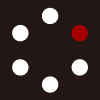
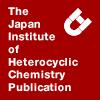
HETEROCYCLES
An International Journal for Reviews and Communications in Heterocyclic ChemistryWeb Edition ISSN: 1881-0942
Published online by The Japan Institute of Heterocyclic Chemistry
e-Journal
Full Text HTML
Received, 17th May, 2010, Accepted, 22nd June, 2010, Published online, 23rd June, 2010.
DOI: 10.3987/COM-10-11978
■ Efficient Synthesis of 2’-O-Cyanoethylated Imidazole C-Ribonucleoside Phosphoramidite: A Practical Building Block for Probing the Catalytic Mechanism of Ribozymes
Lisa Araki, Zheng-yun Zhao, David M. J. Lilley, and Shinya Harusawa*
Osaka University of Pharmaceutical Sciences, 4-20-1 Nasahara, Takatsuki, Osaka 569-1094, Japan
Abstract
A high-yielding synthetic route leading to new 2’-O-cyanoethylated imidazole C-ribonucleoside phosphoramidite (PA) 1 has been developed (41% overall yield in ten steps). This has resulted in a marked improvement of yield compared to unstable 2’-O-TBDMS PA 2. The new synthon PA 1 enables the imidazole moiety to be incorporated into any RNA sequence in principle, and has been used as a practical building block for probing the catalytic mechanism of a ribozyme.INTRODUCTION
RNA catalysis can be used in cells for the processing of RNA molecules, the control of gene expression and even the synthesis of proteins.1 The nucleolytic ribozymes catalyse the site-specific cleavage and ligation of RNA in order to process replication intermediates, or cleave a mRNA species. Current evidence points to the use of general acid-base catalysis by this class of ribozymes to accelerate these trans esterification reactions, but the mechanisms are not fully established. We have developed the use of a nucleoside with imidazole base replacing the conventional nucleobases as a powerful tool to probe general acid-base catalysis in the active sites of these ribozymes, and applied this to the Varkud satellite2e,3 and hairpin ribozymes.4 Evidence suggests that both ribozymes employ adenine and guanine nucleobases in general acid-base catalysis.
In the course of these studies, we have recently reported the synthesis of a 2’-O-tert-butyldimethylsilyl (TBDMS) C4-linked imidazole ribonucleoside phosphoramidite 23 starting from tribenzyl D-ribose 4,5 as shown in Scheme 1. In this study, pivaloyloxymethyl (POM) was introduced as an effective protecting group for the imidazole-N that can be readily removed under mild basic conditions [28% aq NH3-EtOH (1:3, v/v)], which is compatible with 2’-O-TBDMS chemistry. Phosphoramidite (PA) 2 can be applied as a key building block to investigate the role of general acid-base catalysis in the ribozyme situation, since imidazole with a pKa of 7.1 functions as both a good donor and acceptor of protons. Enzymes such as ribonuclease A use the imidazole side chain of histidine in general acid-base catalysis of RNA cleavage. We demonstrated that the ribonucleoside variant 2 could be introduced into VS ribozyme RNA using solid-phase t-BDMS chemistry implemented on an automated synthesizer. We thus generated a modified VS ribozyme (A756 Imz), in which the nucleobase of A756 was replaced by imidazole (Figure 1).2e The A756 Imz resulted in excellent extents of cleavage and ligation at the correct position of natural VS ribozyme, consistent with a role of the nucleobase of A756 in general acid-base catalysis.2e PA 2 was also used to replace G8 in the hairpin ribozyme with the imidazole base.4 The modified hairpin ribozyme (G8Imz) catalyzed both cleavage and ligation reactions, with a pH dependence consistent with a role of G8 in general acid-base catalysis.4
Using 2 an imidazole nucleoside can in principle be incorporated at any site into RNA sequence through chemical synthesis. Incorporation during synthesis is superior to the use of high concentrations of exogenous imidazole in the medium,6 because access to the active site can be prevented by steric blockage. However, there is a serious hindrance to the general application of this approach. As shown in Scheme 1, the synthetic route of PA 2 lacks the selective introduction of t-BDMS group at 2’-hydroxy group of a diol intermediate 7 owing to easy 2’-3’ t-BDMS-migration, leading to the inseparable 1:1 mixture 8ab. Thus, when the mixture 8ab was subjected to phosphitylation by heating at 40 °C for 15 h in 1,2-dichloroethane, PA 2 was only obtained in poor yield (31-49%) together with 2’-PA 9 (25%). Moreover, a great care has to be taken into for purification and isolation of 2 because of its acid-labile character, and contamination by phosphorus residues. For example, the required fractions have to be separated from hydrolysed excess phosphitylating reagent and isomer 9 in the mixture obtained after phosphitylation reaction. The overall yield of 2 is < 5% at most through 8 steps from the starting tribenzyl D-ribose 4. As a result, it is rather difficult to obtain sufficient quantities (at least 50 mg) of PA 2 for conventional solid-phase RNA oligonucleotide synthesis. If a novel imidazole-building block can be efficiently designed and synthesized, it would lead to a more practical pathway to this chemo-genetic approach to investigate RNA functions.
