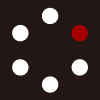
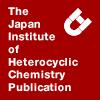
HETEROCYCLES
An International Journal for Reviews and Communications in Heterocyclic ChemistryWeb Edition ISSN: 1881-0942
Published online by The Japan Institute of Heterocyclic Chemistry
e-Journal
Full Text HTML
Received, 28th December, 2010, Accepted, 1st February, 2011, Published online, 4th February, 2011.
DOI: 10.3987/REV-10-691
■ Synthetic Studies on Nitrogen-Containing Fused-Heterocyclic Compounds Based on Thermal Electrocyclic Reactions of 6π-Electron and Aza 6π-Electron Systems
Tominari Choshi and Satoshi Hibino*
Graduate School of Pharmacy and Pharmaceutical Sciences, Faculty of Pharmacy and Pharmaceutical Sciences, Fukuyama University, Fukuyama, Hiroshima 729-0292, Japan
Abstract
Syntheses of carbazole alkaloids and indoles by an allene-mediated electrocyclic reaction of a 6π-electron system (II) through a tautomeric process, and syntheses of several fused pyridine ring systems including alkaloids by a thermal electrocyclic reaction of an aza 6π-electron system having an oxime or isocyanate (III) through either the elimination of a small molecule or a tautomeric process are described.CONTENTS
I. INTRODUCTION
II. SYNTHETIC STUDIES USING AN ALLENE-MEDIATED ELECTROCYCLIC REACTION
III. SYNTHETIC STUDIES USING A THERMAL AZA-ELECTROCYCLIC REACTION
IV. CONCLUSION
I. INTRODUCTION
We are currently interested in the synthesis of the nitrogen-containing condensed heteroaromatic compounds by a thermal electrocyclic reaction of either a 6π-electron or an aza 6π-electron system. A thermal electrocyclic reaction of a 6π-electron system to cyclohexadiene occurs as a disrotatory reversible process.1 The irreversible conversion of cyclohexadiene can be controlled by the elimination of a small molecule. The replacement of one carbon atom of a 6π-electron system with a nitrogen atom produces an aza 6π-electron system. The thermal electrocyclic reaction of an aza 6π-electron system to dihydropyridine occurs in a similar process. The irreversible conversion of dihydropyridine should be controlled by the elimination of a small molecule. In addition, a thermal electrocyclic reaction of a 6π-electron or an aza 6π-electron system where one member of the double bond is a part of an aromatic or heteroaromatic ring should provide a variety of fused heteroaromatic compounds. Since 1980, new syntheses of carbazole alkaloids including pyrido[4.5-b]carbazole alkaloids based on a thermal electrocyclic reaction of a 6π-electron system involving the indole 2,3-bond have been reported.2a-f,3a,b,d,e Moreover, syntheses of thieno[3,2-c]pyridine and pyrido[4,3-b]indole (γ-carboline) using the thermal electrocyclic reaction of aza 6π-electron systems involving the thiophene 2,3-bond and the indole 2,3-bond were initially reported in 1984,2g,3a and subsequently the methodology involving the indole 2,3-bond was proved to be an efficient procedure for nitramarine (β-carboline),2h,3a Trp-P-1 and Trp-P-2 (γ-carbolines),2i,j,3a and AαC and MeAαC (α-carbolines).2k,3a These results have been reviewed as [b]-annelated indoles by thermal electrocyclic reactions, except for thieno[3,2-c]pyridine, from 1980 until 1995.3a Furthermore, the methodology involving the benzene 1,2-bond was initiated to prepare isoquinoline2l and the isoquinoline part of aaptamine.2m,n To develop milder conditions of a thermal electrocyclic reaction for the 6π-electron system, construction of a highly-substituted carbazole framework was established using an allene-mediated electrocyclic reaction followed by a tautomeric process involving the indole 2,3-bond. Taking advantage of this strategy, several total syntheses of carbazole alkaloids were achieved (II).3c-e Furthermore, applications of the thermal electrocyclic reaction of aza 6π-electron systems involving the imidazole 4,5-bond, the indole 2,3-bond, and the benzene 1,2-bond have been also developed through either the oximes or the isocyanate (III).3c These results from 1993 through 2010 are described in the present review.
II. SYNTHETIC STUDIES USING AN ALLENE-MEDIATED ELECTROCYCLIC REACTION
II-1. Synthesis of carbazoles and carbazole alkaloids through 2-allenylindoles
II-1-1. Synthesis of carazostatin, hyellazole, and carbazoquinocins
Total syntheses of carazostatin (9c) isolated from Streptomyces chromofuscus,4 hyellazole (10a) isolated from blue-green alga Hyella caespitosa,5 and carbazoquinocins B-F (10b-f) isolated from Streptomyces violaceus 2448-SVT26 have been achieved (Scheme 1). Treatment of the alkenylindole 1a with ethynylmagnesium bromide, followed by etherification of the resulting alcohol with chloromethyl methyl ether (MOMCl), yielded the 3-alkenyl-2-propargylindole 2a. In a similar manner, compound 2b was obtained from 1b in two steps. Compound 2a was heated at 90 oC in t-BuOH in the presence of t-BuOK, according to the procedure of Kanematsu for allene generation,7 to yield the expected carbazole 4a together with the N-deprotected carbazole 5. By contrast, heating the N-MOM-3-alkenyl-2-propargylindole 2b in t-BuOH in the presence of t-BuOK produced the N-MOM-carbazole 4b. The N-protecting groups of 4a and 4b were removed, respectively, to form the same carbazole 5. Treatment of 5 with TMSCl and NaI followed by treatment of trifluoromethanesulfonic anhydride (Tf2O) and pyridine gave the triflate 7. The Suzuki-Miyaura reaction8 between 7 and phenylboronic acid gave the 1-phenylcarbazole 8a, which was treated with BBr3 followed by O-methylation to produce hyellazole (10a). The cross-coupling reaction between 7 and 9-heptyl-9-BBN also gave the 1-heptylcarbazole 8c, which was treated with BBr3 to produce carazostatin (9c). Carbazoquinocin C (10c) was readily obtained from carazostatin (9c) through an oxidation step with (PhSeO)2O. In a similar way, triflate 7 was subjected to a Pd-catalyzed cross-coupling reaction with 9-alkyl-9-BBN to give the 1-alkylcarbazoles 8b and 8d-f. Subsequent treatment of 8d and 8d-f with BBr3 afforded 3-hydroxycarbazoles 9b and 9d-f, which were oxidized with (PhSeO)2O to yield the corresponding carbazoquinocins B (10b), D (10d), E (10e), and F (10f), respectively.9
II-1-2. Synthesis of oxazolo[5,4-c]carbazole and oxazolo[4.5-c]carbazole
To synthesize the highly substituted carbazole alkaloids, antiostatins A1-4 (21) and antiostatins B2-5 (22),10 we assumed that a new type of tetracyclic oxazolo[5,4-c]carbazole 17 would be a functionalized key-intermediate (Scheme 2). Treatment of the N-SEM-oxazolone with t-BuLi followed by tributyltin chloride gave the stannyloxazolone 12, which was subjected to a Pd-catalyzed cross-coupling reaction11 with 3-iodoindole 11 to give an approximately 1:1 mixture of two isomeric 3-oxazolylindoles 13 and 14. The directed metalation of SEM-oxazolone 12 with t-BuLi did not work regioselectively. Subsequent treatment of indole 13 with NaH followed by the addition of benzenesulfonyl chloride or MOMCl gave 15a and 15b, respectively. The Grignard reaction of 2-formylindole 15a and 15b with ethynylmagnesium bromide yielded the propargyl alcohols, which were protected with MOMCl to give MOM-ethers 16a and 16b. Then, 16a was heated in t-BuOH/THF (3:1) in the presence of t-BuOK at 90 oC for 3 h to give the tetracyclic carbazole 17a. The N-MOM-indole 16b was heated in THF in the presence of tetrabutylammonium fluoride (TBAF) at 90 oC for 0.5 h to produce the tetracyclic carbazole 17b. A base exchange was not effective in either reaction. On the other hand, tetracyclic carbazoles 20a and 20b were prepared from the tentative product 14 in a similar manner. The structures of two tetracyclic N-benzenesulfonylcarbazoles 17a and 20a were determined by 2D-NOESY NMR spectra.12
II-1-3. Synthesis of an antibiotic carbazole alkaloid, carbazomycin G
Carbazomycins G (27a) and H (27b) were isolated from Streptoverticillium ehimense as a racemate together with carbazomycins A-F.13 The total synthesis of carbazomycin G (27a) was completed in seven steps (Scheme 3). The required 3-alkenyl-2-propargylindole 23 was prepared from the N-benzenesulfonyl-3-(2-methoxyethenyl)indole (22) in three steps through the indole-3-carbaldehyde. The precursor 23 was subjected to an allene-mediated electrocyclic reaction using t-BuOK in t-BuOH and THF at 90 oC to produce the desired 1,3-dioxygenated carbazole 24. Cleavage of the MOM-ether of 24 with TMSCl and NaI gave the 1-hydroxycarbazole 25. The oxidation to carbazole-1,4-quinone 26 from the phenol proceeded by [bis(trifluoroaxetoxy)iodo]benzene in aqueous CH3CN. Other oxidizing agents did not work well in this case. Finally, the nucleophilic addition of the carbazole-1,4-quinone 26 with MeLi provided carbazomycin G (27a).14
II-1-4. Synthesis of desprenyl-carquinostatin A and descycloavandulyl-lavanduquinocin
Carbazole-3,4-quinone alkaloids, carquinostatin A (37a) and lavanduquinocin (37b) were isolated from Streptomyces exfoliates 2419-SVT215a and Streptomyces viridochromogenes,15b respectively. An asymmetric synthesis of the common carbazole framework, 6-desprenyl-carquinostatin A 37 and descycloavandulyl-lavanduquinocin 37, toward the total synthesis of carquinostatin A (37a) and lavanduquinocin (37b) was established (Scheme 4). The required carbazole 1-triflate 7 was prepared from 3-alkenyl-2-propargylindole 2a in four steps (see: II-1-1). The 1-allylcarbazole 28 was synthesized by the Suzuki-Miyaura reaction8 between the triflate 7 and allylboronic acid pinacol ester. Subsequently, the Wacker reaction of 28 gave the acetonylcarbazole 29 followed by reduction of 29 with NaBH4 provided the racemic alcohol 30. Lipase QLM catalysis of the enantioselective acetylation of the racemic alcohol 30 gave the (-)-acetate 31 and the (+)-acetate 32 with high enantioselectivity.
