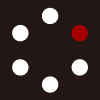
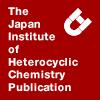
HETEROCYCLES
An International Journal for Reviews and Communications in Heterocyclic ChemistryWeb Edition ISSN: 1881-0942
Published online by The Japan Institute of Heterocyclic Chemistry
e-Journal
Full Text HTML
Received, 2nd March, 2011, Accepted, 14th April, 2011, Published online, 22nd April, 2011.
DOI: 10.3987/COM-11-S(P)5
■ Mechanistic Investigations of the Cyclocondensation Step of the Knorr Pyrrole Synthesis
Scott A. Snyder,* Ferenc Kontes, and Adel M. ElSohly
Department of Chemistry, Columbia University, 3000 Broadway, New York, NY 10027, U.S.A.
Abstract
A mechanistic investigation of the cyclocondensation step of the Knorr pyrrole synthesis of pyrrolo[2,1,5-cd]indolizidines is reported. Kinetic isotope effect measurements suggest the participation of two protic solvent molecules in a rate-determining ketone protonation prior to cyclization and dehydration.Although the pyrrole ring system is a prominent and widely-occurring motif within biologically-active natural products, pharmaceutical agents, and other useful synthetic materials (such as 1–3, Figure 1),1,2 their preparation, particularly when fully substituted, can provide a high challenge for synthetic chemistry. As a result, many methods exist for the preparation of this heterocycle,3 one of the earliest being the classic Knorr pyrrole synthesis first described in 1884. As shown in Scheme 1, this sequence involves a cyclocondensation reaction of appropriate acyclic starting materials.4
This process typically condenses an α-aminoketone (5) with a β-ketoester (6) or a related molecule to form an α-enamino ketone intermediate (7) that then spontaneously cyclizes under the reaction conditions to form a pyrrole (8). Due to its propensity to undergo self-condensation, the α-aminoketone component (5) is generally prepared in situ by one of a variety of methods, such as the reduction of a hydroxime (4).3 We recently employed an intramolecular variant of the Knorr pyrrole synthesis in a total synthesis of the monomeric pyrroloindolizidine myrmicarin alkaloids (9–12, Scheme 2).5,6 In this work, disubstituted piperidine derivative 14 was prepared in eight steps from pyridine. Following N-Boc deprotection and basic work-up, the secondary amine underwent an intramolecular condensation to quantitatively generate dienamine 15. Contrary to reports on related monoenamine compounds,6 this material (15) demonstrated remarkable configurational and chemical stability in aprotic solvents; however, a simple switch to a protic solvent effected a quantitative cyclodehydration to afford myrmicarin 215B (10). This ability to arrest the sequence after enamine condensation and then promote the cyclodehydration by switching solvent was critical in achieving the synthesis of the all-trans upper half of the cyclopentane core of myrmicarin 430A (13). Additionally, this unexpected stability provided the opportunity to explore the mechanism of the cyclodehydration step of the Knorr pyrrole synthesis since it provided an isolable variant of 7 (cf. Scheme 1); that work is the subject of this communication.
The generally accepted mechanism for the cyclization step of the Knorr pyrrole synthesis applied to the myrmicarin system would involve an initial 5-(enol-endo)-exo-trig cyclization of 15 to form 17 (Scheme 3, Path A).7 Intriguingly, though this pathway is formally disfavored by Baldwin’s rules, it is the preferentially invoked mechanism even though no conclusive evidence exists in its support.8,9 A mechanistic alternative, one based on literature reports involving the electrocyclization of related systems,10 would be for a 6π-electrocyclization to effect critical bond constructions; in this paradigm, 15 would first tautomerize to 21, thereby enabling a subsequent cyclization generating azomethine ylide 22. A final loss of a molecule of water would then generate the pyrrole (10, Path B).