In our systematic studies on the catalytic mechanism of VS ribozyme,2-4 we have reported an efficient synthesis of novel 2’-O-cyanoethylated C4-linked C2-imidazole ribonucleoside PA 3 with two-carbon linker between imidazole and ribose moiety.7 The modified VS ribozyme (G638C2Imz) synthesized from 3 supported a direct role for the guanine base at position 638 in the catalytic mechanism of the VS ribozyme.2a In this study, the cyanoethyl (CE) group, which was developed by Sekine and co-workers,8 was used as the smallest available protecting group for the 2’-hydroxy function. It is particularly significant that a combination of cyanoethyl (CE) and POM protecting groups inhibited 2’-3’ migration between 2’- and 3’-hydroxy groups and provided additional stability for purification of PA 3. Therefore, our attention was directed to the use of CE and POM groups for a new type of PA 2. In this paper we describe the efficient synthesis of novel 2’-O-CE-C4-linked imidazole ribonucleoside PA 1, that enables the insertion of imidazole at a given position in an RNA sequence.
RESULTS AND DISCUSSION
PA 1 was synthesized in five steps from a previous triol precursor 63a,3b as shown in Scheme 2. 3’,5’-O-TIPS-protection (TIPS= 1,1,3,3-tetraisopropyldisiloxanediyl) of 6 gave a compound 103b (73%) to facilitate the selective introduction of a 2’-hydroxy protecting group. Cyanoethylation8 of 10 successfully afforded a fully-protected intermediate 11 in high yield (91%). The TIPS group of 11 was selectively removed by treatment with Et3N・3HF to give the 3’,5’-O-unprotected ribonucleoside derivative 12 (quant). It should be noted that the imN-POM group remains intact under the basic conditions that exist during cyanoethylation and the fluoride deprotection of TIPS group. After DM-tritylation of 12 (98%) in the usual manner, 3’-free derivative 13 was finally phosphitylated. Treatment of 13 with 2-cyanoethyl N,N,N’,N’-tetraisopropylphosphordiamidite in the presence of 4,5-dicyanoimidazole (DCI) proceeded smoothly at room temperature (rt) for 1h in dichloroethane to give the final 2’-CE-PA 1. Purification of the crude product was easily achieved by basic silica gel column chromatography to yield PA 1 (78%) as a white foam, in contrast to the more time-consuming purification of 2’-O-t-BDMS-PA 2. The total yield of 1 was 41% in ten steps from the commercially-available tribenzyl D-ribose 4, demonstrating the effectiveness of this approach. 31P-NMR of 1 revealed two P-diastereomers at δ 149.5 and 149.7 ppm (CDCl3). We recently reported MS measurement of nucleoside and non-nucleoside phosphoramidites, with the new matrix system [triethanolamine (TEOA)-NaCl] on LSIMS or FABMS using a double-focusing mass spectrometer.9 The present method was consistent with the composition formula {C47H60N5O9PNa [(M+Na)+]; calculated: 892.4027, observed: 892.4025}. To assess the stability of the PA 1 against CH3CN used in the automated RNA synthesizer, CD3CN solutions of 1 were allowed to stand at rt in an NMR tube. After one week, 1H, 13C, and 31P NMR measurements of the solution did not reveal any significant decomposition, indicating that compound 1 is stable in CH3CN. Furthermore, PA 1 could be stored in vials at rt for at least one month without significant spectral change.