The absolute stereochemistries of the (-)-alcohol 33 and the (+)-alcohol 32 were determined to be R- and S-configurations, respectively, by the advanced Mosher method. Cleavage of the ethyl ether of 29 with BBr3 afforded the 3-hydroxycarbazole 34, which was reduced with NaBH4 to provide the racemic alcohol 34. Subsequent lipase QLM catalyzed enantioselective esterification of 34 in the same manner gave the (-)-acetate 35 and the (+)-alcohol 36, respectively. This (-)-acetate 35 was the same as the (R)-(-)-acetate 35 derived from (-)-31 by treatment with BBr3. Oxidation of the (R)-(-)-acetate 35 followed by hydrolysis afforded the (R)-(-)-6-desprenyl-carquinostatin [and the (R)-(-)-descycloavandulyl-lavanduquinocin] 37. In addition, oxidation of the (S)-(+)-alcohol 36 provided the (S)-(+)-37 as an enantiomer.16
II-1-5. Synthesis of 1,3-disubstututed carbazole alkaloids, mukonine and clausine E
The simple 1-oxygenated 3-substituted carbazole alkaloids mukonine (47a) and clausine E (47b) (clauzoline I) were isolated from Murraya koenigii and Clausena excavata (and anisata), together with the related alkaloids.17 Two alkaloids were newly synthesized (Scheme 5). Nucleophilic addition reaction of ethyl 3-[2-formyl-N-(methoxymethyl)indol-3-yl]acrylate 38 with ethoxyacetylide (prepared from chloroacetaldehyde diethyl acetal with n-BuLi and diethylamine) gave the propargyl alcohol, which was treated with MOMCl to produce the propargyl ether 39. An allene-mediated electrocyclic reaction of 39 was carried out by t-BuOK to yield the exclusively carbazole-3-carboxylic acid 40 through a ring closure together with hydrolysis. On the other hand, the same reaction of 39 using TBAF gave the ester 42. The carbazole-3-carboxylic acid 40 was converted to the methyl carbazole-3-carboxylate 41. Subsequent oxidation of the alkoxymethyl group at the 2-position of 42 with DDQ provided the 2-formyl-1-hydroxycarbazole 43. Alkylation of the 1-hydroxycarbazole 43 was carried out by MeI or benzyl bromide to give the 1-methoxycarbazole 44a or 1-benzyloxycarbazole 44b, respectively. The Baeyer-Villiger reaction of 44a and 44b with m-chloroperbenzoic acid (mCPBA) afforded 2-hydroxycarbazole 45a and 45b, respectively. Additional treatment of 45a and 45b with N-phenyl(bistrifluoromethanesulfonylamide) (Tf2NPh) and NaH gave the triflates 46a and 46b, which were subjected to hydrogenolysis to produce mukonine (47a) and clausine E (47b) along with O-debenzylation.18
II-1-6. Synthesis of the poly-substituted carbazole alkaloid carbazomadurin A
Carbazomadurins A (56a) and B (56b) were isolated from Actinomadura madurae 2808-SV1.19 The required 7-oxygenated 2,3,4,7-tetrasubstituted indole 48 was prepared from the known ethyl 7-isopropyoxylindole-2-carboxylate in six steps (57%). We then synthesized 1,3,8-trioxygenated 1,2,3,5,8-pentasubstituted carbazole 50 from 48. The Pd-catalyzed cross-coupling reaction11 of 3-iodoindole 48 with tributyl(2-ethoxyvinyl)tin, the Grignard reaction of the 3-alkenylindole with ethynylmagnesium bromide, followed by protection of the resulting alcohol with MOMCl gave the 3-alkenyl-2-propargylindole 49 in three steps. When 49 was heated at 90 oC in the presence of t-BuOK in t-BuOH, the expected carbazole 50 was not detected. Alternatively, treatment of 49 with TBAF in THF at 80 oC afforded the expected carbazole 50 in somewhat low yield. Oxidation of the O-MOM-methyl group at the C-5 position of 50 with DDQ to the 5-formylcarbazole, cleavage of the O-MOM group with 4M HCl to the 1-hydroxycarbazole, followed by sequential treatment of the 1-hydroxy group with Tf2NPh gave the corresponding triflate 51 in three steps. Additional cleavage of both ethers at the 3- and 8-positions of 51 with BBr3 afforded the 3,8-dihydroxycarbazole, which was immediately protected with SEMCl and i-Pr2NEt to produce the 3,8-bis-O-SEM-carbazole 52. On the other hand, a pinacol borate 53 to introduce an alkenyl side chain with an E-configuration at the C1 position of 52 was prepared from 5-methyl-1-hexyne. Namely, zirconium-catalyzed carboalumination of 5-methyl-1-hexyne with Me3Al and zirconocene dichloride,20 followed by the addition of I2 afforded E-alkenyl iodide.
Subsequently, the Suzuki-Miyaura reaction8 of E-alkenyl iodide with bis(pinacolato)diboron in the presence of PdCl2(dppf) afforded the pinacol borate 53 (48% in three steps). The Suzuki-Miyaura reaction8 between the triflate 52 and the pinacol borate 53 gave the 1-alkenylcarbazole 54 in good yield. Removal of both SEM groups of 54 with TBAF afforded the dihydroxycarbazole 55. Finally, reduction of 55 with NaBH4 provided carbazomadurin A (56a) (Scheme 6).21
II-2. Synthesis of carbazole through a 3-allenylindole
II-2-1. Synthesis of a carbazole-1,4-quinone alkaloid, murrayaquinone A
The carbazole-1,4-quinone, murrayaquinone A (63) was isolated from Murraya eucrestifolia Hayata, together with three closely related alkaloids.22 For synthesis of the known 4-hydroxy-3-methylcarbazole (62), the benzyloxymethyl (BOM) group was used as a N-protecting group of 2-chloroindole-3-carbaldehyde (57a). Treatment of 57a with benzyl chloromethyl ether (BOMCl) afforded the N-BOM-indole 57b. The Pd-catalyzed cross-coupling reaction11 between 57b and ethenyl tributylstannane gave the 2-ethenylindole 58. The Grignard reaction of 58 with ethynylmagnesium bromide followed by treatment of the resulting alcohol with BOMCl produced the 2-ethenyl-3-propargylindole 59. The propargylindole 59 was subjected to a thermal electrocyclic reaction in the presence of t-BuOK to yield the carbazole 60. Deprotection of the N,O-bis-BOM groups of 60 was carried out under the Birch condition to give a separable mixture of N-hydroxymethyl-4-hydroxy-3-methylcarbazole (61) and 4-hydroxy-3-methylcarbazole (62). The N-hydroxymethyl group of 61 was removed by heating with Triton B to yield 62. A formal total synthesis of murrayaquinone A (63) was achieved (Scheme 7).23
II-2-2. Synthesis of the furo[3,2-a]carbazole alkaloid furostifoline
The tetracyclic furo[3,2-a]carbazole alkaloid furostifoline (71) was isolated from Murraya eucrestifolia Hayata along with the furo[3,2-g]carbazole alkaloid eustifoline D.24 The cross-coupling reaction8 of 2-chloroindole 57a with furane-3-boronic acid (64) was carried out in the presence of Pd(OAc)2 and PPh3 to give the 2-(3-furyl)indole 65. After protection of the indole nitrogen atom of 65 with BOMCl, a subsequent Grignard reaction with ethynylmagnesium bromide followed by additional protection of the resulting alcohol with BOMCl and NaH yielded the 2-(3-furyl)-3-propargylindole 66. Compound 66 was subjected to a thermal electrocyclic reaction in the presence of t-BuOK to produce the 4-oxygenated furocarbazole 67. The Birch reduction of 67 for the deprotection of N,O-bis-BOM groups gave a separable mixture of the expected 4-hydroxy-3-methylfuro[3,2-a]carbazole (69) and its N-hydroxymethylfuro[3,2-a]carbazole 68. Compound 68 was converted to 69 by heating with Triton B. Finally, treatment of the phenol 69 with Tf2O and pyridine afforded the triflate 70, which was subjected to a reductive cleavage of the 4-trifluoromethanesulfonyloxy group of 70 with Pd(OAc)2 and PPh3 in the presence of HCO2H and Et3N at 60 oC to give furostifoline (71) in excellent yield (Scheme 8).23b