Noting that the starting C-5 proton within dienamine 15 underwent neither exchange nor epimerization in deuterated protic solvents, deuterium labeling at that position would allow us to measure a kinetic isotope effect (KIE) during the cyclodehydration reaction. Thus, we prepared deuterated homolog 1611 to resolve this mechanistic quandary. Our expectation was that if Path A were operative, an inverse secondary KIE would be observed, consistent with a decrease in hyperconjugation in the transition state and a consequent strengthening of the C-5 C–H(D) bond.12 On the other hand, if Path B were operative, a primary normal KIE would be observed, given that no exchange occurs at C-5 in deuterated protic solvents and the deprotonation at the α-position of a ketone is typically the slow step during acid-catalyzed enol formation.13
We began our mechanistic probes by obtaining rate constants (kobs) from 1H NMR experiments for the cyclization of 15 and 16. Initial experiments in deuterated isopropanol exhibited excellent fits to simple first-order rate equations, and a normal secondary β KIE was measured (kH/kD = 1.23, Figure 2). This finding is consistent with a weakening of the C–H(D) bond in the transition state due to increased hyperconjugation with an increasingly electron deficient sp2 carbon atom of the ketone. Therefore, based on these results, direct attack of the enamine on the unprotonated ketone (Path A, Scheme 3) is unlikely, as that pathway would lead to a strengthening of the C–H(D) bond from a decrease in hyperconjugation and, therefore, an inverse secondary KIE would result.12 Additionally, the 6π-electrocyclization mechanism (Path B, Scheme 3) is also improbable given that that pathway would involve a slow deprotonation at C-5 and afford a primary KIE.13 On the other hand, the observed KIE is consistent with a rate-determining step involving protonation of the ketone to form an oxocarbenium ion prior to enamine attack. Additionally, this result suggested that the observed rate constants above were actually pseudo-first order and that solvent molecules were likely involved in the transition state of the protonation step.
In order to probe this hypothesis, a series of rate measurements in DMSO-d6/D2O were undertaken. This solvent combination has been previously used to analyze the effect of the concentration of water on the observed rate in other pseudo-first order processes.14 As shown in Figure 3, the use of this solvent system also resulted in a normal secondary β kinetic isotope effect (kH/kD = 1.11 ± 0.05, n = 3), suggesting that the cyclization is occurring via the same mechanism. Thus, the pseudo-first order rate constant (kobs) of the cyclization of 15 was determined at various concentrations of D2O in DMSO-d6. The rate of cyclization of 15 increased with increasing [D2O], and a plot of kobs vs. [D2O] (Figure 4a) displayed significant polynomial character. A plot of kobs/[D2O] vs. [D2O] (Figure 4b), on the other hand, was linear with a non-zero slope indicating that the rate-determining protonation step is second order in water; that is, two water molecules in addition to those involved in solvation are required in the course of the rate-determining step of this cyclization.
Overall, these results are consistent with the experimental observation that the cyclization of 15 does not readily occur in aprotic solvents. Indeed, the zwitterionic intermediate 17 that would result from direct attack of the enamine β-carbon on the unprotonated ketone is likely a very unstable intermediate, especially in a non-polar medium. This statement is supported by semi-empirical (PM3) theoretical calculations in which we were unable to locate 17 as a stationary point on the potential energy surface. Secondly, without the presence of an excess proton source in a polar environment (i.e. a protic solvent), a stabilized oxocarbenium likely cannot form. Furthermore, the basicity of the enamine precludes the use of acids to promote this cyclization, a finding that was confirmed by our own experiments showing that 15 does not cyclize cleanly under acidic conditions5 as well as a previous study that has determined the optimal pH for cyclization to be near neutrality (pH = 6.9).15 Nonetheless, our results cannot rule out a 6π-electrocyclization mechanism in which the rate-determining step is protonation of the ketone prior to enol formation. However, the protonation of the ketone in enol formation is not typically rate-determining.13 Moreover, our semi-empirical (PM3) calculations indicate that the proposed enol tautomer 21 (cf. Scheme 3) is a prohibitively high-energy intermediate, likely due to the placement of five contiguous sp2 atoms in a highly rigid and constrained ring system. Collectively, our experimental and theoretical findings lead us to propose the revised mechanism for the cyclization of 15 shown in Scheme 4. In this sequence, two water molecules participate in a slow protonation of its ketone to afford intermediate 19, which then undergoes a fast cyclization to afford iminium 23 followed by a fast tautomerization and loss of a molecule of water to afford myrmicarin 215B (10).
Finally, the scope of this cyclization to produce various pyrrolo[2,1,5-cd]indolizidines was examined (see Scheme 5). Mono- and dienamines as well as a vinylogous amide readily cyclized to afford products 9, 11, 24 and 25 in good to quantitative yields and short reaction times in MeOH at ambient temperature. Furthermore, it was also possible to effect N-Boc deprotection and double cyclization of piperidine derivative 14 in one pot to afford the pyrroloindolizidine 10 by simply quenching the N-Boc deprotection reaction with basic MeOH, albeit in somewhat reduced yield (71%, Scheme 2, bottom portion).