We have demonstrated an efficient synthesis of the stable compound 2’-O-CE PA 1 with an overall yield of 41% in ten steps beginning from the starting material 4. We see no reason why the synthesis could not be scaled up to meet the needs of RNA studies. Using PA 1 the imidazole nucleoside can be incorporated at any site in a DNA or RNA sequence through chemical synthesis, hence, it promises to open a more practical pathway to this chemo-genetic strategy for investigating ribozyme mechanism as well as other RNA functions. Studies of modified VS ribozyme and other imidazole oligonucleotides are under way, and will be published in due course.
EXPERIMENTAL
Melting points have been determined using a hot-stage apparatus; these data are uncorrected. IR spectra were recorded on Shimadzu IR-435 spectrometer. 1H- and 13C- spectra were recorded using tetramethylsilane as the internal standard on Varian Mercury-300 or Varian UNITY INOVA-500 spectrometers. 31P-NMR spectra were recorded at 121 MHz (Varian UNITY INOVA-500) and the chemical shifts were measured using 85% H3PO4 as an external standard. Fast atom bombardment mass spectrometric studies (FAB-MS) were performed using a JMS-700 (JEOL Ltd.) spectrometer in the positive ion mode, with TEOA-NaCl or 3-nitrobenzylalcohol (NBA) as matrix. Reactions with air- and moisture-sensitive compounds were carried out under an argon atmosphere. Unless otherwise noted, all extracts were dried over Na2SO4, and the solvents were removed using a rotary evaporator under reduced pressure. Anhydrous solvents were purchased from Wako Chemical Co. and Nacalai Chemical Co. Fuji Silysia FL-60D silica gel, Fuji Silysia BW-127ZH silica gel, and Merck 60F254 were used for flash column chromatography, column chromatography and thin-layer chromatography (TLC), respectively. Chromatorex NH-DM 1020 (Fuji Silysia Chemical Ltd.) was used for basic (N-H) silica gel chromatography.
[4-(2-O-CE-3,5-O-TIPDS-β-D-ribofuranosyl)imidazolyl]methyl 2,2-dimethylpropionate (11)
Acrylonitrile (0.27 mL, 4.1 mmol) and cesium carbonate (67.5 mg, 0.2 mmol) were added to the solution of 103b (113 mg, 0.20 mmol) in t-BuOH (1.0 mL). After being vigorously stirred at rt for 4 h, the mixture was filtered through Celite. The solvent was evaporated, and the residue was purified by column chromatography (50 to 100% EtOAc in hexane) to obtain 11 (112 mg, 91%) as an oil; 1H NMR (CDCl3) δ 0.99-1.10 (m, 28H), 1.16 (s, 9H), 2.65 (t, 2H, J = 6.8 Hz), 3.78-4.19 (m, 4H), 3.85 (t, 2H, J = 6.8 Hz), 4.40 (dd, 1H, J = 9.1, 4.4 Hz), 4.94 (s, 1H), 5.75 (dd, 2H, J = 15.7, 10.6 Hz), 7.10 (s, 1H), 7.62 (s, 1H); 13C NMR (CDCl3) δ 13.1, 13.2, 13.5, 13.8, 17.4, 17.5, 17.6, 17.7, 17.8, 17.9, 19.6, 27.1, 38.9, 60.6, 65.6, 67.7, 71.2, 79.9, 80.2, 83.7, 108.9, 116.7, 117.4, 137.8, 142.0, 176.8; IR (film, cm-1) 1735, 2250 (CN); HRMS(FABMS: NBA) calcd for C29H52N3O7Si2 (M+H)+ 610.3343, found 610.3334.