II-2-3. Synthesis of the indolo[3,2-j]phenanthridine alkaloid calothrixin B
Novel indolo[3,2-j]phenanthridine alkaloids, calothrixins A (N-oxide of 79) and B (79) were isolated from Calothrix cyanobacteria.25 The Wittig reaction of indole-2-carbaldehyde 72 with 2-nitrobenzyltriphenylphosphorane gave the trans-2-(2-styryl)indole 73. Subsequent treatment of 73 with Cl2CHOMe in the presence of AlCl3 afforded the 2-alkenyl-3-formylindole 74. The Grignard reaction of 74 with ethynylmagnesium bromide yielded the propargyl alcohol, which was protected with MOMCl and i-Pr2NEt to produce the O-MOM ether 75. The 2-alkenyl-3-propargylindole 75 was subjected to a thermal electrocyclic reaction to yield the expected 4-oxygenated 2,3,4-trisubstituted carbazole 76 along with elimination of the benzenesulfonyl group. Sequential oxidation of 76 with DDQ followed by deprotection with 6 M HCl gave the 3-formylcarbazole 77. Reduction of the nitro group of 77 with 10% Pd-C and H2 followed by condensation afforded the indolo[3,2-j]phenanthridine 78, which was oxidized with cerium ammonium nitrate (CAN) to provide calothrixin B (79) (Scheme 9).26a,c,d
II-2-4. Biomimetic synthesis of calothrixin B
The Rickards group proposed that calothrixins A (79: N-oxide) and B (79) are derived from a hypothetical metabolite indolo[2,3-a]carbazole 85a, biosynthetically.25 The Suzuki-Miyaura coupling reaction8 of 2-bromoindole 80 with indole-2-boronic acid 81 gave the bisindole 82. Cleavage of the N-BOC group in 82 with TFA, protection of the both nitrogen atoms of bisindole with MOMCl and NaH, the Grignard reaction to the formyl group of bisindole, followed by treatment of the resulting secondary alcohol with MOMCl to produce the propargyl MOM-ether 83 in four steps. The propargylindole 83 was subjected to an allene-mediated electrocyclic reaction to yield the indolo[2,3-a]carbazole 84. Oxidation of 6-methylindolocarbazole 84 with DDQ afforded the 6-formylindolocarbazole 85b, which was an additional oxidation of 85b with CAN to produce the N-MOM-calothrixin B 88, directly. This result indicates that a quinone-imine structure 86 was formed, and then immediate hydrolysis of an imino group in 86, followed by an intramolecular condensation occurred to give the indolo[3,2-j]phenanthridine 88. Cleavage of the N-MOM group in 88 with provided calothrixin B (79). Thus a biomimetic synthesis of calothrixin B (79) was achieved through the fully protected indolo[2,3-a]carbazole 85b (Scheme 10).26b-d
II-3. Synthesis of indole through an 3- or 2-allenylpyrrole
II-3-1. Synthesis of 5-methylindole-4,7-quinone
A new antimicrobial indolequinone, 5-methylindole-4,7-quinone (99) was isolated from the mid-intestinal gland of the muricid gastropod Drupella fragum, together with two related indole-4,7-quinones.27 We planned a new synthesis of a 4-oxygenated 5-methylindole 96 based on an application of the allene-mediated electrocyclic reaction involving the pyrrole 2,3-bond (Scheme 11). After benzylation of the known pyrrole 89 with benzyl bromide (BnBr), the Pd-catalyzed cross-coupling reaction11 with ethenyl tributylstannane afforded the 2-ethenylpyrrole 91, which was
treated with ethynylmagnesium bromide, followed by O-methylation of the resulting alcohol with MeI to yield the propargyl methyl ether 92. The propargyl ether 92 was subjected to an electrocyclic reaction with t-BuOK to produce the desired 4-oxygenated indole 93 along with hydrolysis of ester. Cleavage of the benzyl group of 93 with AlCl3 failed. On the other hand, cleavage of the benzyl group of the ester 94, derived from 93, with AlCl3 provided the N-deprotected indole 95. Hydrolysis of 95, followed by decarboxylation with Cu afforded the 4-methoxy-5-methylindole (96). Direct cleavage of the methyl ether of 96 with BBr3 failed. The protection of the indole nitrogen atom of 96 with benzenesulfonyl group, followed by treatment of BBr3 gave the 4-hydroxyindole 97. Finally, oxidation of 97 with CAN provided the indole-4,7-quinone 98, which was treated with an aqueous NaHCO3 to yield 5-methylindole-4,7-quinone (99). The synthetic 99 was not consistent with the reported 99, however, in any aspect.28a
II-3-2. Synthesis of 6-methylindole-4,7-quinone
6-Methylindole-4,7-quinone (107) was synthesized to clarify the structure of the natural product, 5-methylindole-4,7-quinone (99) isolated from Drupella fragum (II-3-1).27 We also planned a synthesis of 7-oxygenated 6-methylindole 104 through an 3-allenyl-2-propargylpyrrole intermediate103 (Scheme 12). The Stille reaction11 of 3-bromopyrrole-2-carbaldehyde (100) with ethenyl tributylstannane afforded the 3-ethenylpyrrole 101. The Grignard reaction of 101 with ethynylmagnesium bromide followed by treatment of the alcohol with MOMCl produced 2-propagyl ether 102. The propargylpyrrole 102 was subjected to an electrocyclic reaction under the conditions of t-BuOK at room temperature to yield the 6-methylindole 104. Subsequent deprotection of the O-MOM group of 104 with 6 M HCl, followed by oxidation of the phenol 105 with salcomine and O2 gave the indole-4,7-quinone 106. Finally, removal of the N-tosyl group of 106 was carried out by
an aqueous NaHCO3 to produce the expected 6-methylindole-4,7-quinone (107). The structure of natural indole-4,7-quinone 99,26 however, was not identical with either synthetic 99 or 107. The exact structure of reported 99 is now unclear.28b
III. SYNTHETIC STUDIES USING THE THERMAL AZA-ELECTROCYCLIC REACTON
III-1. Synthesis of fused pyridine rings by a thermal electrocyclic reaction of an aza 6π-electron system using an oxime or oxime ether
III-1-1. Synthesis of 1H- and 3H-imidazo[4,5-c]pyridines
Synthetic routes to 1H- and 3H-imidazo[4,5-c]pyridines have been developed by an thermal electrocyclic reaction of 1-aza 6π-electron systems involving the imidazole 4,5-bond (Scheme 13). For the synthesis of 1H-imidazo[4,5-c]pyridines 112, the Wittig reaction of 4-bromo-5-formylimidazole 108 with several alkylidenetriphenylphosphoranes (CH2=, MeCH=, PhCH=) gave the 5-alkenylindoles 109. Subsequent treatment of 4-bromoimidazoles 109 with n-BuLi followed by quenching with several electrophiles [DMF, (MeCO)2O, PhCOCl] gave the corresponding 4-acylimidazoles 110, which were treated with hydroxylamine to yield the oximes 111 in an usual manner. The thermal electrocyclic reaction of 111 was carried out at reflux temperature in o-dichlorobenzene to produce the proposed 1H-imidazo[4,5-c]pyridine 112. Of eight examples attempted, seven were obtained in moderate to good yields, and the eighth was obtained in only 17.5% yield. On the other hand, the synthesis of 3H-imidazo[4.5-c]pyridine was investigated as follows. Benzylation of the 4,5-dibromoimidazole 113 with benzyl bromide or 4-acetoxybenzyl bromide gave the N-benzylimidazoles, which were treated with n-BuLi followed by quenching with DMF to yield the 5-formylimidazoles 114, regioselectively.
