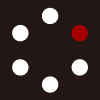
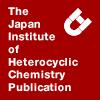
HETEROCYCLES
An International Journal for Reviews and Communications in Heterocyclic ChemistryWeb Edition ISSN: 1881-0942
Published online by The Japan Institute of Heterocyclic Chemistry
e-Journal
Full Text HTML
Received, 23rd July, 2010, Accepted, 3rd September, 2010, Published online, 6th September, 2010.
DOI: 10.3987/COM-10-12027
■ Mild Oxidation of Alcohols Using Soluble Polymer-Supported TEMPO in Combination with Oxone: Effect of a Basic Matrix of TEMPO Derivatives
Kazutsugu Matsumoto,* Toshiaki Iwata, Masahiro Suenaga, Masayuki Okudomi, Masaki Nogawa, Mariko Nakano, Ai Sugahara, Yuta Bannai, and Kenji Baba
Department of Chemistry, Meisei University, Hodokubo 2-1-1, Hino, Tokyo 191-8506, Japan
Abstract
The highly efficient and mild oxidation of alcohols using new soluble polymer-supported TEMPO derivatives in combination with Oxone is disclosed. Two types of the PEG-supported TEMPO derivative were easily synthesized by a simple esterification or Click Chemistry. The oxidation of primary alcohols with the precatalysts, TBAB, and Oxone in CH2Cl2 or BTF proceeded to afford the corresponding oxidized products. As a result of the reactions using several TEMPO derivatives including the nonpolymer-supported and insoluble polymer-supported compounds under the same reaction conditions, the synthesized PEG-supported TEMPO bearing a succinate spacer between the PEG and TEMPO parts was chosen as the best due to its high reactivity and product selectivity. This reaction was also applicable for the oxidation of secondary alcohols. In this system, the isolation of the products was achieved by simple filtration. The PEG-supported TEMPO bearing a succinate spacer was easily recovered and reused at least 10 times without a purification process.
INTRODUCTION
The development of safe and mild oxidation procedures is still an attractive research field, and many oxidizing reagents have been utilized for the transformation of alcohols to carbonyl compounds. In particular, the reaction using a metal-free catalyst, such as 2,2,6,6-tertamethylpiperidinyl-1-oxyl (TEMPO), is preferable for an environment-friendly system. On the other hand, the use of polymer-bound catalysts has emerged because the recoverable and recyclable reagents allow us to realize a sustainable synthesis. In this context, several polymer-bound TEMPO species have also been reported.1 Recently, poly(ethylene glycol) (PEG) has been recognized as an inexpensive and convenient soluble polymer,2-5 and the oxidations of alcohols using a PEG-supported TEMPO have also been developed under homogeneous conditions.6-10 In almost all cases, the reactions were tested in combination with aqueous bleach, which is a strong terminal oxidant, and the troublesome extraction procedure must also be examined. We now disclose a mild and environment-friendly oxidation process of alcohols using a new PEG-supported TEMPO in combination with Oxone (2KHSO5·KHSO4·K2SO4) as a co-oxidant, and the easy isolation of the products was accomplished by simple filtration. We also investigated the effect of a basic matrix of TEMPO derivatives.
RESULTS AND DISCUSSION
The purity of PEG-supported compounds can be determined by a 1H NMR analysis, and the integrated intensities of the protons of a TEMPO part can be compared to that of the PEG-methylenes as the reference. The simplest PEG-supported TEMPO (1, Scheme 1), which has no spacer between the PEG and TEMPO, is commercially available from Aldrich. The average MW of the basic PEG of the commercial 1 was estimated to be 3400 by an ESI-TOFMS analysis, but a 1H NMR analysis of the commercial 1 indicated that the purity was estimated to be ca.50%. Moreover, in many previous cases, the structure of the spacer between the PEG and the functionalized part should be important for the reactivity, selectivity, etc.2-5 We then decided to prepare a new and highly pure PEG-supported TEMPO bearing a spacer by a simple procedure.
For the easy and effective preparation of the PEG-supported TEMPO, we tried to combine PEG with TEMPO as ester bonds through a succinate spacer (Scheme 2). The reaction of 4-hydroxy TEMPO, the free radical 2, and succinic anhydride with DMAP in CH2Cl2 proceeded at room temperature to give the corresponding monocarboxylic acid 3 in an excellent yield. The sequential coupling of 3 with PEG4600-OH (4, av MW 4600) using DMAP and DCC in CH2Cl2 at room temperature afforded the PEG-supported TEMPO 5. The purity was estimated to be over 95% by a 1H NMR analysis. Compound 5 was easily precipitated by dilution with Et2O, and was treated as a solid.