In summary, we have completed a detailed mechanistic study of the Knorr pyrrole cyclization of indolizidine-based dienamine 15. Based on the results from kinetics experiments, we have proposed a revised mechanism for the cyclization step in which a slow ketone protonation step precedes the 5-(enol-endo)-exo-trig cyclization and dehydration steps. We have also determined that this protonation only occurs in polar protic solvents and that under aqueous conditions, two water molecules participate in this event.
ACKNOWLEDGEMENTS
We thank Professor Jack R. Norton for helpful discussions. Financial support for this work comes from Columbia University, the Research Corporation for Science Advancement (Cottrell Scholar Award to S.A.S.), Eli Lilly (New Faculty and Grantee Awards to S.A.S.), Bristol-Myers Squibb (Unrestricted Grant to S.A.S.), and the Alfred P. Sloan Foundation (Research Fellowship to S.A.S.). Fellowship support from Bristol-Myers Squibb (F.K.), the National Science Foundation (A.M.E.), and the Paul and Daisy Soros Foundation (A.M.E.) is gratefully acknowledged.
EXPERIMENTAL
General Procedures. All reactions were carried out under an argon atmosphere with dry solvents under anhydrous conditions, unless otherwise noted. Dry benzene and methylene chloride (CH2Cl2) were obtained by passing commercially available, pre-dried, oxygen-free formulations through activated alumina columns. Yields refer to chromatographically and spectroscopically (1H and 13C NMR) homogeneous materials, unless otherwise stated. Reagents were purchased at the highest commercial quality and used without further purification, unless otherwise stated. Reactions were magnetically stirred and monitored by thin-layer chromatography (TLC) carried out on 0.25 mm E. Merck silica gel plates (60F-254) using UV light as visualizing agent and cerium sulfate (CAM) or aqueous KMnO4, and heat as developing agents. SiliCycle® silica gel (60, academic grade, particle size 0.040–0.063 mm) or Aldrich neutral alumina was used for flash chromatography. NMR spectra were recorded on a DMX 500 instrument and calibrated using residual undeuterated solvent as an internal reference.
General Procedure for N-Boc Deprotection. To a solution of the N-Boc piperidine derivative starting material in CH2Cl2 (10 mL/mmol) was added water (2.5 mL/mmol) at 0 °C and the resultant biphasic mixture was stirred vigorously for 5 min before TFA (10 mL/mmol) was added dropwise. The resultant mixture was stirred vigorously at 0 °C for 1 h and then was diluted with CH2Cl2. The reaction contents were then poured into a separatory funnel containing an ice-cold 1 M NaOH / 0.5 M K2CO3 solution and shaken vigorously for 20 sec. The resultant layers were separated and the aqueous layer was extracted twice with CH2Cl2. The combined organic layers were then dried (Na2SO4), filtered, and concentrated. This material was taken up in benzene at 23 °C for 1 h (to ensure complete cyclization) then concentrated to afford enamines as light yellow to orange oils. All spectroscopic data for these materials matched those previously reported.5,16 [Note: vigorous shaking of the separatory funnel is necessary to ensure complete deprotonation of the amine. When not shaken vigorously enough, the resultant products exhibit reduced E-/Z- ratios. The use of saturated aqueous NaHCO3 in this operation led to poor mass recoveries, even following multiple extractions. These compounds were found to be unstable to isolation on neutral or basic alumina or Et3N-deactivated silica gel.]