[4-(2-O-CE-β-D-ribofuranosyl)imidazolyl]methyl 2,2-dimethylpropionate (12)
Compound 11 (111 mg, 0.18 mmol) was dissolved in anhydrous THF (2.0 mL). To the solution were added Et3N・3HF (0.11 ml, 0.64 mmol) and Et3N (0.05 mL, 0.33 mmol). After stirring at rt for 2.5 h, the reaction mixture was evaporated. The residue was subjected to chromatography on a silica gel column using MeOH-CHCl3 (0:100 to 3:97 v/v) as eluent to give compound 12 (68 mg, quant) as white foam. 1H NMR (CDCl3) δ 1.18 (s, 9H), 2.56 (td, 2H, J = 5.4, 2.2 Hz), 3.62-3.82 (m, 3H), 3.93 (dd, 1H, J = 12.8, 2.2 Hz), 4.16 (d, 1H, J = 2.2 Hz), 4.34 (dd, 1H, J = 6.3, 4.8 Hz), 4.46 (dd, 1H, J = 4.8, 2.2 Hz), 4.88 (d, 1H, J = 6.3 Hz), 5.80 (s, 2H), 7.12 (s, 1H), 7.71 (s, 1H); 13C NMR (CDCl3) δ 19.4, 27.1, 39.0, 63.5, 65.4, 67.8, 72.1, 76.2, 84.7, 86.4, 117.1, 117.7, 138.3, 140.8, 176.9; IR (film, cm-1) 1735, 2250 (CN), 3300; HRMS(FABMS: NBA) calcd for C17H26N3O6 (M+H)+ 368.1822, found 368.1830.
[4-(2-O-CE-5-O-DMT-β-D-ribofuranosyl)imidazolyl]methyl 2,2-dimethylpropionate (13)
Compound 12 (177 mg, 0.48 mmol) was co-evaporated three times with pyridine (2 mL) and redissolved in dry pyridine (2.5 mL). DMTCl (257 mg, 0.72 mmol), Et3N (0.10 mL, 0.72 mmol), and DMAP (1.5 mg, 0.01 mmol) were added to the solution, and the mixture stirred at rt for 3h. MeOH (1 mL) was added and the mixture stirred for further 5 min. The solvents were removed to leave a residue that was purified by the column chromatography (NH-silica gel) with CHCl3-benzene (0:100, 15:85, 70:30, to 100:0 v/v) as eluent to give the white foam of compound 13 (316 mg, 98%). 1H NMR (CDCl3) δ 1.14 (s, 9H), 2.65 (t, 2H, J = 6.4 Hz), 3.30 (dd, 1H, J = 9.9, 4.6 Hz), 3.39 (dd, 1H, J = 9.9, 3.9 Hz), 3.77 (s, 6H), 3.80-3.98 (m, 2H), 4.05 (m, 1H), 4.17 (m, 2H), 4.97 (d, 1H, J = 4.6 Hz), 5.65 (d, 1H, J = 10.7 Hz), 5.73 (d, 1H, J = 10.7 Hz), 6.81 (d, 4H, J = 8.5 Hz), 7.10 (s, 1H), 7.14-7.49 (m, 9H), 7.66 (s, 1H); 13C NMR (CDCl3) δ 19.4, 27.1, 38.9, 55.3, 63.9, 65.1, 67.7, 71.6, 78.0, 82.5, 83.1, 86.0, 112.8, 117.4, 126.3, 127.3, 127.9, 129.7, 129.8, 135.5, 135.6, 137.7, 141.0, 144.4, 157.9, 176.8; IR (film, cm-1) 1605, 1735, 2250 (CN), 3300; HRMS(FABMS: NBA) calcd for C38H44N3O8 (M+H)+ 670.3129, found 670.3126.