The production of 5 was confirmed by an ESI-TOFMS analysis. Figure 1a shows a part of the mass spectrum obtained for PEG4600-OH (4). Because the spectrum shows the characteristic PEG series of ions with a m/z 22 spacing, the most abundant ion was estimated to be 2301 Da and the molecular mass of 4 was determined to be ca. 4556 Da ([HO(CH2CH2O)103H·2Na]2+; z = +2). On the other hand, the mass spectrum series of compound 5 was shifted to a higher m/z range (Figure 1b). The estimated m/z of 5 is then 2555 Da as the most abundant ion, and the molecular mass was found to be ca. 5064 Da. This result is almost consistent with the calculated mass (5061 Da) from C13H21NO5(CH2CH2O)103C13H21NO4.
On the other hand, a tetra(ethylene glycol)(TEG)-supported TEMPO 7 bearing a shorter chain was also synthesized by the coupling of 3 with TEG-OH (6) using the same procedure mentioned above (Scheme 3). Compound 7 was purified by column chromatography on silica gel, because compound 7 was liquid.
We also synthesized an alternative PEG-supported TEMPO 11 with Click Chemistry as the key step (Scheme 4).11 The mesylate 8 derived from 4 was treated with sodium azide to afford the modified PEG-N3 9. On the other hand, the 4-propargylated TEMPO 10 was prepared using propargyl bromide by the same already reported method.12 After some trials, compound 10 was smoothly ligated with 9 using a catalytic amount of CuSO4 and sodium ascorbate in CH2Cl2-H2O at room temperature to give the PEG-supported TEMPO 11 bearing a triazol ring as the linker, although the purity (ca. 70%) was lower than that of compound 7.
For the oxidation of alcohols with the PEG derivatives, we decided to use Oxone, which was well-known as an environmentally safe and nontoxic reagent, as the most suitable co-oxidant, and we noticed that the use of Oxone allowed us to proceed with easy handling and a controllable addition.13,14 In our case, the PEG-supported precatalysts were soluble in some organic solvents, while Oxone was not. These compounds could then be separated by simple filtration.
Initially, the oxidation of 1-octanol (12a) as a representative aliphatic primary alcohol by the PEG supported TEMPO 5 bearing an ester linker was investigated under various reaction conditions (Scheme 5). In the preliminary experiments, we focused on the reaction conversion, which was determined by GC. The experimental procedure was quite simple, and a typical example is as follows. To a solution of 5 (0.01 eq) in CH2Cl2 were added Oxone (2.2 eq), tetrabutyl ammonium bromide (TBAB, 0.04 eq), and 12a (100 mg, 0.77 mmol; substrate conc., 0.15 M), and then the mixture was stirred at room temperature. Because compound 5 contained two TEMPO parts per molecule, the solution substantially included 2 mol% of TEMPO. As expected, the PEG-supported TEMPO 5 proved to be an effective preoxidant, and the conversion reached 100% after a 24 h-reaction. During the reaction, the halide ion significantly affected the efficiency of the catalysis, and the reaction with TBAF (fluoride) and TBAI (iodide) hardly proceeded. The addition of even TBAC (chloride), which indicated an effective halide source coupling with TEMPO,13 decreased the conversion to 51%. We also investigated the influence of the solvent. Many solvents including 1,4-dioxane, THF, DMF, and ethyl acetate scarcely provided the oxidized products, while the reaction in toluene, which was effective for the oxidation of alcohols with TEMPO,13 gave a low conversion (34%). On the other hand, the alcohol 12a was also effectively oxidized in CHCl3 and benzotrifluoride (CF3C6H5, BTF), and the conversions were 98% and 100%, respectively. BTF is a relatively low toxic compound, and has recently been used as an alternative solvent to CH2Cl2 in organic reactions.15,16 Because BTF has a lower volatility as compared to CH2Cl2, we easily maintained the reaction conditions in BTF. We then decided to mainly use BTF mainly in the following experiment.
