbert, Nature, 1986, 319, 618. CrossRef
9. A. Vidonne and D. Philp, Eur. J. Org. Chem., 2009, 5, 583. CrossRef
10. K. Severin, D. H. Lee, A. J. Kennan, and M. R. Ghadiri, Nature, 1997, 389, 706. CrossRef
11. M. Mauksch, S. B. Tsogoeva, I. M. Martynova, and S. Wei, Angew. Chem. Int. Ed., 2007, 46, 393. CrossRef
12. A. Eschenmoser, Tetrahedron, 2007, 63, 12821. CrossRef
13. S. Kamioka, D. Ajami, and J. Rebek Jr., Proc. Nat. Acad. Sci. USA, 2009, 107, 541. CrossRef
14. S. Kamioka, D. Ajami, and J. Rebek, Jr., Chem. Comm., 2009, 7324. CrossRef
15. V. Rotello, J. I. Hong, and J. Rebek Jr., J. Am. Chem. Soc., 1991, 113, 9422. CrossRef
16. G. von Kiedrowski, B. Wlotzka, J. Helbing, M. Matzen, and S. Jordan, Angew. Chem., Int. Ed. Engl., 1991, 30, 423. CrossRef
17. C. R. Jones, G. Dan Pantos¸ A. J. Morrison, and M. D. Smith, Angew. Chem. Int. Ed., 2009, 48, 7391. CrossRef
18. A. Wittkopp and P. Schreiner, Chem. Eur. J., 2003, 9, 407. CrossRef
19. X. Li, H. Deng, S. Luo, and J.-P. Cheng, Eur. J. Org. Chem., 2008, 4350. CrossRef
20. A. G. Wenzel, M. P. Lalonde, and E. N. Jacobsen, Synlett, 2003, 12, 1919. CrossRef
21. M. Sigman, P. Vachal, and E. Jacobsen, Angew. Chem. Int. Ed., 2000, 39, 1279. CrossRef
22. A. G. Wenzel and E. N. Jacobsen, J. Am. Chem. Soc., 2002, 124, 12964. CrossRef
23. M. S. Taylor and E. N. Jacobsen, Angew Chem. Int. Ed., 2006, 45, 1520; CrossRef A. G. Doyle and E. N. Jacobsen, Chem. Rev., 2007, 107, 5713. CrossRef
24. “The Limits of Life in Planetary Systems” The National Academies Press, Washington, DC, 2007, Ch. 5: Origins of Life, pp. 53-68.
25. G. K. Mittapalli, K. R. Reddy, H. Xiong, O. Munoz, B. Han, F. De Riccardis, R. Krishnamurthy, and A. Eschenmoser, Angew. Chem. Int. Ed., 2007, 46, 2470. CrossRef
26. G. K. Mittapalli, Y. M. Osornio, M. A. Guerrero, K. R. Reddy, R. Krishnamurthy, and A. Eschenmoser, Angew. Chem. Int. Ed., 2007, 46, 2478. CrossRef
27. C. F. Barbas, III, Angew. Chem. Int. Ed., 2008, 47, 42 CrossRef