The Pd-catalyzed cross-coupling reaction11 of 114 with alkenyl(tributyl)tin (ethenyl or isopropenyl) afforded the 4-alkenylimidazoles 115, which were treated with hydroxylamine to give oximes 116. Four types of oximes 116 were subjected to a thermal electrocyclic reaction to provide 3H-imidazo[4,5-c]pyridines 117. In addition, a thermal electrocyclic reaction using the oxime methyl ether was also investigated (72-75% yields). There was almost no difference in this case.29
III-1-2. Synthesis of the imidazo[4,5-c]quinoline imiquimod
Imiquimod (127), 4-amino-1-isobutyl-1H-imidazo[4,5-c]quinoline, is a potent inducer of interferon-α in many animal species, as well as humans, and is a potent antiviral and antitumor agent.30 2,4,5-Tribromoimidazole (118) was alkylated with isobutyl bromide to give the isobutylimidazole 119, which was converted to the methylsulfanylimidazole 120 by treatment with n-BuLi, followed by the addition of MeSSMe. Treatment of 120 with t-BuLi and subsequent addition of cyclohexanone
regioselectively produced the cyclohexyl alcohol 121. Heating 121 in the presence of p-TsOH yielded the cyclohexenylimidazole 122, which was then treated with n-BuLi, followed by the introduction of CO2 gas to afford the corresponding carboxylic acid 123. Reaction of 123 with hydroxylamine O-methyl ether, followed by the addition of PPh3-CCl4 gave the α-chloro-oxime ether 124, with an aza 6π-electron system. The electrocyclic reaction of 124 was carried out in o-dichlorobenzene, yielding cyclyzed tetrahydroimidazo[4.5-c]quinoline 125, which was treated with DDQ to provide the imidazo[4,5-c]pyridine 126. Finally, treatment of 126 with conc. NH4OH in a sealed tube followed by desulfurization with Red-Al afforded imiquimod (127) (Scheme 14).31
III-1-3. Synthesis of the β-carboline alkaloids oxopropalines G and D
The novel cytocidal β-carbolines, oxopropalines D (141b) and G (137) were isolated from Streptomyces sp. G324 together with three related oxopropalines,32 which produces lavendamycin. Initially, 1-methoxycarbonyl-4-methyl-β-carboline (134) was synthesized as a common key compound (Scheme 15). The Pd-catalyzed cross-coupling reaction11 between 3-iodoindole-2-carbaldehyde (128) and isopropenyl tributylstannane gave the isopropenylindole 129. The N-protection of 129 with MOMCl followed by treatment with hydroxylamine produced the oxime 130, which was subjected to a thermal electrocyclic reaction to yield the β-carboline nucleus 131. Treatment of 131 with mCPBA followed by heating in Ac2O yielded the 1-hydroxy-β-carboline 132, which was treated with Tf2O to furnish the triflate 133. Methoxycarbonylation of 133 with CO and MeOH in the presence of Pd(OAc)2 and 1,1’-bis(diphenylphosphino)ferrocene (dppf) provided the common key compound 134. Nucleophilic addition of 134 with the acetate carbanion afforded the N-MOM-β-keto ester 135. The N-MOM deprotected β-carboline 136 was successfully obtained by heating in MeOH, CH(OMe)3, CF3SO3H, and MeNO2.33 Reduction of 136 with diborane followed by selective oxidation of the resulting 1,3-diol with act. MnO2 gave oxopropaline G (137). 34a,b
Furthermore, the synthesis of oxopropaline D (141b) was attempted using 134 (Scheme 16). The N-MOM carbazole 134 was converted to 135 using the our procedure.33 Reduction of 135 with DIBAL-H afforded the aldehyde, which was treated with vinylmagnesium bromide followed by silylation of the resulting alcohol 136 with TBDMSCl to obtain the allyl silyl ether 137. The allyl ether 137 was treated with AD-mix-α using the Sharpless oxidation35 to produce the 1,2-diol 138, which was then converted to the acetonide 139 along with its desilylated acetonide 140. Compound 139 was converted to 140 with TBAF. Oxidation of the alcohol 140 with act. MnO2, followed by treatment of the resulting ketone with dil. H2SO4 provided (-)-oxopropaline D (141a). On the other hand, the allyl ether 137 was oxidized with AD-mix-β to produce the other 1,2-diol 142, which was converted to the acetonide 143. Similarly, removal of the TBDMS group of 143 followed by oxidation of the alcohol furnished the ketone 145. The keto-acetonide 145 was treated with dil. H2SO4 to give (+)-oxopropaline D (141b). The specific rotation of 141b showed the same direction of rotation and
approximate value as that of the natural product.34a,b
To determine the absolute configuration of 145 and 141b, treatment of the 1-bromo-β-carboline 146, derived from 132, with [(Me)3Si]2NK and n-BuLi followed by the reaction with (R)-glyceraldehyde acetonide produced the alcohol 147, which was oxidized with act. MnO2 to yield (R)-(+)-oxopropaline D acetonide 148. The HPLC data and the specific rotation of 148 were identical with those of synthetic 145. The absolute stereochemistry of the C-11 position of natural (+)-oxopropaline D (141b) was determined to be the R-configuration.34c
III-1-4. Synthesis of β-carboline alkaloids, (R)-(-)-pyridindolols
Pyridindolol (161) was initially isolated from Streptomyces alboverticillatus as a β-galactosidase inhibitor.36a Three pyridindolols containing the glucoside were also isolated from Streptomyces parvulus, strain Tu2480.36b Furthermore, pyridindolols K1 (159) and K2 (158a) were isolated from Streptomyces sp. K93-0711 together with pyridindolol (161). The stereochemistry of the C-14 position of all pyridindolols was reported to be in an R-configuration.36c The Pd-catalyzed cross-coupling reaction11 of N-MOM-3-iodoindole 149 with tributyl[3-(MOMoxy)prop-1-en-1-yl]stannane gave the 3-alkenylindole 150. After the aldehyde 150 was converted to the oxime 151, it was subjected to a thermal electrocyclic reaction to produce the β-carboline 152. Subsequent oxidation of 152 with mCPBA afforded the β-carboline N-oxide 153. Synthesis of compound 153 was also investigated using Sakamoto’s procedure37 through the ring closure of the 3-alkynylindole-2-aldoxime. Next, heating 153 with Ac2O at reflux temperature, followed by treatment of the resulting 1-hydroxy-β-carboline with Tf2O and pyridine, afforded the triflate 154. The triflate 154 was used in the Pd-catalyzed cross-coupling reaction11 with vinyl tributylstannane to give the 1-ethenyl-β-carboline 155. Deprotection of the N-MOM-group of 155 with our reported procedure33 yielded the ethenyl-β-carboline 156. After acetylation of 156 with Ac2O, the Sharpless asymmetric dihydroxylation35 of 157 with AD-mix-α provided (S)-(+)-pyridindolol K2 (158b). By contrast, the reaction of 157 with AD-mix-β was carried out in a similar way to produce (R)-(-)-pyridindolol K2 (158a). In addition, selective acetylation of 158a afforded (R)-(-)-pyridindolol K1 (159) along with the triacetate 160. On the other hand, hydrolysis of 158a with K2CO3 provided pyridindolol (161). Total syntheses of the three pyridindolols together with (S)-(+)-enantiomer 158b were achieved (Scheme 17).38
III-1-5. Synthesis of the β-carboline alkaloid, dichotomine C
(-)-Dichotomine C (174b) was isolated from Stellaria dichotoma along with five related β-carboline alkaloids. The absolute configuration of the C14 position in dichotomine C (174b) was determined to be the S-configuration.39 The required β-carboline 169 was prepared from 3-iodoindole 149 (Scheme 18). The Heck reaction40 between the iodoindole 149 and methyl acrylate gave the 3-alkenylindole-2-carbaldehyde 167. Subsequent treatment of hydroxylamine produced the oxime 168, which was subjected to a microwave (MW)-assisted thermal electrocyclic reaction to yield the methyl β-carboline-3-carboxylate 169. Subsequently, treatment of 169 with mCPBA followed by chlorination with oxalyl chloride yielded the N-MOM-1-chloro-β-carboline 171. Cleavage of the N-MOM group of 171 with our reported procedure33 afforded the 1-chloro-β-carboline 172. The 1-ethenyl-β-carboline 173 was synthesized from 172 with ethenyl(tributyl)tin by the Stille reaction.11 Finally, asymmetric dihydroxylations35 of 173 with AD-mix-α or AD-mix-β were carried out to provide the corresponding (-)-dichotomine C (174b) or (+)-dichotomine C (174a), respectively,41 contrary to the Sharpless rule.35
III-1-6. Synthesis of isoquinoline-5,8-quinone alkaloids, renierol and related natural products
Renierone (196) was isolated from the major metabolite of Reniera sp.42 Mimocin (200), isolated from a metabolite of Streptomyces lavendulae, contains a pyruvamide in place of the angelate ester side chain of 196.43a Renierol (195) was isolated from the hard blue sponge Xestospongia caycedoi.43b Further studies of the metabolites of Reniera sp. have resulted in the isolation of 7-methoxy-1,6-dimethylisoquinoline-5,8-dione (199),42b,43c which was also found in a blue Phillipin marine sponge of the genus Xestospongia sp.43b In addition, renierol acetate (197) and renierol propionate (198) were isolated from the marine sponge Xestospongia sp. and its associated nudibranch Jorunna funebris.44 For the synthesis of key compound 181 (Scheme 19), treatment of benzaldehyde 175 with BBr3, followed by conversion of the 2-hydroxybenzaldehyde into the benzyl ether, which was subjected to the Baeyer-Villiger reaction to produce the phenol 176. The Duff reaction of 176 followed by treatment of the resulting 2-hydroxybenzaldehyde with Tf2O gave the triflate, which was carried out by the Stille reaction of the triflate with ethenyl tributylstannan to yield the 2-ethenylbenzaldehyde 177. The Grignard reaction of 177 with dimethylisopropylsilylmethylmagnesium chloride, followed by treatment with KF and H2O2, afforded the 1,2-diol 178. Selective protection of 178 with TBDMSCl, followed by oxidation with PCC, gave the ketone, which was treated with hydroxylamine to produce the ketoxime 179. The oxime 179 was subjected to a thermal electrocyclic reaction to furnish the 5-benzyloxyisoquinoline 180. Although the electrocyclic reaction of the highly substituted substrate 179 also proceeded, the yield of 180 was only marginally better than that of the simple 2-alkenylbenzaldoxime.2 Deprotection of the TBDMS group of 180 with TBAF provided the desired 5-benzyloxy-1-hydroxymethylisoquinoline 181 as the common precursor with the appropriate substituents. For the next stage, precursor 181 was converted to the corresponding esters, angelate 183, acetate 184, and propionate 185 by treatment of 181 with PhLi, followed by addition of the mixed anhydride 182, Ac2O, and (EtCO)2O, respectively.