Table 1 shows the oxidations of 12a with some TEMPO derivatives including the PEG-supported compounds 5 and 11 under the optimum conditions. The oxidation of 12a with 5 (0.05 eq, 10 mol% of TEMPO) in BTF completely proceeded for 9 h to afford the corresponding oxidized compounds (entry 1). During the reaction process, we achieved the easy separation of the products due to the use of PEG as the basic matrix of TEMPO without any extraction procedures. When the reaction was finished, a simple filtration removed Oxone from the reaction mixture. After evaporation, the residue was poured into Et2O to precipitate the mixture of the PEG-supported 5 and TBAB, which was collected by filtration. Finally, the products were obtained after evaporation. The products of the reaction with 5 included both octanal (13a) and octanoic acid (14a, the ratio of 13a/14a was 84/16), but the aldehyde 13a as the major product was easily isolated by column chromatography in a good isolated yield (83%). The oxidation of 13a to 14a was caused by Oxone,17,18 because TEMPO without Oxone did not catalyze the oxidation of 13a under the same reaction conditions. Practically, the oxidation of 13a with Oxone (2.2 eq) in BTF at room temperature proceeded to afford the carboxylic acid 14a, and the conversion of the 24-h reaction was up to 100%. Unfortunately, reducing the amount of Oxone did not improve the selectivity, but required a longer reaction time for completely consuming the starting alcohol. On the other hand, the original TEMPO had a lower activity in this system, and the conversion for the 9-h reaction was 81%, while the ratio of 13a/14a was slightly up to 92/8 (entry 2). The alternative PEG-supported compound 11 bearing a triazol spacer also had a higher catalytic activity. The oxidation of 12a with 11 also finished within 9 h, but the main product was the carboxylic acid 14a (entry 3). Interestingly, the commercially available PEG-TEMPO 1 gave the almost same result of that of 11 (entry 4). In these cases, lowering the reaction time did not significantly improve the selectivity. It is noteworthy that the reaction using TEG-TEMPO 7, which has the same spacer as that of the compound 5 and a shorter ethylene glycol chain, gave the aldehyde 13a as the major product, but the conversion was quite low (64%). These results indicated that the oxidation of 12a could be accelerated by the presence of the PEG part of the catalyst in this reaction system, and the structure of the spacer between the PEG and TEMPO parts could also affect the oxidation activity of 13a with Oxone. We also examined the reaction of 12a using insoluble polystyrene and silica gel-supported TEMPO derivatives, which were purchased from Aldrich (entries 6 and 7, respectively). As expected, the reactivities were lower than those of the PEG-TEMPO derivatives, and the conversions with polystyrene-TEMPO and silica gel-TEMPO sfter 9 hours were 69 and 37%, respectively. Finally, we decided to use compound 5 in the following studies, because we needed the lowest amount of the precatalyst 5 in the reaction due to the highest purity, and the ratio of the products was easy to control when using 5.
We next tried to examine the oxidation of other primary alcohols using the PEG-supported 5 (Table 2). In the case of benzyl alcohol (12b) (entry 1), the oxidation in BTF was finished in only 3 h with an excellent selectivity to afford benzaldehyde (13a) as almost the sole product (13b/14b = 98/2). On the other hand, the reaction of 3-phenyl-1-propanol (12c) in BTF for 10 h gave 3-phenypropanoic acid (14c) as the major product (13c/14c = 33/67). In this case, changing the solvent to CH2Cl2 inverted the 13c/14c ratio, and the reaction of 12c in CH2Cl2 for 9 h gave the 3-phenylpropanal (13c) in 62% isolated yield (13c/14c = 76/24; entry 2). In the case of 12a, a longer reaction time in BTF gave the carboxylic acid 14a as the major product, but the time taken to thoroughly react the aldehyde 13a needed 24 h. In a previous paper, DMF was used as an effective solvent for the oxidation of aldehydes to carboxylic acids.18 In order to accelerate the further oxidation of 12a to 13a, we then examined the addition of DMF to the reaction mixture after 12a was completely consumed (after 9h; entry 3). As expected, the oxidation of 13a was smoothly finished within only another 2 h, and the compound 14a was produced in 98% yield. This procedure was also applicable for the efficient production of other carboxylic acids, and the reactions of 12b and 12c also gave benzoic acid (14b) and 14c in good yields, respectively (entries 4 and 5). In these cases, the carboxylic acids containing no significant impurities were obtained without any purification steps.