(4-{5-O-DMT-2-O-CE-3-O-[(2-cyanoethoxy)(N,N-diisopropylamino)phosphino]-β-D-ribofuranosyl}-imidazolyl)methyl 2,2-dimethylpropionate (1a and 1b)
Compound 13 (76 mg, 0.11 mmol) was dissolved in dry dichloroethane (1.2 mL) and 4,5-DCI (17.0 mg, 0.14 mmol) and 2-cyanoethyl N,N,N’,N’-tetraisopropylphosphordiamidite (0.07 ml, 0.23 mmol) were added. The resulting mixture was stirred at 40°C for 1 h and then evaporated. The residual oil was subjected to chromatography on NH-silica gel with the solvent system; 30%, 50%, and 80% EtOAc in hexane, to give a ca. 1:1 mixture of diastereomers 1a and 1b (77 mg, 78%) as a white foam. Although the separation of 1a and 1b was not required for the following oligonucleotide synthesis, they could be partially resolved by the use of the above solvent system. Compound 1a (more polar): white foam; Rf = 0.42 (EtOAc); 1H NMR (CDCl3) δ 0.99 [s, 3H, -CH(CH3)CH3], 1.01 [s, 3H, -CH(CH3)CH3], 1.09-1.16 [overlapped peaks, 17H, -C(CH3)3, -CH(CH3)CH3 , -CH(CH3)CH3 x 2], 2.59 (m, 4H, -POCH2CH2CN, -OCH2CH2CN), 3.18 (dd, 1H, J = 9.6, 4.8 Hz, 5’-H), 3.38 (dd, 1H, J = 9.6, 3.8 Hz, 5’-H), 3.54-3.96 (m, 4H, -POCH2CH2CN, -OCH2CH2CN), 3.77 (s, 6H, -OCH3 x 2), 4.20 (m, 2H, 2’-H, 4’-H), 4.33 (dt, 1H, J = 11.2, 4.8 Hz, 3’-H), 4.96 (d, 1H, J = 5.7 Hz, 1’-H), 5.65 [d, 1H, J = 10.9 Hz, -CHHOCO(CH3)3], 5.74 [d, 1H, J = 10.9 Hz, -CHHOCO(CH3)3 ], 6.78 (d, 4H, J = 8.2 Hz, Ar-H x 4), 7.11 (s, 1H, Im-H), 7.14-7.29 (m, 5H, Ar-H x 5), 7.34 (dd, 2H, J = 8.2, 2.7 Hz, Ar-H x 2), 7.46 (d, 2H, J = 7.1 Hz, Ar-H x 2), 7.61 (s, 1H, Im-H); 13C NMR (CDCl3) δ 19.3, 20.7, 20.8, 24.8, 24.9, 27.1, 38.9, 43.2, 43.4, 55.3, 58.4, 58.7, 63.8, 65.3, 67.8, 72.4, 72.6, 77.6, 82.2, 82.8, 86.1, 112.7, 117.5, 117.8, 126.3, 127.3, 128.0, 129.7, 129.8, 135.5, 137.7, 141.0, 144.3, 157.8, 176.8; 31P NMR (CDCl3) δ 149.5. Compound 1b (less polar): white foam; Rf = 0.52 (EtOAc); 1H NMR (CDCl3) δ 1.10-1.19 [overlapped peaks, 23H, -C(CH3)3, -CH(CH3)CH3 x 2], 2.33 (t, 2H, J = 6.1 Hz, -POCH2CH2CN), 2.57 (t, 2H, J = 6.1 Hz, -OCH2CH2CN), 3.21 (dd, 1H, J = 10.6, 4.7 Hz, 5’-H), 3.44 (dd, 1H, J = 10.6, 4.2 Hz, 5’-H), 3.50-3.67 (m, 2H, -POCH2CH2CN), 3.67-3.90 (m, 2H, -OCH2CH2CN ), 3.78 (s, 6H, -OCH3 x 2), 4.16 (t, 1H, J = 5.2 Hz, 2’-H), 4.24 (q, 1H, J = 4.7 Hz, 4’-H), 4.39 (dt, 1H, J = 10.6, 5.2 Hz, 3’-H), 4.96 (d, 1H, J = 5.2 Hz, 1’-H), 5.64 [d, 1H, J = 11.1 Hz, -CHHOCO(CH3)3 ], 5.74 [d, 1H, J = 11.1 Hz, -CHHOCO(CH3)3], 6.82 (d, 4H, J = 9.2 Hz, Ar-H x 2), 7.14 (s, 1H, Im-H), 7.14-7.30 (m, 5H, Ar-H x 5), 7.36 (dd, 2H, J = 9.2, 2.1 Hz, Ar-H x 2), 7.48 (d, 2H, J = 7.9 Hz, Ar-H x 2), 7.62 (d, 1H, J = 1.3 Hz, Im-H), 13C NMR (CDCl3) δ 19.2, 20.4, 20.5, 24.9, 25.0, 27.1, 38.9, 43.4, 43.6, 55.3, 58.0, 58.2, 63.4, 65.1, 67.8, 72.5, 72.7, 77.5, 82.1, 82.5, 86.0, 108.9, 112.7, 117.1, 117.8, 126.4, 127.3, 128.0, 129.9, 135.6, 137.7, 141.1, 144.4, 157.8, 176.8; 31P NMR (CDCl3) δ 149.7; HRMS (FABMS: TEOA+NaCl)9 of diastereomers 1ab: calcd for C47H60N5O9P+Na [(M+Na)+] 892.4027, found 892.4025.
