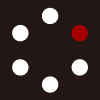
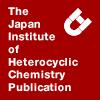
HETEROCYCLES
An International Journal for Reviews and Communications in Heterocyclic ChemistryWeb Edition ISSN: 1881-0942
Published online by The Japan Institute of Heterocyclic Chemistry
e-Journal
Full Text HTML
Received, 22nd June, 2011, Accepted, 4th August, 2011, Published online, 11th August, 2011.
DOI: 10.3987/REV-11-SR(P)4
■ Truncated Aspidosperma Alkaloid-Like Scaffolds: Unique Structures for the Discovery of New, Bioactive Compounds
Scott C. Benson, Lily Lee, Wanguo Wei, Feng Ni, Julian David Janna Olmos, Kyle R. Strom, Aaron B. Beeler, Ken Chih-Chien Cheng, James Inglese, Smitha Kota, Virginia Takahashi, A. Donny Strosberg, John H. Connor, G. Guy Bushkin, and John K. Snyder*
Department of Chemistry, Boston University, 590 Commonwealth Ave RM 474, Boston, MA 02215, U.S.A.
Abstract
Exploration into the dienophilicity of indole in inverse electron demand Diels-Alder reactions has led to the development of the triazatetracyclo[7.7.0.01,13.02,7]hexadeca-2,4,6,10,12-pentaene and related scaffolds for diversification in the search for new, biologically active compounds. The libraries constructed from these core structures have lead to the discovery of new anti-viral and anti-malarial compounds.INTRODUCTION
We have long been interested in the inverse electron demand Diels-Alder (IEDDA) chemistry of heteroaromatic compounds with latent enamine functionalities serving as dienophiles. Within this general theme, we have focused primarily on the dienophilicity of indole,1 and to a lesser extent pyrrole2 and imidazole,3 in reactions with electron deficient heteroaromatic azadienes, and applied this chemistry to the synthesis of new heterocycles. Indeed, these are areas of research which Professor Al Padwa has greatly influenced as a prolific leader with his ground-breaking work. Most notable in this effort is his work with the cycloadditions of indole with electron deficient dienes and dipoles applied to the synthesis of a large number of alkaloids and alkaloidal analogues.4
Quite some time ago we began an investigation into the use of a sequence of inverse electron demand Diels-Alder reactions between 1,2,4,5-tetrazines (1, X = N) tethered to tryptamine or tryptophan as a route to access the Aspidosperma alkaloidal skeleton 4 (Scheme 1).1b,5 Simultaneously, an analogous approach with tethered 1,2,4-triazines (1, X = CR’) was also developed. This work began with an examination into the dienophilicity of the indole subunits of tryptamine and tryptophan with tethered tetrazines and triazines in intramolecular IEDDA reactions, which proved to be very successful, exemplified by the reactions in Scheme 2. Linking the heterocycle to the tryptamine or tryptophan amino group was easily accomplished by simple SNAr chemistry with displacement of an appropriate leaving group (OMe, SMe, or Cl).1b In the course of this work, it was discovered that in order for the reaction to proceed, the tethering nitrogen linking the tetrazine to the tryptamine must be acylated to reduce electron donation from the amine lone pair into the heteroaromatic diene. The extent of this electron donation in non-acylated tethered triazines was readily apparent from the 1H NMR spectra of the tethered 1,2,4-triazines which displayed slowly interconverting rotamers reminiscent of rotamers frequently observed with amides (Figure 1). Indeed, the ~0.8 ppm downfield shift of the H-9 methylene protons of tryptamine upon tethering of the triazines was reminiscent of acylation shifts upon amide formation.6 Furthermore, we speculated that in the case of tethered triazines, the IEDDA reaction required a second acylation of a triazine nitrogen since N-acetyl tethered tryptamine/triazine pair 5 did not undergo a cycloaddition upon heating to 232 oC, though the reaction proceeded smoothly in refluxing Ac2O (bp 140 oC, Scheme 2, Eq 1). N-Acylated tethered tetrazines such as 7 did participate in the thermally promoted cycloadditions in the absence of in situ acylation (Scheme 2, Eq 2).