We next applied our oxidation protocol to various secondary alcohols 15, and these results are summarized in Table 3. As expected, in all cases, the PEG-supported TEMPO 5 efficiently catalyzed the oxidations of 15a-g, and the conversions reached 100%. Although the reactivity of the aliphatic secondary alcohols was lower than that of the primary alcohols, the 24-h reactions of 2-octanol (15a, entry 1), 2-decanol (15b, entry 2), 4-phenyl-2-butanol (15c, entry 3), and 4-benzyloxy-2-butanol (15d, entry 4) gave the corresponding ketones, 2-octanone (16a), 2-decanone (16b), 4-phenyl-2-butanone (16c), and 4-benzyloxy-2-butanone (16d) in good yields, respectively. On the other hand, the oxidation of an aromatic alcohol, 1-phenylethanol (15e, entry 5), and cyclic alcohols, cyclohexanol (15f, entry 6) and cyclooctanol (15g, entry 7), smoothly proceeded in a manner similar to the primary alcohols to afford acetophenone (16e), cyclohexanone (16f), and cyclooctanone (16g), respectively.
As mentioned above, the recovery of the PEG-supported TEMPO 5 was quite simple, and the reusability of the precatalyst 5 was examined for the oxidation of 15b (Table 4). In almost cases, the precatalyst was recovered in ca. 90% yield based on the weight of the starting 5. The recovered and dried solid 5 included a small amount of TBAB, however, the contained TBAB could be removed by washing the solution of the solid in CH2Cl2 with 2 M HCl. However, for those listed in Table 4, we directly reused the recovered 5 again for the next run without any further purification in order to produce a simple procedure. It is noteworthy that the conversions of the reactions from the first run to sixth run remained almost constant (>93%). The conversions gradually decreased from the seventh run, because the impurities in the recovered 5 steadily increased. However, we observed the moderate activity (conv. 69%) in the recovered precatalyst even after ten uses.
CONCLUSION
In summary, we have easily prepared new soluble polymer bound TEMPO derivatives and used it for the oxidation of primary and secondary alcohols in combination with Oxone as the co-oxidant in BTF at room temperature. We also investigated the effect of a basic matrix of TEMPO derivatives, and we found that the PEG-supported TEMPO derivative bearing a succinate spacer gave the best result. Our mild and environment-friendly oxidation system has the advantages not only for the facile separation of products, but also for an easy recovery and convenient recycling.
EXPERIMENTAL
4.1. General
1H (300 MHz) and 13C (75 MHz) NMR spectra were measured on a JEOL JNM AL-300 with tetramethylsilane (TMS) as the internal standard. ESI-TOF mass spectra were measured in MeOH-H2O solution including AcONa with a JEOL JMS-T100. GLC data were obtained on GL Sciences GC 353B and sic 480II data station (System Instruments Inc.) using CP-Cyclodextrin-B-236-M19 (Chrompack; 0.25 mm x 50 m; carrier gas, He; head pressure, 2.4 kg/cm2). E. Merck Kieselgel 60 F254 Art.5715 was used for analytical TLC. Preparative TLC was performed on E. Merck Kieselgel 60 F254 Art.5744. Column chromatography was performed with Silica Gel 60N (63-210 mm, Kanto Chemical Co. Inc.). All other chemicals were also obtained from commercial sources. Polymer-bound reagents were purchased from Aldrich Chem Co. [PEG supported-TEMPO with an ether linker (No. 678325), TEMPO polymer-bound (No. 566098), TEMPO on silica gel (No. 709859)].
4.2. Preparation of PEG-supported TEMPO 5.
Under an argon atmosphere, to a solution of 4-hydroxy TEMPO, the free radical (2, 500 mg, 2.90 mmol) in CH2Cl2 (5.5 mL) were added solutions of DMAP (1.06 g, 8.71 mmol) in CH2Cl2 (7 mL) and succinic anhydride (872 mg, 8.71 mmol) in CH2Cl2 (39 mL) at 0 °C. After stirring overnight at room temperature, the mixture was washed with 2 M HCl, and the products were extracted from the water layer with CH2Cl2 (x3). The combined organic layer was dried over Na2SO4. After evaporation under reduced pressure, the residue was purified by flash column chromatography on silica gel (hexane/AcOEt = 1/1) to give the compound 3 as an orange crystal (779 mg, 99%); 1H NMR (500 MHz, CDCl3) δ = 1.29 (s, 6H), 1.38 (s, 6H), 1.94 (dd, J1=J2=12.5 Hz, 2H), 2.30 (dd, J1=4.0 Hz, J2=13.5 Hz, 2H), 2.42-2.48 (m, 2H), 2.63–2.69 (m, 2H), 4.95–5.04 (m, 2H); 13C NMR (125 MHz, CDCl3) δ = 20.9, 27.9, 30.5, 31.2, 41.7, 64.7, 65.0, 173.6, 179.4.