ACKNOWLEDGEMENTS
We are grateful to Dr. K. Minoura and Ms. M. Fujitake at Osaka University of Pharmaceutical Sciences for NMR and MS measurements. This work was supported in part by Grant-in-Aid for Scientific Research [Grant No.21590130 (to S.H.)] from JSPS and Grant-in-Aid for “High Technology Research Center” Project for Private Universities: matching subsidiary fund from MEXT (Ministry of Education, Sciences, Sports and Culture), 2007-2009, Japan. We also acknowledge the financial support of Cancer Research UK.
References
1. D. M. J. Lilley and F. Eckstein, ‘Ribozymes and RNA Catalysis,’ Royal Society of Chemistry, Cambridge, 2008.
2. (a) T. J. Wilson, A. C. McLeod, and D. M. J. Lilley, EMBO J., 2007, 26, 2489; CrossRef (b) D. A. Lafontaine, T. J. Wilson, D. G. Norman, and D. M. J. Lilley, J. Mol. Biol., 2001, 312, 663; CrossRef (c) D. A. Lafontaine, T. J. Wilson, Z. Zhao, and D. M. J. Lilley, J. Mol. Biol., 2002, 323, 23; CrossRef (d) J. Lipfert, J. Ouellet, D. G. Norman, S. Doniach, and D. M. J. Lilley, Structure, 2008, 16, 1357; CrossRef (e) Z. Zhao, A. McLeod, S. Harusawa, L. Araki, M. Yamaguchi, T. Kurihara, and D. M. J. Lilley, J. Am. Chem. Soc., 2005, 127, 5026. CrossRef
3. (a) L. Araki, S. Harusawa, M. Yamaguchi, S.Yonezawa, N. Taniguchi, D. M. J. Lilley, Z. Zhao, and T. Kurihara, Tetrahedron Lett., 2004, 45, 2657; CrossRef (b) L. Araki, S. Harusawa, M. Yamaguchi, S. Yonezawa, N. Taniguchi, D. M. J. Lilley, Z. Zhao, and T. Kurihara, Tetrahedron, 2005, 61, 11976; CrossRef (c) We reported in error a singlet-like peak [δ 148.9] for the two P-diastereomers of PA 2 in ref. 3a and 3b, but the two P-diastereomers [δ 148.9, 150.5] of 2 could be resolved by using a basic preparative TLC plate [Chromatorex-NH DM1020HP (pH 10.1) purchased from Fuji Silysia Chemical Ltd].
4. T. J. Wilson, J. Ouellet, Z. Zhao, S. Harusawa, L. Araki, T. Kurihara, and D. M. J. Lilley, RNA, 2006, 12, 980. CrossRef
5. 2,3,5-Tri-O-benzyl-D-ribofuranose 4 was purchased from Carbosynth Ltd.
6. A. T. Perrotta, I. Shih, and M. D. Been, Science, 1999, 286, 123. CrossRef
7. L. Araki, K. Morita, M. Yamaguchi, Z. Zhao, T. J. Wilson, D. M. J. Lilley, and S. Harusawa, J. Org. Chem., 2009, 74, 2350. CrossRef
8. (a) H. Saneyoshi, K. Ando, K. Seio, and M. Sekine, Tetrahedron, 2007, 63, 11195; CrossRef (b) H. Saneyoshi, K. Seio, and M. Sekine, J. Org. Chem., 2005, 70, 10453. CrossRef
9. (a) M. Fujitake, S. Harusawa, L. Araki, M. Yamaguchi, D. M. J. Lilley, Z. Zhao, and T. Kurihara, Tetrahedron, 2005, 61, 4689; CrossRef (b) M. Fujitake, S. Harusawa, Z. Zhao, and T. Kurihara, Bull. Osaka Univ. Pharm. Sci., 2007, 1, 107; (c) S. Harusawa, M. Fujitake, T. Kurihara, Z. Zhao, and D. M. J. Lilley, ‘Current Protocols in Nucleic Acid Chemistry, ed. by S. L. Beaucage, D. E. Bergstrom, P. Herdewijn, and A. Matsuda, John Wiley & Sons,: New York, 2006, pp. 10.11.1-10.11.15.