Furthermore, treatment of 181 with PhLi, followed by addition of p-toluenesulfonyl chloride gave the tosylate 189, which was reduced with LiEt3BH to yield the 1-methylisoquinoline 191. In addition, the nucleophilic substitution reaction of 189 with NaN3 produced the azide derivative 192, which was treated with PPh3 in situ, followed by addition of pyruvoyl chloride to furnish the 1-pyruvoylaminomethylisoquinoline 194. Sequential cleavages of the benzyl groups of 181, 184, 185, 190, and 193 were carried out by hydrogenolysis to give the corresponding phenols 182, 187, 188, 191, and 194, respectively. Although debenzylation of 183 with hydrogenolysis failed, cleavage of the ether of 183 was subjected to the Fuji’s procedure45 to successfully produce the phenol 186. At the final stage, the 5-hydroxyisoquinolines 182, 186-188, 191, and 194 were oxidized by salcomine with O2 (or CAN) to provide the corresponding isoquinoline-5,8-quinone alkaloids 195-200 in excellent yields.46
III-1-7. Synthesis of the furo[3,2-h]isoquinoline alkaloids TMC-120B and A
Furo[3,2-h]isoquinoline alkaloid TMC-120B (210b) was isolated from Aspergillus ustus TMC1118, together with TMC-120A (210a) and TMC-120C (210c).47 The aldehyde 202 was prepared in three steps from 2,4-diMOMoxybenzaldehyde (201) (Scheme 20). The subsequent benzaldehyde 203 was synthesized in three steps from 202. The selective cleavage of MOM-ether group of 203 with conc. HCl (one equiv.) afforded the 2-hydroxybenzaldehyde, which was converted to the ether with methyl bromoacetate and NaH. Cleavage of the 4-MOM group of the ether with an aqueous AcOH produced the 4-hydroxybenzaldehyde 204. The phenol 204 was treated with Tf2O, and then the Pd-catalyzed cross-coupling reaction11 of the triflate with tributyl(1-propenyl)tin yielded the 2-alkenylbenzaldoxime 205. The subsequent thermal electrocyclic reaction of the oxime ether 205 was carried out under
both conventional conditions and MW-irradiation to provide the 3,7,8-trisubstituted isoquinoline 206. The MW-irradiation condition was slightly more effective than the conventional condition. The 7-formylisoquinoline 206 was converted to the methyl ester 207, which was cyclized with NaOMe to produce the β-ketoester 208, and then 208 was treated with LiOH to give the furanone 209. The furanone 209 was treated with LDA and acetone, and subsequent treatment with MsCl and DMAP provided TMC-120B (210b). 210b was converted to TMC-120A (210a) with 10% Pd-C and H2.48
III-1-8. Synthesis of the phenanthridine alkaloid trispheridine
The phenanthridine alkaloid trispheridine (216) was found in a member of the Amaryllidaceae plant family.49 The required 2-cyclohexenylaldoxime 214 was prepared as follows (Scheme 21). The Suzuki-Miyaura reaction8 of 2-bromopiperonal (211) with cyclohexenylboronic acid pinacol ester (212) was carried out under conditions of PdCl2(PPh3)2 and NaOMe at 70 oC to give the cyclohexenylbenzaldehyde 213. This reaction was also attempted under MW-irradiation for 5 min to produce 213 in excellent yield. Subsequent treatment of 213 with NH2OMe afforded the required oxime ether 214, which was subjected to a thermal electrocyclic reaction under both MW-assisted and conventional conditions to yield the tetrahydrophenanthridine 215. The yield and reaction rate of this reaction were promoted by the MW irradiation. Finally, heating 215 with 10% Pd-C in Ph2O in the presence of diethyl fumarate provided trispheridine (216).50
III-1-9. Synthesis of benzo[c]phenanthridine alkaloids and structural revision of broussonpapyrine
Benzo[c]phenanthridine alkaloids were isolated from the Rutaceae, Papaveraceae, and Fumariaceae plants.51 We planned a bond formation of C4b and N5 using a microwave-assisted electrocyclic reaction for construction of the tetracyclic benzo[c]phenanthridine nucleus (Scheme 22). The Suzuki-Miyaura reaction8 of 2-bromobenzaldehydes 217, 211, and 218 with the pinacol borate 219 (prepared from 2-allyl-4,5-methylenedioxyphenol in seven steps) proceeded smoothly to give the 2-cycloalkenylbenzaldehydes 220, 221, and deacetylated 222, which were converted to the oxime ethers 223, 224, and 225, respectively. The oxime ethers 223, 224, and acetylated 226 were subjected to a thermal electrocyclic reaction under MW-irradiation to yield the corresponding 11, 12-dihydrobenzo[c]phenanthridines 227, 228, and 229. Finally, the dihydro-compounds 227, 228, and 229 were oxidized by refluxing with 10% Pd-C in o-dichlorobenzene to give nornitidine (230), noravicine (231), and O-acetylisodecarine 232, respectively. Hydrolysis of the acetyl group of 232 with KHCO3 provided isodecarine (233).52a Similarly, norchelerythrine (234), norsanguinarine (235), zanthoxyline (236), and norbroussonpapyrine (237) were also synthesized. Although the structure of synthetic zanthoxyline (236) was consistent with zanthoxyline (236) synthesized previously by the Abe group,53a the structure of the reported zanthoxyline by the Morel group53b was revised to the structure of decarine.53a The structure of the synthetic broussonpapyrine (239) was not consistent with the reported structure 239 by the Qin group.53c The structure of the reported broussonpapyrine (239) was revised to the structure of chelerythrine (238) based on comparison of the 1H- and 13C-NMR spectra.52
III-1-10. Synthesis of the 2-azaanthraquinone alkaloid scorpinone
The 2-azaanthraquinone alkaloid scorpinone (248) was isolated from the mycelium of a Bispora-like tropical fungus.54 The 2-propenylbenzaldoxime 241 was prepared from 2-hydroxy-6-methoxybenzaldehyde (240) in three steps (Scheme 23). Treatment of 240 with Tf2O and pyridine afforded the triflate. The Stille reaction11 of the resulting triflate with tributyl(1-propenyl)tin produced the 2-propenylbenzaldehyde, which was treated with hydroxylamine methyl ether to yield the aldoxime methyl ether 241. A thermal electrocyclic reaction of 241 was carried out in o-dichlorobenzene under MW-irradiation to provide the isoquinoline 242 along with the loss of methanol. This reaction of 241 was also attempted under the conventional conditions, but the isoquinoline 242 was obtained in low yield together with a byproduct. Cleavage of methyl ether of 242 with 48% HBr afforded the 8-hydroxyisoquinoline 243. Bromination of 243 was performed to give the 5,7-dibromoisoquinoline 244, which was immediately oxidized with CAN to yield the isoquinoline-5,8-dione 245. Subsequent Diels-Alder reaction of 245 with the diene 246 gave the known 6-deoxybostrycoidine (247) with the elimination of a methoxy group. The use of MW-irradiation was more effective than the conventional condition for improving the yield and decreasing the reaction time. Alkylation of 247 with MeI and K2CO3 provided scorpinone (248).55
III-2. Synthesis of fused pyridine rings by a thermal electrocyclic reaction of an aza 6π-electron system using an isocyanate
III-2-1. Synthesis of the imidazo[4,5-b]pyridines PhIP and DMIP
Mutagens, 2-amino-1-methyl-6-phenylimidazo[4.5-b]pyridine (PhIP) (254a) and 2-amino-1,6-dimethylimidazo[4,5-b]pyridine (DMIP) (254b) were isolated from cooked beef and fried Norwegian sausage,56 along with the other mutagenic heterocyclic amines. We planned the synthesis of an imidazo[4,5-b]pyridin-5(4H)-one 253 by a thermal electrocyclic reaction of 5-alkenylimidazole-4-isocyanate 252 (Scheme 24), according to the modified Eroy’s pyrido-annulation.57 Treatment of 1-methyl-2,4,5-tribromoimidazole (249) with n-BuLi followed by the addition of dimethyldisulfide gave the 2-methylsulfanylimidazole, which was treated in a similar way with n-BuLi followed by the addition of DMF to yield the 5-formylimidazole 250. The Wittig reaction of 250 with alkyltriphenylphosphorane (R=Ph and Me) produced 5-alkenylimidazoles, and then treatment of 5-alkenylimidazoles with n-BuLi followed by the addition of gaseous CO2 afforded the 4-carboxylic acids 251a and 251b, respectively. Carboxylic acids 251a and 251b were heated in benzene with DPPA and Et3N under the Curtius conditions to give the relatively stable isocyanates 252a and 252b. The isocyanates 252a and 252b were subjected to a thermal electrocyclic reaction to produce the expected pyridones 253a and 253b. Finally, reduction of 253a and 253b with Red-Al followed by replacement of the methylsulfanyl group with NaNH2 provided PhIP (254a) and DMIP (254b), respectively.58