Under an argon atmosphere, to a solution of PEG4600-OH (4, 2.00 g, 0.435 mmol) in CH2Cl2 (20 mL) were added a solution of 3 (391 mg, 1.44 mmol) in CH2Cl2 (5 mL), DMAP (266 mg, 2.18 mmol), and a solution of DCC (283 mg, 1.31 mmol) in CH2Cl2 (25 mL) at 0 °C. After stirring overnight at room temperature, the mixture was filtrated through a Celite pad. After the filtrate was washed with 2 M HCl, the organic layer was dried over Na2SO4. After evaporation in vacuo, the residue was poured into Et2O to precipitate the PEG-supported TEMPO 5 as a white solid in 98% yield (2.18 g; purity, ca. 96%); 1H NMR (500 MHz, CDCl3 with phenylhydrazine) δ 1.21 (s, 12H), 1.24 (s, 12H), 1.56-1.72 (m, 4H), 1.88-1.97 (m, 4H), 2.57-2.66 (m, 8H), 3.44-3.80 (m, PEG-methylenes), 4.25 (t, J = 5.0 Hz, 4H), 5.04-5.11 (m, 2H); 13C NMR (125 MHz, CDCl3 with phenylhydrazine) δ 22.9, 28.2, 28.6, 40.8, 43.0, 63.1, 66.3, 68.3, 69.5, 69.8 (PEG-methylenes), 70.0, 171.0, 171.6; ESI-TOF MS m/z 2555 Da (2553.5 Da calcd for [C13H21NO5(CH2CH2O)103C13H21NO4·2Na]2+). The purity of 5 was determined by a 1H NMR analysis. The integrated intensities of protons of a TEMPO part were compared with that of the PEG-methylenes as the reference.
4.3. Preparation of TEG-supported TEMPO 7.
Under an argon atmosphere, to a solution of 3 (706 mg, 2.59 mmol) in CH2Cl2 (10 mL) were added a solution of TEG-OH (6, 62.9 mg, 0.324 mmol) in CH2Cl2 (5 mL), DMAP (949 mg, 7.77 mmol), and a solution of DCC (3.20 g, 15.5 mmol) in CH2Cl2 (10 mL) at 0 °C. After stirring overnight at room temperature, the mixture was filtrated through a Celite pad. After evaporation under reduced pressure, the residue was purified by flash column chromatography on silica gel (hexane/AcOEt = 5/1 to 1/1, to AcOET) to give the compound 7 as an orange liquid (192 mg, 84%); 1H NMR (500 MHz, CDCl3 with phenylhydrazine) δ 1.21 (s, 12H), 1.24 (s, 12H), 1.62 (dd, J1 = J2 = 11.5 Hz, 4H), 1.87-1.97 (m, 4H), 2.56-2.62 (m, 4H), 2.62-2.68 (m, 4H), 3.60-3.68 (m, 8H), 3.71 (t, J = 4.5 Hz, 4H), 4.26 (t, J = 4.5 Hz, 4H), 5.02-5.10 (m, 2H); 13C NMR (125 MHz, CDCl3 with phenylhydrazine) δ 21.1, 28.9, 29.2, 41.8, 43.2, 63.8, 65.5, 69.0, 70.5, 70.6, 171.7, 172.2; ESI-TOF MS m/z 725.3825 (725.3831 calcd for C34H58N2O13Na+).
4.4. Preparation of PEG-supported TEMPO 11.
Under an argon atmosphere, methanesulfonyl chloride (0.35 mL, 4.51 mmol) and triethylamine (1.50 mL, 10.8 mmol) were added to a solution of 4 (3.00 g, 0.60 mmol) in CH2Cl2 (13 mL), and the solution was stirred overnight at room temperature. After evaporation in vacuo, the residue was poured into Et2O to precipitate a white solid. After the solid was washed with 2-propanol, evaporation and precipitation gave the mesylate 8 as a white solid in 99% yield (3.03 g); 1H NMR (500 MHz, CDCl3) δ 3.09 (s, 3H) 3.38 (s, 3H), 3.46-3.82 (m, PEG-methylenes), 4.39 (t, J = 4.5 Hz, 2H); 13C NMR (125 MHz, CDCl3) δ 37.6, 58.9, 68.9, 69.2, 70.4 (PEG), 70.5, 70.6, 71.8.