The intramolecular cycloadditions of various tethered tetrazines and triazines could be accomplished by refluxing in acetic anhydride or in o-dichlorobenzene in the presence of trifluoroacetic anhydride (TFAA). With tryptophan derivatives, only single diastereomers were obtained, indicating that the stereogenic center of this amino acid is able to control the facial approach of the electron-rich indole to the electron deficient heteroaromatic azadiene (Scheme 3).1b
Acylation of the tethering nitrogen presumably occurs prior to the cycloaddition. In acetic anhydride, the cycloadducts were isolated as the N-14 acetyl derivatives (Table 1). With TFAA, the trifluoroacetate group was cleaved during chromatography on SiO2, thereby leaving this position poised for diversification. All of the tetrazines tethered to either tryptamine or tryptophan participated in IEDDA reactions under acetylation conditions, producing the desired cycloadducts in good to excellent yields. With electron-withdrawing substituents at C-6, the cycloadditions of tethered tetrazines could be accomplished under relatively mild conditions, proceeding at temperatures as low as rt to 40 oC (Table 1, Items 3 and 4) Less electron deficient tetrazines required heating to 180 oC (Table 1, Items 1 and 2). Chlorotetrazine 17 also underwent a cycloaddition upon heating to 40 oC, but the cycloadduct proved to be unstable. Nonetheless, 17 proved to be an excellent precursor to tetrazinylnitrile 18, which also participated in the cycloaddition, producing 24 (Table 1, Item 3). In general, the tethered triazines required two electron withdrawing groups at the triazinyl C-5 and C-6 positions, (Table 1, Items 5 and 6) as well as the higher temperatures (179 - 180 oC) in order to accomplish the cycloaddition.
Interestingly, the C-rings of tetrazine cycloadducts 8, 22, 23, and 24 were in the 1,2-dihydro tautomeric states, as evidenced by the C-9 methine singlet in the δ 3.80 – 4.35 range, depending on the C-10 substituent, with the corresponding carbon at δ 67.0 – 70.1. In cycloadduct 25 the vinylogous carbamate form of the C-ring predominated exclusively (Figure 2).
This tautomer was initially suggested by the low field singlet at δ 11.48 (NH) in the 1H NMR spectrum which did not correlate with a carbon in the HSQC spectrum, and which very slowly exchanged with deuterium in D2O-saturated CDCl3 (90% exchanged after 3 days), indicating a strong intramolecular hydrogen bond.7 This hydrogen bond is likely the cause of the predominance of the vinylogous carbamate form. Furthermore, a non-hydrogen bearing enamine-type β-carbon appeared at δ 92.7. In addition, a methine singlet was observed at δ 4.32 with the corresponding carbon at δ 67.9 assigned to H-9. An NOE between this ring fusion methine proton and H-16b not only supported the cis B,C-ring fusion, but also ruled out the 1,4-dihydropyridazine tautomer 25’. As with other cycloadducts, NOE’s from H3 to both H15 and H16a allowed assignment of the facial selectivity in the cycloaddition, and hence the absolute stereochemistry given the enantiopurity of the starting tryptophan.
As noted above, the triazines were much less reactive than the tetrazines. Only those tethered triazines with two electron-withdrawing groups at the C-5 and C-6 positions participated in the cycloadditions. Those triazines which failed to undergo the intramolecular Diels-Alder reactions are shown in Figure 3. Emphasizing the importance of two electron withdrawing substituents was the failure of triazine 31, with a single ester substituent, to react with either tryptophan or tryptamine. Triazine 14 (Table 1, Item 6) represents a previously unreported heterocycle, which was surprisingly made with complete regioselectivity by the condensation of dione 32 with S-methylisothiosemicarbazide (Scheme 4). The regioselectivity of this cyclocondensation was confirmed by 13C-19F couplings observed in the 13C-NMR spectrum of the cycloadduct 27 (Inset), notably 2J = 29.8 Hz to C-10 (δ 109.7) and 3J = 3.7 Hz to C-11 (δ 140.5). In addition, 5J = 2.3 Hz to the N-methyl carbon (δ 37.7) was also seen, with the Fermi contact presumably propagated by fluorine interaction with spatially close N-methyl protons.8
Unfortunately, we were never able to affect a second cycloaddition in the designed sequence to 4 (cf. Scheme 1). Triazine cycloadduct 33, produced by the cycloaddition in TFAA followed by acylation with pentynoyl anhydride,1b underwent a second cycloaddition as hoped, but the cycloreversion produced the pyridine subunit 34 rather than the desired pentacyclic core 35, which would have formed by the release of methyl cyanformate (Scheme 5). The analogous pentynoylated tethered cycloadduct 36, failed to participate in further cycloadditions. The alkyne in tethered tetrazine 1a, proved to be more dienophilic than the indole subunit, producing pyridazine 37 (x = 0) in near quantitative yield. Neither this thiomethyl pyridazine cycloadduct, nor the sulfoxide 38 (x = 1), produced by m-CPBA oxidation of 37, underwent a cycloaddition under the many conditions investigated.