General Procedure for the Knorr Pyrrole Cyclization. Freshly prepared enamine was dissolved in degassed MeOH (0.1 M, sparged with Ar for at least 20 min) at 23 °C, and after standing for 1 h, the solvent was removed to afford the crude pyrrole. Pure material was obtained by purification on a short plug of neutral alumina (Et2O/pentane, 1:9). All spectroscopic data for this material matched those previously reported.5,16
General Kinetic Procedures and Analysis. Kinetic measurements were made using 1H NMR spectroscopy on a Bruker DMX 500 MHz spectrometer at 35 °C. DMSO-d6/D2O solutions of known concentration were prepared using a Hamilton microliter syringe and a 2 mL volumetric flask. To obtain kobs for the cyclization, freshly prepared dienamine 15 or 16 was taken up in a solution of isopropanol-d8 or DMSO-d6/D2O and immediately transferred to an NMR tube. Following temperature equilibration to 35 °C, the instrument was locked, tuned, and shimmed. A 1H NMR spectrum was then obtained every 3 or 6 minutes using the kineticzg program, and the disappearance of starting material was measured by monitoring the integration of the peak corresponding to the dienamine β-CH relative to residual benzene as internal standard. A plot of ln [SM] vs. time (s) allowed a fit of a line with slope kobs. To obtain kH/kD, this procedure was repeated with both 15 and 16 using isopropanol-d8 or the same solution of DMSO-d6/D2O. To calculate the order of water in the cyclization, the above procedure was repeated at various concentrations of D2O in DMSO-d6. A plot of kobs vs. [D2O] then showed an excellent fit to an exponential curve (y=axb), whereas a plot of kobs/[D2O] vs. [D2O] showed a linear relationship.
General Procedure for One-Pot Deprotection-Knorr Pyrrole Synthesis. To a solution of the alkene starting material 14 (7.0 mg, 0.02 mmol) in CH2Cl2 (0.2 mL) was added water (0.05 mL) at 0 °C and the resultant biphasic mixture was stirred vigorously for 5 min before TFA (0.2 mL) was added dropwise. The resultant mixture was stirred vigorously at 0 °C for 1 h. The deprotection was then was quenched by addition of saturated aqueous NaHCO3 (2.5 mL) and MeOH (5.0 mL), and the reaction was stirred at 23 °C for 2 h. The reaction contents were then poured into a separatory funnel containing 1 M NaOH (10 mL) and extracted with CH2Cl2 (2 × 10 mL). The combined organic layers were then dried (Na2SO4), filtered, and concentrated to afford a crude orange solid. This crude material was purified on a short plug of neutral alumina (Et2O/pentane, 1:9) to afford myrmicarin 215B (10) as a white crystalline solid (3.0 mg, 71% yield). All spectroscopic data for this synthetic material matched those previously reported.5
References
1. V. F. Ferreira, M. C. B. V. de Souza, A. C. Cunha, L. O. R. Pereira, and M. L. G. Ferreira, Org. Prep. Proc. Int., 2001, 33, 411; CrossRef K. I. Shinohara, T. Bando, and H. Sugiyama, Anti-Cancer Drugs, 2010, 21, 228; CrossRef S. Thirumalairajan, B. M. Pearce, and A. Thompson, Chem. Commun., 2010, 46, 1797. CrossRef
2. For synthetic work towards the molecules in Figure 1, see: T. Yamaguchi, T. Fukuda, F. Ishibashi, and M. Iwao, Tetrahedron, 2006, 62, 594; CrossRef P. S. Baran, A. L. Zografos, and D. P. O’Malley, J. Am. Chem. Soc., 2004, 126, 3726; CrossRef B. D. Roth, Prog. Med. Chem., 2002, 40, 1. CrossRef