Under an argon atmosphere, sodium azide (256 mg, 3.94 mmol) were added to a solution of 8 (4.00 g, 0.788 mmol) in toluene (10.5 mL), and the solution was stirred overnight at 95 °C. The mixture was evaporated in vacuo, and the residue was filtrated through a Celite pad. After evaporation, the residue was poured into Et2O to precipitate the compound 9 as a white solid in 95% yield (3.75 g); 1H NMR (500 MHz, CDCl3) δ 3.38 (s, 3H), 3.40 (t, J = 5.0 Hz, 2H), 3.46-3.82 (m, PEG-methylenes); 13C NMR (125 MHz, CDCl3) 50.5, 58.9, 69.9, 70.4 (PEG), 70.55, 70.62, 71.8.
Under an argon atmosphere, to NaH (558 mg, 12.8 mmol) were added a solution of 2 (2.00 g, 11.6 mmol) in DMF (8 mL) at 0 °C. After stirring 1 h, propargyl bromide (1.89 ml, 15.9 mmol) was added to the mixture. After stirring for 20 h at room temperature, the mixture was quenched with water, and the products were extracted with AcOEt (x3). The combined organic layer was washed with brine (x2), and dried over Na2SO4. After evaporation under reduced pressure, the residue was purified by flash column chromatography on silica gel (hexane/AcOEt = 10/1) to give the compound 10 as an orange crystal (1.56 g, 64%); 1H NMR (500 MHz, CDCl3) δ = 1.19 (s, 6H), 1.25 (s, 6H), 1.51 (dd, J1=J2=11.5 Hz, 2H), 1.91-2.07 (m, 2H), 2.43 (dd, J1=J2=2.5 Hz, 1H), 3.85 (dddd, J1=J2=4.0 Hz, J3=J4=11.5 Hz, 1H), 4.17 (d, J = 2.5 Hz, 2H); 13C NMR (125 MHz, CDCl3) δ = 20.7, 31.8, 44.1, 55.2, 59.8, 69.5, 74.1, 80.0.
Under an argon atmosphere, to a solution of 9 (1.00 g, 0.290 mmol) in CH2Cl2 (1.5 mL) were added CuSO4·5H2O (7.3 mg, 29.2 mmol), sodium ascorbate (17.4 mg, 87.8 mmol), 10 (146 mg, 0.695 mmol), and water (1.5 mL). After stirring overnight at room temperature, 2 M HCl was added to the mixture, and the products were extracted with CH2Cl2 (x4). The combined organic layer was dried over Na2SO4. After evaporation in vacuo, the residue was poured into Et2O to precipitate the PEG-supported TEMPO 11 as an orange solid in 97% yield (1.10 g; purity, ca. 70%); 1H NMR (500 MHz, CDCl3 with phenylhydrazine) δ 1.35 (s, 12H), 1.48 (s, 12H), 1.78-2.00 (m, 4H), 2.07-2.20 (m, 4H), 3.50 (t, J = 5.0 Hz, 4H), 3.45-3.75 (m, PEG-methylenes), 3.78 (t, J = 5.0 Hz, 4H), 3.87 (t, J = 5.0 Hz, 8H), 4.55 (t, J = 5.0 Hz, 4H), 4.65 (s, 4H), 7.36 (s, 2H); 13C NMR (125 MHz, CDCl3 with phenylhydrazine) δ 21.1, 30.8, 43.9, 50.2, 61.8, 69.5, 70.5 (PEG-methylenes), 123.7, 145.3, 151.2; ESI-TOF MS m/z 2536 Da (2523 Da calcd for [C12H20N4O2(CH2CH2O)103 C12H20N4O2·2Na]2+). The purity of 11 was determined by a 1H NMR analysis. The integrated intensities of protons of a TEMPO part were compared with that of the PEG-methylenes as the reference.
4.5. Typical procedure for the oxidation of 2-decanol (15b) using 5 with Oxone.
Under an argon atmosphere, to a solution of 5 (648 mg, 0.126 mmol) in BTF (10 mL) were added Oxone (3.41 g, 5.54 mmol), TBAB (28.1 mg, 0.101 mmol), and a solution of 15b (398 mg, 2.52 mmol) in BTF (10 mL). After stirring for 24 h at room temperature, the mixture was filtrated through a cotton plug. After evaporation in vacuo, the residue was poured into Et2O to precipitate 5 (617 mg, 95%). After evaporation in vacuo, the residue was purified by flash column chromatography on silica gel (hexane/AcOEt = 20/1) to give 16b as a colorless oil (314 mg, 80%).