III-2-2. Synthesis of the imidazo-α-carboline alkaloids, grossularines-1 and -2
Grossularines-1 (269) and -2 (267) were isolated from dendrodoa grossularia (Stylidae), a tunicate collected in Britany.59 These antitumor substances are the first example of naturally occurring pyrido[2.3-b]indole. Treatment of the N-SEM-imidazole (255) with t-BuLi, followed by the addition of tributyltin chloride, gave the stannylimidazole 256, which was subjected to the Pd-catalyzed cross-coupling reaction11 to yield the 3-(5-imidazolyl)indole 258. Hydrolysis of 258 with Na2CO3 yielded the carboxylic acid, which was treated with DPPA at 60 oC to provide the isocyanate 259 as the required aza 6π-electron system. The thermal electrocyclic reaction of 259 was carried out at 170 oC for 5 min to produce the tetracyclic pyrido[2,3-b]indole 260. Furthermore, 260 was converted into the triflate 261. The three-component Pd-catalyzed cross-coupling reaction8 between the triflate 261, CO, and 4-MOMoxyphenylboronic acid (262) was carried out in anisole at 80 oC to provide the 2-benzoylpyrido[2,3-b]indole 263 along with the 2-phenylpyrido[2,3-b]indole 264 as a major product (Scheme 25). Subsequent hydrolysis of the N-SEM group of 263 with dil. HCl gave groussularine-2 (267). A similar application of this reaction to the synthesis of groussularine-1 (269), however, failed. In addition, triflate 261 was converted into the methyl esters, either N-deprotected 265a or 265b, by a Pd-catalyzed carbonylation, according to the Ortar’ procedure,60 depending upon the amount of Et3N. The nucleophilic addition to the methyl ester 265a or 265b with phenyllithium 266, followed by hydrolysis with dil. HCl, gave the desired groussularine-2 (267) from both substrates. Furthermore, the reaction to methyl ester 265a or 265b with 3-indolyllithium 268, followed by hydrolysis with dil. HCl, also provided groussularine-1 (269) in a similar way. This approach based on the thermal electrocyclic reaction of an aza 6π-electron system provided an effective route to produce the imidazo[4’,5’:3,4]pyrido[2,3-b]indole nucleus.61
III-2-3. Synthesis of imidazo-α-carbolines through an anomalous Stille reaction
During the course of our studies toward the total synthesis of groussularines-1 (269) and -2 (267),61 we required 3-imidazolylindole 273 for a tetracyclic α-carboline framework (Scheme 26). 5-Stannylimidazole (271) was prepared from 1-ethoxymethyl(EOM)-5-bromo-2-methylsulfanylimidazole (270) by a bromine-lithium exchange reaction with n-BuLi followed by treatment with tributyltin chloride. The Pd-catalyzed cross-coupling reaction11 of 271 with 3-iodoindole 257 was carried out in DMF at 120 oC to tentatively give two separable imidazolylindoles 272 and 273. To determine the structures of 272 and 273, both products independently led to tetracyclic α-carbolines. Hydrolysis of esters 272 and 273 followed by Curtius rearrangement using DPPA yielded isocyanates 275 and 278, which were subjected to thermal electrocyclic reaction to provide α-carbolines 276 and 279, respectively. The two structures were determined by NOE experiments to be 276 and 279, respectively. Thus, this reaction proceeds by way of not only ipso-substitution but also cine-substitution reaction, and seems to be the first example of cine substitution of heteroarylstannane. The Stille reaction of N-SEM-imidazole 256 with 3-iodoindole 257 fortunately proceeded normally, however, to provide the desired product (III-2-2).62
III-2-4. Synthesis of the imidazo[4,5-c]quinoline imiquimod through isocyanate
Imiquimod (127) was also synthesized by constructing the imidazo[4,5-b]quinoline ring 286 based on the thermal electrocyclic reaction through the isocyanate 285 (Scheme 27). Treatment of the 5-bromoimidazole 281 with n-BuLi, followed by the addition of tributyltin chloride gave stannnylimidazole, which was then subjected to a Pd-catalyzed cross-coupling reaction11 with methyl 2-bromobenzoate (280) to yield the 5-phenylimidazole 283. Hydrolysis of the crude 283 with 10% NaOH yielded the carboxylic acid 284. Compound 284 was treated with DPPA at 50 oC in benzene, and then the solvent was replaced with o-dichlorobenzene. The electrocyclic reaction of 285 proceeded smoothly to give imidazo[4,5-b]quinoline 286, which was converted to 4-chloroimidazo[4,5-c]quinoline 126 with POCl3. Compound 126 was consistent with the former 126 synthesized through the oxime’s route (III-1-2). Finally, treatment of conc. NH4OH in a sealed tube followed by the reduction of Red-Al provided imiquimod (127).30
IV. CONCLUSION
As described above, our synthetic strategy was confirmed to be applicable for the construction of a variety of fused hetero-aromatic ring systems including natural products. Based on these results, we are attempting to synthesize a highly substituted carbazole alkaloid by applying the allene-mediated electrocyclic reaction. The development of a synthesis of bioactive fused pyridine ring systems including natural products using a MW-assisted electrocyclic reaction of an aza 6π-electron system is also in progress.
ACKNOWLEDGEMENTS
The research described herein was skillfully completed by the hard work of graduate students, undergraduate students, and research assistants in our laboratory. We thank all of our co-workers, whose names are cited in the references. We are grateful for the partial support for this research provided by a Grant-in Aid for Scientific Research (C) and for Young Scientists (S) of Japan Society for the Promotion of Science (JSPS). We also acknowledge the financial support our studies provided by several pharmaceutical companies and Fukuyama University. We dedicate this review to the late Dr. E. Sugino.
References
1. (a) E. N. Marvel, Thermal Electrocyclic Reactions, Academic Press, New York, 1980, p. 260; (b) F. B. Mallory and C. W. Mallory, Org. React., 1984, 30, 1; (c) W. H. Okamura and A. R. de Lera, In Comprehensive Organic Synthesis, ed. by B. M. Trost, I. Fleming, and L. A. Paquette, Pergamon Press, New York, 1991, Vol. 5, p. 699. CrossRef
2. (a) S. Kano, E. Sugino, and S. Hibino, J. Chem. Soc. Chem. Commun., 1980, 1241; CrossRef (b) S. Kano, E. Sugino, S. Shibuya, and S. Hibino, J. Org. Chem., 1981, 46, 3856; CrossRef (c) S. Hibino, A. Tonari, T. Choshi, and E. Sugino, Heteocycles, 1993, 35, 441; CrossRef (d) S. Kano, E. Sugino, S. Shibuya, and S. Hibino, J. Org. Chem., 1981, 46, 2979; CrossRef (e) S. Kano, E. Sugino, and S. Hibino, Heterocycles, 1982, 19, 1673; CrossRef (f) S. Hibino and E. Sugino, J. Heterocycl. Chem., 1990, 27, 1751; CrossRef (g) S. Hibino, S. Kano, N. Mochizuki, and E. Sugino, J. Org. Chem., 1984, 49, 5006; CrossRef (h) S, Hibino, E, Sugino, T. Kuwada, H. Hashimoto, K. Sato, F. Amanuma, and Y. Karasawa, Chem. Pharm. Bull., 1987, 35, 2261; (i) S. Hibino, E. Sugino, N. Ogura, Y. Shintani, and K. Sato, Heterocycles, 1990, 30, 271; CrossRef (j) S. Hibino, E, Sugino, T. Kuwada, N. Ogura, K. Sato, and T. Choshi, J. Org. Chem., 1993, 57, 5917; CrossRef (k) S. Hibino, E. Sugino, T. Kuwada, N. Ogura, Y. Shintani, and K. Sato, Chem. Pharm. Bull., 1991, 39, 79; (l) S. Hibino, E. Sugino, Y. Adachi, K. Nomi, K. Sato, and K. Fukumoto, Heterocycles, 1989, 28, 275; CrossRef (m) S. Hibino, E. Sugino, T. Choshi, and K. Sato, J. Chem. Soc. Perkin Trans. 1, 1988, 2429; CrossRef (n) E. Sugino, T. Choshi, and S. Hibino, Heterocycles, 1999, 50, 543. CrossRef
3. (a) S. Hibino and E. Sugino, In Advances in Nitrogen Heterocycles, ed. by C. J. Moody, JAI Press, Greenwich, CT, 1995, Vol. 1, p. 205; (b) T. Kawasaki and M. Sakamoto, J. Indian Chem. Soc., 1994, 71, 443; (c) T. Choshi, Yakugaku Zasshi, 2001, 121, 487; CrossRef (d) H.-J. Knölker, Chem. Rev., 2002, 102, 4303; CrossRef (e) H.-J. Knölker and K. R. Reddy, In The Alkaloids, ed. by G. A. Cordell, Academic Press, Amsterdam, 2008, Vol. 65, p. 1.