3. S. Umio, K. Kariyone, K. Tanaka, and H. Nakamura, Chem. Pharm. Bull., 1969, 17, 559; A. C. Goudie, H. E. Rosenberg, and R. W. Ward, J. Hereocycl. Chem., 1983, 20, 1027; J. M. Hamby and J. C. Hodges, Heterocycles, 1993, 35, 843; CrossRef R. K. Bellingham, J. S. Carey, N. Hussain, D. O. Morgan, P. Oxley, and L. Powling, Org. Proc. Res. Dev., 2004, 8, 279; CrossRef J. M. Manley, M. J. Kalman, B. G. Conway, C. C. Ball, J. L. Havens, and R. Vaidyanathan, J. Org. Chem., 2003, 68, 6447; CrossRef For review articles on the synthesis of pyrroles, see: C. Schmuck and D. Rupprecht, Synthesis, 2007, 20, 3095; CrossRef J. C. Morris and A. J. Phillips, Nat. Prod. Rep., 2008, 25, 95; CrossRef V. Estévez, M. Villacampa, and J. C. Menéndez, Chem. Soc. Rev., 2010, 39, 4402. CrossRef
4. L. Knorr, Ber., 1884, 17, 1635; L. Knorr, Liebigs Ann. Chem., 1886, 236, 290. CrossRef
5. S. A. Snyder, A. M. ElSohly, and F. Kontes, Angew. Chem. Int. Ed., 2010, 49, 9693. CrossRef
6. F. Schröder and W. Francke, Tetrahedron, 1998, 54, 5259; CrossRef For the isolation of the myrmicarins, see: W. Francke, F. Schröder, F. Walter, V. Sinnwell, H. Baumann, and M. Kaib, Liebigs Ann., 1995, 965; CrossRef F. Schröder, S. Franke, W. Francke, H. Baumann, M. Kaib, J. M. Pasteels, and D. Daloze, Tetrahedron, 1996, 52, 13539; CrossRef F. Schröder, V. Sinnwell, H. Baumann, and M. Kaib, Chem. Commun., 1996, 2139; CrossRef F. Schröder, V. Sinnwell, H. Baumann, M. Kaib, and W. Francke, Angew. Chem., 1997, 109, 161 and Angew. Chem., Int. Ed. Engl., 1997, 36, 77. CrossRef
7. E. Fabiano and B. T. Golding, J. Chem. Soc., Perkin Trans. 1, 1991, 3371. CrossRef
8. S. K. Khetan and A. George, Tetrahedron, 1969, 25, 527; CrossRef G. Sánchez and A. Cert Ventulá, Carbohyd. Res., 1971, 17, 275; CrossRef L. Calvo, A. Gonzáles-Ortega, and C. Sañudo, Synthesis, 2002, 16, 2450. CrossRef
9. J. B. Paine III, J. R. Brough, K. K. Buller, and E. E. Erikson, J. Org. Chem., 1987, 52, 3986; CrossRef V. A. Yaylayan and A. Keyhani, Food Chemistry, 2001, 74, 1. CrossRef
10. G. Capozzi, G. Modena, and L. Ronzini, J. Chem. Soc., Perkin Trans. 1, 1972, 1136; CrossRef A. G. Schultz and W. K. Hagmann, J. Chem. Soc., Chem. Commun., 1976, 726; CrossRef N. Vivona, S. Buscemi, V. Frenna, and G. Cusmano, Adv. Heterocycl. Chem., 1993, 56, 49; CrossRef A. P. Piccionello, A. Pace, S. Buscemi, and N. Vivona, Org. Lett., 2009, 11, 4018; CrossRef E. E. Maciver, S. Thompson, and M. D. Smith, Angew. Chem. Int. Ed., 2009, 48, 9979; CrossRef X. Wang, B. Han, J. Wang, and W. Yu, Org. Biomol. Chem., 2010, 8, 386.
11. 16 was prepared according to the procedure in ref. 5, using methanol-d4 in the epimerization of C-5.
12. J. M. Jones and M. L. Bender, J. Am. Chem. Soc., 1960, 82, 6322; CrossRef P. Geneste, G. Lamaty, and J. P. Roque, Tetrahedron, 1971, 27, 5539; CrossRef S. A. Adediran, S. A. Deraniyagala, Y. Xu, and R. F. Pratt, Biochemistry, 1996, 35, 3604. CrossRef
13. J. Toullec and J. E. Dubois, J. Am. Chem. Soc., 1974, 96, 3524. CrossRef
14. G. S. Hammond, C. E. Reeder, F. T. Fang, and J. K. Kochi, J. Am. Chem. Soc., 1958, 80, 568; CrossRef E. S. Hand and W. P. Jencks, J. Am. Chem. Soc., 1975, 97, 6221; CrossRef J. W. Henderson and P. Haake, J. Org. Chem., 1977, 42, 3989; CrossRef G. Gopalakrishnan and J. L. Hogg, J. Org. Chem., 1984, 49, 3161; CrossRef J. A. Byers and T. F. Jamison, J. Am. Chem. Soc., 2009, 131, 6383. CrossRef
15. C. A. C. Haley and P. Maitland, J. Chem. Soc., 1951, 3155. CrossRef
16. B. Sayah, N. Pelloux-Léon, and Y. Vallée, J. Org. Chem., 2000, 65, 2824; CrossRef M. Santarem, C. Vanucci-Bacqué, and G. Lhommet, Heterocycles, 2010, 81, 2523. CrossRef