The reactions of the other substrates were carried out by the same procedure. The results were shown in the text.
4.6. Several data of the GC conditions.
Oxidation of 1-octanol (12a): injection, 160 °C; detection, 160 °C; oven, 140 °C; retention time, 5.0 (1-octanal (13a)), 6.4 (12a), and 15.1 (octanoic acid (14a)) min.
Oxidation of benzyl alcohol (12b): injection, 160 °C; detection, 160 °C; oven, 140 °C; retention time, 5.4 (benzaldehyde (13b)), 6.3 (12b), and 6.8 (methyl benzoate) min.
Oxidation of 3-phenyl-1-propanol (12c): injection, 200 °C; detection, 200 °C; oven, 180 °C; retention time, 5.4 (3-phenylpropanal (13c)), 6.5 (12c), and 11.8 (3-phenylpropanoic acid (14c)) min.
Oxidation of cyclohexanol (15f): injection, 160 °C; detection, 160 °C; oven, 140 °C; retention time, 4.7 (cyclohexanone (16f)) and 6.6 (dodecane as a internal standard) min.
ACKNOWLEDGEMENTS
This work was partially supported by a Grant-in-Aid for Scientific Research (No. 21550051) from the Ministry of Education, Culture, Sports, Science and Technology, Japan. We thank Material Science Research Center (Meisei university) for NMR analysis.
References
1. For representative examples, see: T. Fey, H. Fischer, S. Bachmann, K. Albert, and C. Bolm, J. Org. Chem., 2001, 66, 8154; CrossRef T. Yuen Sze But, Y. Tashino, H. Togo, and P. H. Toy, Org. Biomol. Chem., 2005, 3, 970; CrossRef A. Gheorghe, A. Matsuno, and O. Reiser, Adv. Synth. Catal., 2006, 348, 1016. CrossRef
2. For representative reviews, see: D. J. Gravert and K. D. Janda, Chem. Rev., 1997, 97, 489; CrossRef P. Wentworth and K. D. Janda, Chem. Commun., 1999, 1917; CrossRef T. J. Dickerson, N. N. Reed, and K. D. Janda, Chem. Rev., 2002, 102, 3325; CrossRef D. E. Bergbreiter, Chem. Rev., 2002, 102, 3345. CrossRef
3. For examples, see: M. Benaglia, G. Celentano, and F. Cozzi, Adv. Synth. Catal., 2001, 343, 171; CrossRef G. Pozzi, M. Cavazzini, S. Quici, M. Benaglia, and G. Dell'Anna, Org. Lett., 2004, 6, 441; CrossRef M. Oikawa, T. Tanaka, S. Kusumoto, and M. Sasaki, Tetrahedron Lett., 2004, 45, 787; CrossRef N. N. Reed, T. J. Dickerson, G. E. Boldt, and K. D. Janda, J. Org. Chem., 2005, 70, 1728; CrossRef J.-H. Li, X.-C. Hu, Y. Liang, and Y.-X. Xie, Tetrahedron, 2006, 62, 31; CrossRef S. H. Hong and R. H. Grubbs, Org. Lett., 2007, 9, 1955; CrossRef G. Tocco, A. Fais, G. Meli, M. Begala, G. Podda, M. B. Fadda, M. Corda, O. A. Attanasi, P. Filippone, and S. Berretta, Bioorg. Med. Chem., 2009, 19, 36; CrossRef G. Tocco, C. M. Carnonaro, G. Meli, and G. Podda, Molecules, 2009, 14, 1044; CrossRef R. Zimmer, V. Dekaris, M. Knauer, L. Schefzig, and H.-U. Reissig, Synth. Commun., 2009, 39, 1012. CrossRef
4. For reviews about PEG use in pre-drug chemistry, see: F. M. Veronese and M. Morpurgo, Il Farmaco, 1999, 54, 497; CrossRef R. B. Greenwald, J. Controlled Release, 2001, 74, 159. CrossRef