4. S. Kato, H. Kawai, T. Kawasaki, Y. Toda, T. Urata, and Y. Hayakawa, J. Antibiot., 1989, 42, 1879.
5. J. H. Cardellina, M. P. Kirkup, R. E. Moore, J. S. Mynderse, K. Seff, and C. J. Simmons, Tetrahedron Lett., 1979, 4915. CrossRef
6. M. Tanaka, K. Shin-ya, K. Furihata, K. Furihata, and H. Seto, J. Antibiot., 1995, 48, 326.
7. (a) K. Hayakawa, S. Ohsuki, and K. Kanematsu, Tetrahedron Lett., 1986, 27, 4205; CrossRef (b) S. Nagashima and K. Kanematsu, Tetrahedron Asymmetry, 1990, 1, 743. CrossRef
8. (a) N. Miyaura and A. Suzuki, Chem. Rev., 1995, 95, 2457; CrossRef (b) N. Miyaura, Top. Curr. Chem., 2002, 219, 11. CrossRef
9. (a) T. Choshi, T. Sada, H. Fujimoto, C. Nagayama, E. Sugino, and S. Hibino, Tetrahedron Lett., 1996, 37, 2593; CrossRef (b) T. Choshi, T. Sada, H. Fujimoto, C. Nagayama, E. Sugino, and S. Hibino, J. Org. Chem., 1997, 62, 2535. CrossRef
10. (a) C. J. Mo, K. Shin-ya, K. Furihata, S. Shimizu, Y. Hayakawa, and H. Seto, J. Antibioti., 1990, 43, 1337; (b) M. Tanaka, K. Shin-ya, K. Furihata, and H. Seto, J. Antibiot., 1995, 48, 326.
11. (a) J. K. Stille, Angew. Chem. Int. Ed. Engl., 1986, 25, 508; CrossRef (b) T. N. Mitchell, Synthesis, 1992, 803; CrossRef (c) V. Farina, V. Krishnamurthy, and W. J. Scott, Org. React., 1998, 50, 1.
12. T. Choshi, H. Fujimoto, E. Sugino, and S. Hibino, Heterocycles, 1996, 43, 1847. CrossRef
13. M. Kaneda, T. Naid, T. Kitahara, and S. Nakamura, J. Antibiot., 1988, 41, 602.
14. H. Hagiwara, T. Choshi, H. Fujimoto, E. Sugino, and S. Hibino, Tetrahedron, 2000, 56, 5807. CrossRef
15. (a) K. Shin-ya, M. Tanaka, K. Furihata, Y. Hayakawa, and H. Seto, Tetrahedron Lett., 1993, 34, 4943; CrossRef (b) K. Shin-ya, S. Shimizu, T. Kunigami, K. Furihata, K. Furihata, and H. Seto, J. Antibiot., 1995, 48, 574.
16. T. Choshi, Y. Uchida, Y. Kubota, J. Nobuhiro, M. Takeshita, T. Hatano, and S. Hibino, Chem. Pharm. Bull., 2007, 55, 1060. CrossRef
17. (a) B. K. Chowdhury and D. P. Chakraborty, Chem. Ind. (London), 1969, 549; (b) B. K. Chowdhury and D. P. Chakraborty, Phytochemistry, 1971, 10, 481; CrossRef (c) D. P. Chakraborty, P. Bhattacharyya, S. Roy, S. P. Bhattacharyya, and A. K. Biswas, Phytochemistry, 1978, 17, 834; CrossRef (d) T.-S. Wu, S. C. Huang, P.-L. Wu, and C.-M. Teng, Phytochemistry, 1978, 17, 834; (e) T.-S.Wu, S.-C. Huang, P.-L. Wu, and C.-S. Kuoh, Phytochemistry, 1999, 52, 523; CrossRef (f) C. Ito, S. Katsuno, H. Ohta, M. Omura, I. Kajiura, and H. Furukawa, Chem. Pharm. Bull., 1997, 45, 48; (g) C. Ito, S. Katsuno, M. Itoigawa, N. Ruangrungsi, T. Mukainaka, M. Okuda, Y. Kitagawa, H. Tokuda, H. Nishino, and H. Furukawa, J. Nat. Prod., 2000, 63, 125. CrossRef
18. S. Tohyama, T. Choshi, S. Azuma, H. Fujioka, and S. Hibino, Heterocycles, 2009, 79, 955. CrossRef
19. N. Kotoda, K. Shinya, K. Furihata, Y. Hayakawa, and H. Seto, J. Antibiot., 1997, 50, 770.
20. (a) D. E. van Horn and E. Negishi, J. Am. Chem. Soc., 1978, 100, 2252; CrossRef (b) E. Negishi, D. E. van Horn, A. O. King, and N. Okukado, Synthesis, 1979, 501; CrossRef (c) C. L. Rand, D. E. van Horn, M. W. Moore, and E. Negishi, J. Org. Chem., 1981, 46, 4093; CrossRef (d) D. E. van Horn, E. Negishi, and T. Yoshida, J. Am. Chem. Soc., 1985, 107, 6639. CrossRef
21. Y. Hieda, T. Choshi, S. Kishida, H. Fujioka, and S. Hibino, Tetrahedron Lett., 2010, 51, 3593. CrossRef
22. (a) T.-S. Wu, T. Ohta, and H. Furukawa, Heterocycles, 1983, 20, 1267; CrossRef (b) H. Furukawa, T.-S. Wu, T. Ohta, and C.-S. Kuoh, Chem. Pharm. Bull., 1985, 33, 4132.
23. (a) H. Hagiwara, T. Choshi, H. Fujimoto, E. Sugino, and S. Hibino, Chem. Pharm. Bull., 1998, 46, 1948; (b) H. Hagiwara, T. Choshi, J. Nobuhiro, H. Fujimoto, and S. Hibino, Chem. Pharm. Bull., 2001, 49, 881. CrossRef
24. C. Ito and H. Furukawa, Chem. Pharm. Bull., 1990, 38, 1548.
25. R. W. Rickards, J. M. Rothschild, A. C. Willis, N. M. de Chazel, J. Kirk, K. Kirk, K. J. Saliba, and G. D. Smith, Tetrahedron, 1999, 55, 13513. CrossRef
26. (a) S. Tohyama, T. Choshi, K. Matsumoto, A. Yamabuki, K. Ikegata, J. Nobuhiro, and S. Hibino, Tetrahedron Lett., 2005, 46, 5263; CrossRef (b) A. Yamabuki, H. Fujinawa, T. Choshi, S. Tohyama, K. Matsumoto, K. Ohmura, J. Nobuhiro, and S. Hibino, Tetrahedron Lett., 2006, 47, 5859; CrossRef (c) S. Tohyama, T. Choshi, K. Matsumoto, A. Yamabuki, Y. Hieda, J. Nobuhiro, and S. Hibino, Heterocycles, 2010, 82, 397; CrossRef (d) T. Choshi and S. Hibino, Heterocycles, 2009, 77, 85. CrossRef
27. Y. Fukuyama, C. Iwasaki, M. Kodama, M. Ochi, K. Kataoka, and K. Shibata, Tetrahedon, 1998, 54, 10007. CrossRef
28. (a) M. Hirayama, T. Choshi, T. Kumemura, S. Tohyama, J. Nobuhiro, and S. Hibino, Heterocycles, 2004, 63, 1765; CrossRef (b) J. Nobuhiro, M. Hirayama, T. Choshi, K. Kamoshita, S. Maruyama, Y. Sukenaga, T. Ishizu, H. Fujioka, and S. Hibino, Heterocycles, 2006, 70, 491. CrossRef
29. H. Yoshioka, T. Choshi, E. Sugino, and S. Hibino, Heterocycles, 1995, 41, 161. CrossRef
30. (a) J. F. Gerster, Patent US 553157, 1981 (Chem. Abstr., 1985, 103, 196090s); (b) M. Chen, B. P. Griffith, H. Lucia, and G. D. Hsiung, Antimicrob. Agents Chemother., 1988, 32, 678; (c) C. J. Harrison, L. Jenski, T. Voychehovsky, and D. L. Bernstein, Antiviral Res., 1988, 10, 209. CrossRef
31. H. Yoshioka, Y. Matsuya, T. Choshi, E. Sugino, and S. Hibino, Chem. Pharm. Bull., 1996, 44, 709.
32. (a) N. Abe, Y. Nakakita, T. Nakamura, N. Enoki, H. Uchida, S. Takeo, and M. Munekata, J. Antibiot., 1993, 46, 1672; (b) N. Abe, N. Enoki, Y. Nakakita, H. Uchida, T. Nakamura, and M. Munekata, J. Antibiot., 1993, 46, 1678.
33. T. Kuwada, M. Fukui, M. Hirayama, J. Nobuhiro, T. Choshi, and S. Hibino, Heterocycles, 2002, 58, 325. CrossRef
34. (a) T. Choshi, Y. Matsuya, M. Okita, K. Inada, E. Sugino, and S. Hibino, Tetrahedron Lett., 1998, 39, 2341; CrossRef (b) T. Choshi, T. Kuwada, M. Fukui, Y. Matsuya, E. Sugino, and S. Hibino, Chem. Pharm. Bull., 2000, 48, 108; (c) T. Kuwada, M. Fukui, T. Hata, T. Choshi, J. Nobuhiro, Y. Ono, and S. Hibino, Chem. Pharm. Bull., 2003, 51, 20. CrossRef
35. (a) K. B. Sharpless, W. Amberg, Y. L. Bennami, G. A. Cripino, J. Hartung, K.-S. Jeoung, H.-L. Kwong, K. Morikawa, Z.-M. Wang, D. Xu, and X.-L. Zhang, J. Org. Chem., 1992, 57, 2768; CrossRef (b) S. C. Shinha, A. Ainha-Bagehi, and E. Keinami, J. Org. Chem., 1993, 58, 7789. CrossRef
36. (a) T. Aoyagi, M. Kumagai, T. Hazato, M. Hamada, T. Takeuchi, and H. Umezawa, J. Antibiot., 1975, 28, 555; (b) H. Naganawa, T. Aoyagi, H. Umezawa, H. Nakamura, and Y. Iitaka, J. Antibiot., 1975, 28, 696; (c) M. Kumagai, T. Aoyagi, and H. Umezawa, J. Antibiot., 1976, 29, 696; (d) L. Hagem and W. Keller-Schrierlein, J. Antibiot., 1988, 41, 289; (e) Y.-P. Kim, S. Takamatsu, M. Hayashi, K. Komiyama, and S. Omura, J. Antibiot., 1997, 50, 189.