5. For enzymatic hydrolysis with PEG-supported substrates, see: M. Shimojo, K. Matsumoto, M. Nogawa, Y. Nemoto, and H. Ohta, Tetrahedron Lett., 2004, 45, 6769; CrossRef M. Nogawa, M. Shimojo, K. Matsumoto, M. Okudomi, Y. Nemoto, and H. Ohta, Tetrahedron, 2006, 62, 7300; CrossRef M. Okudomi, M. Shimojo, M. Nogawa, A. Hamanaka, N. Taketa, and K. Matsumoto, Tetrahedron Lett., 2007, 48, 8540; CrossRef M. Okudomi, M. Nogawa, N. Chihara, M. Kaneko, and K. Matsumoto, Tetrahedron Lett., 2008, 49, 6642; CrossRef M. Okudomi, M. Shimojo, M. Nogawa, A. Hamanaka, N. Taketa, T. Nakagawa, and K. Matsumoto, Bull. Chem. Soc. Jpn., 2010, 83, 182. CrossRef
6. G. Pozzi, M. Cavazzini, S. Quici, M. Benaglia, and G. Dell’Anna, Org. Lett., 2004, 6, 441; CrossRef M. Benaglia, A. Puglisi, O. Holczknecht, S. Quici, and G. Pozzi, Tetrahedron, 2005, 61, 12058. CrossRef
7. P. Ferreira, W. Hayes, E. Phillips, D. Rippon, and S.-C. Tsang, Green. Chem., 2004, 6, 310; CrossRef P. Ferreira, E. Phillips, D. Rippon, S.-C. Tsang, and W. Hayes, J. Org. Chem., 2004, 69, 6851. CrossRef
8. F. Pedro, P. Emyr, D. Rippon, and S.-C. Tsang, Appl. Cat. B: Envionmental, 2005, 61, 206. CrossRef
9. J. Luo, C. Pardin, W. D. Lubell, and X. X. Zhu, Chem. Commun., 2007, 2136. CrossRef
10. C. W. Y. Chung and P. H. Toy, J. Comb. Chem., 2007, 9, 115. CrossRef
11. C. W. Tornoe, C. Christensen, and M. Meldal, J. Org. Chem., 2002, 67, 3057; CrossRef V. V. Rostovtsev, L. G. Green, V. V. Fokin, and K. B. Sharpless, Angew. Chem. Int. Ed., 2002, 41, 2596. CrossRef
12. A. Gheorghe, E. Cuevas-Yanez, J. Horn, W. Bannwarth, B. Narsaiah, and O. Reiser, Synlett, 2006, 17, 2767. CrossRef
13. C. Bolm, A. S. Magnus, and J. P. Hildebrand, Org. Lett., 2000, 2, 1173. CrossRef
14. For examples, see: P. L. Bragd, A. C. Besemer, and H. Van Bekkum, Carbohydrate Polymers, 2002, 49, 397; CrossRef R. Kowalczyk and J. Skarzewski, Polish J. Chem., 2002, 76, 1413; A. P. Dobbs, M. J. Penny, and P. Jones, Tetrahedron Lett., 2008, 49, 6955. CrossRef
15. I. Ryu, H. Matsubara, C. Emnet, J. A. Gladysz, S. Takeuchi, Y. Nakamura, and D. P. Curran, 'Green Reaction Media in Organic Synthesis,' ed. by K. Mikami, Blackwell Publishing Ltd., 2005, p. 59.
16. For recent examples, see: S. G. Nelson, C. Zhu, and X. Shen, J. Am. Chem. Soc., 2004, 126, 14; CrossRef K. Mikami, M. N. Islam, M. Yamanaka, Y. Itoh, M. Shinoda, and K. Kudo, Tetrahedron Lett., 2004, 45, 3681; CrossRef D. Crich and Y. Zou, J. Org. Chem., 2005, 70, 3309; CrossRef K. W. C. Poon and G. B. Dudley, J. Org. Chem., 2006, 71, 3923; CrossRef Q. Chu, C. Henry, and P. D. Curran, Org. Lett., 2008, 10, 2453; CrossRef E. Hasegawa, Y. Ogawa, K. Kakinuma, H. Tsuchida, E. Tosaka, S. Takizawa, H. Muraoka, and T. Saikawa, Tetrahedron, 2008, 64, 7724. CrossRef
17. For examples using Oxone in oxidation of aldehydes, see: K. S. Webb and S. J. Ruszkay, Tetrahedron, 1998, 54, 401; CrossRef R. Gandhari, P. P. Maddukuri, and T. K. Vinod, J. Chem. Educ., 2007, 84, 852; CrossRef M. Uyanik, M. Akakura, and K. Ishihara, J. Am. Chem. Soc., 2009, 131, 251. CrossRef
18. B. R. Travis, M. Sivakumar, G. O. Hollist, and B. Borhan, Org. Lett., 2003, 5, 1031. CrossRef