37. (a) T. Sakamoto, Y. Kondo, N. Miura, K. Hayashi, and H. Yamanaka, Heterocycles, 1986, 24, 2311; CrossRef (b) T. Sakamoto, A. Numata, H. Saitoh, and Y. Kondo, Chem. Pharm. Bull., 1999, 47, 1740; (c) T. Sakamoto, A. Numata, and Y. Kondo, Chem. Pharm. Bull., 2000, 48, 669.
38. (a) N. Kanekiyo, T. Choshi, T. Kuwada, E. Sugino, and S. Hibino, Heterocycles, 2000, 53, 1877; CrossRef (b) N. Kanekiyo, T. Kuwada, T. Choshi, J. Nobuhiro, and S. Hibino, J. Org. Chem., 2001, 66, 8793. CrossRef
39. B. Sun, T. Morikawa, H. Matsuda, S. Tewtrakul, L. J. Wu, S. Harima, and M. Yoshikawa, J. Nat. Prod., 2004, 67, 1464. CrossRef
40. R. F. Heck, Org. React., 1982, 58, 345.
41. K. Omura, T. Choshi, S. Watanabe, Y. Satoh, J. Nobuhiro, and S. Hibino, Chem. Pharm. Bull., 2008, 56, 237. CrossRef
42. (a) D. E. McIntire, D. J. Faulkner, D. V. Engen, and J. Clardy, Tetrahedron Lett., 1979, 20, 4163; CrossRef (b) J. M. Flinke, J. Am. Chem. Soc., 1982, 104, 265. CrossRef
43. (a) A. Kubo, S. Nakahara, R. Iwata, K. Takahashi, and T. Arai, Tetrahedron Lett., 1980, 21, 3207; CrossRef (b) R. A. Edrada, P. Proksch, V. Wray, R. Christ, L. Witte, and R. W. M. van Soest, J. Nat. Prod., 1996, 59, 973; CrossRef (c) T. McKee and C. M. Ireland, J. Nat. Prod., 1987, 50, 754. CrossRef
44. Y. Kitahara, S. Nakahara, R. Numata, K. Inaba, and A. Kubo, Chem. Pharm. Bull., 1985, 33, 823.
45. (a) K. Fuji, T. Kawabata, and E. Fujita, Chem. Pharm. Bull., 1980, 28, 3662; (b) Y. Miki, R. Fujita, and K. Matshushita, J. Chem. Soc. Perkin Trans. 1, 1998, 2533. CrossRef
46. (a) N. Kuwabara, H. Hayashi, N. Hiramatsu, T. Choshi, E. Sugino, and S. Hibino, Chem. Pharm. Bull., 1998, 47, 1805; (b) N. Kuwabara, H. Hayashi, N. Hiramatsu, T. Choshi, T. Kumemura, J. Nobuhiro, and S. Hibino, Tetrahedron, 2004, 60, 2943. CrossRef
47. (a) J. Kohno, H. Hiramatsu, M. Nishio, M. Sakurai, T. Okuda, and S. Komatsubara, Tetrahedron, 1999, 55, 11247; CrossRef (b) J. Kohno, M. Sakurai, N. Kameda, M. Nishio, K. Kawano, N. Kishi, and S. Komatsubara, J. Antibiot., 1999, 52, 913.
48. (a) T. Kumemura, T. Choshi, A. Hirata, M. Sera, Y. Takahashi, J. Nobuhiro, and S. Hibino, Heterocycles, 2003, 61, 13; CrossRef (b) T. Kumemura, T. Choshi, A. Hirata, M. Sera, Y. Takahashi, J. Nobuhiro, and S. Hibino, Chem. Pharm. Bull., 2005, 53, 393. CrossRef
49. (a) S. Ghosal, K. S. Saini, S. Razdan, and Y. Kumar, J. Chem. Res. (S), 1985, 100; (b) A. A. Ali, H. M. El Sayed, O. M. Abdallah, and W. Steglich, Phytochemistry, 1986, 25, 2399; CrossRef (c) R. Suau, A. I. Gomez, and R. Rico, Phytochemistry, 1990, 29, 1710; CrossRef (d) F. Vildomat, M. Selles, C. Codina, and J. Bastida, Planta Med., 1997, 63, 583. CrossRef
50. T. Kumemura, T. Choshi, J. Yukawa, A. Hirose, J. Nobuhiro, and S. Hibino, Heterocycles, 2005, 66, 87. CrossRef
51. (a) H. Ishii and T. Ishikawa, Yakugaku Zasshi, 1981, 101, 663; (b) B. D. Krane, M. O. Fagbule, and M. Shamma, J. Nat. Prod., 1984, 47, 1; CrossRef (c) V. Simanek, In The Alkaloids, ed. by A. Brossi, Academic Press, New York, 1985, Vol. 26, p. 185; (d) M. Suffness and G. A. Cordell, In The Alkaloids, ed. by A. Brossi, Academic Press, New York, 1985, Vol. 26, p. 178; (e) I. Ninomiya and T. Naito, Recent Dev. Chem. Nat. Carbon Comp., 1984, 10, 9; (f) J. Dostal and M. Potacek, Collect. Czech. Chem. Commun., 1990, 55, 2840; CrossRef (g) M. Hanaoka, In The Alkaloids, ed. by A. Brossi, Academic Press, New York, 1988, Vol. 33, p. 141; (h) S. P. MacKey, O. Meth-Cohn, and R. D. Waigh, Adv. Heterocyclic Chem., 1996, 67, 345. CrossRef
52. (a) K. Kohno, S. Azuma, T. Choshi, J. Nobuhiro, and S. Hibino, Tetrahedron Lett., 2009, 50, 590; CrossRef (b) Y. Ishihara, S. Azuma, T. Choshi, K. Kohno, K. Ono, H. Tsutsumi, T. Ishizu, and S. Hibino, Tetrahedron, 2011, 67, 1320. CrossRef
53. (a) H. Abe, N. Kobayashi, Y. Takeuchi, and T. Harayama, Heterocycles, 2010, 80, 873; CrossRef (b) N. F. DeMoura, H. B. Ribeiro, E. C. S. Machado, E. M. Ethur, N. Zanatta, and A. D. Morel, Phytochemistry, 1997, 46, 1443; CrossRef (c) S.-Q. Pang, G.-Q. Wang, B.-K. Huang, Q.-Y. Zhang, and L.-P. Qin, Chem. Nat. Compds., 2007, 43, 100. CrossRef
54. A. Miljkovic, P. G. Mantle, D. J. Williams, and B. Rassing, J. Nat. Prod., 2001, 64, 1251. CrossRef
55. T. Choshi, T. Kumemura, J. Nobuhiro, and S. Hibino, Tetrahedron Lett., 2008, 49, 3725. CrossRef
56. (a) Y. Hashimoto, K. Shudo, and T. Okamoto, Acc. Chem. Res., 1984, 17, 403; CrossRef (b) T. Sugimura, Science, 1986, 233, 312; CrossRef (c) J. S. Felton, M. G. Knize, N. H. Shen, P. R. Lewis, B. D. Andresen, J. Happe, and F. T. Hatch, Carcinogenesis, 1986, 7, 1081; CrossRef (d) M. G. Knize and J. S. Felton, Heterocycles, 1986, 24, 1815. CrossRef
57. (a) F. Eroy and A. Deryckere, Helv. Chim. Acta, 1969, 52, 1755; CrossRef (b) F. Eroy and A. Deryckere, Helv. Chim. Acta, 1970, 53, 645; CrossRef (c) F. Eroy and A. Deryckere, Bull. Soc. Chim. Belg., 1970, 79, 301; (d) F. Eroy and A. Deryckere, J. Heterocycl. Chem., 1970, 7, 1191. CrossRef
58. T. Choshi, A. Tonari, H. Yoshioka, K. Harada, E. Sugino, and S. Hibino, J. Org. Chem., 1993, 58, 7952. CrossRef
59. (a) C. Moquin-Pattey and M. Guyot, Tetrahedron, 1989, 45, 3445; CrossRef (b) D. Carre, C. Moquin-Pattey, and M. Guyot, Acta Crystallogr., 1986, C42, 483.
60. S. Cacci, P. G. Ciatini, E. Morera, and G. Ortar, Tetrahedron Lett., 1986, 27, 3931. CrossRef
61. (a) T. Choshi, S. Yamada, E. Sugino, T. Kuwada, and S. Hibino, Synlett, 1995, 147; CrossRef (b) T. Choshi, S. Yamada, E. Sugino, T. Kuwada, and S. Hibino, J. Org. Chem., 1995, 60, 5899. CrossRef
62. T. Choshi, S. Yamada, J. Nobuhiro, Y. Mihara, E. Sugino, and S. Hibino, Heterocycles, 1998, 48, 11. CrossRef