Ultimately, Dale Boger cleverly showed, by constructing a 1,3,4-oxadiazole heteroaromatic diene system onto tryptamine, that the desired Aspidosperma scaffolds could be obtained following the tandem cycloaddition strategy.9 Boger’s group has subsequently applied this chemistry to the synthesis of a number of Aspidosperma and Vinca alkaloids, including minovine,9 fendleridine,10 vindorosine,11 vindoline12 and their analogues.13
Our efforts in the cycloaddition chemistry of tryptamine and tryptophan went into hibernation after the publication in 2000.1b However, the rise of diversity oriented synthesis (DOS)14 as a means to build upon complex, non-natural scaffolds led us to consider the development of the 8,12,14-triazatetracyclo[7.7.0.01,13.02,7]hexadeca-2,4,6,10,12-pentaene (39) and 8,11,12,14-tetraazatetracyclo[7.7.0.01,13.02,7]hexadeca-2,4,6,10,12-pentaene (40) cycloadduct cores (Figure 4) as scaffolds for library synthesis. The rapid assembly of complex molecular libraries in DOS has become an important approach to discovering novel chemotypes as new pharmacological tools. Libraries with scaffolds that structurally mimic natural products can provide to new leads with unique pharmacological properties.15 Several comparatively simple indole-based libraries have been reported,16 as have more complex unnatural indole alkaloid-type libraries,17 with subsequent screening revealing bioactivities worthy of further biological investigation.18 Scaffolds 39 and 40 resemble truncated Aspidosperma alkaloids with transposed nitrogens in the C-ring, and represent ideal candidates for library synthesis and subsequent screening. These scaffolds are of relatively moderate molecular weight (350-400), with several distinct sites for elaboration (R1 through R6). With this in mind, the synthesis of a series of libraries based on these core structures was completed within the Center for Chemical Methodology and Library Development at Boston University (CMLD-BU),19 an NIH funded center for reaction and novel chemotype discovery. What has emerged from this work is the recognition that these tetracyclic scaffolds exhibit biological activity against various infectious diseases differentiated by the mode of diversification.
DIVERSIFICATION OF TETRAZINE AND TRIAZINE CYCLOADDUCTS OF TRYPTOPHAN
Figure 5. Triazine- and tetrazine-derived scaffolds for diversificationThe first generation libraries from the two scaffolds 39 and 40 employed (L)-tryptophan as the starting dienophile, resulting in diastereomerically and enantiomerically pure cycloadducts. Furthermore, (D)-tryptophan is commercially available, so the preparation of enantiomers would be straightforward. Following the strategy outlined in Scheme 3, and employing the triazine 13 and tetrazine 9 as azadienes (cf. Table 1) with TFAA as the acylating reagent to promote the cycloaddition, six triazine and six tetrazine cycloadducts, 41a-f and 42a-f, were prepared from the corresponding tryptophan dienophiles20 (Figure 5). Deacylation of the trifluoroacetate group occurred during flash chromatography on silica gel, rendering the N-14 position available for diversification. These twelve scaffolds were prepared on 2 g scales.
The scaffolds were subsequently diversified through acylations and sulfonylations with seventeen commercially available acid chlorides and ten sulfonyl chlorides.20 As illustrated for the triazine-derived cycloadducts (Scheme 6), the acylations used PS-DMAP21 as a base promoter, while the sulfonylations utilized Et3N, with subsequent treatment with PS-trisamine22 to scavenge excess sulfonyl chloride. No competing acylation or sulfonylation of the unprotected indoline nitrogen was observed in the diversification of scaffolds 41a and 42a (R1 = H). Final purification was achieved through mass directed LC-MS.
Representative members of the library prepared are shown in Figure 6. The formation of ureas 49 from scaffolds 41 was also investigated (Scheme 7). While the ureas could be isolated in good yields, they proved unstable to storage, undergoing a deacylation, regenerating the original scaffold 41 and the isocyanate. This instability is perhaps not surprising given the potential of the dihydropyridine ring N-12 to function as an intramolecular base catalyst for urea cleavage.23 Base treatment of 49 also resulted in complete deacylation with isocyanate release and recovery of 41. Consequently, these ureas were not further considered for inclusion in a library.
The library members from both scaffolds 41 and 42 were stable upon storage on the bench-top in the solid state. While the triazine adducts were also stable in a variety of solvents including DMSO, the tetrazine analogues slowly formed adducts with DMSO upon storage in that solvent, as seen by UPLC. The DMSO adduct presumably forms at C-13 position of the tetrazine cycloadducts, a position sensitive to addition of other nucleophiles such as primary amines.24 No such adducts were observed with the triazine cycloadducts, wherein the C-13 is considerably less electrophilic. Given the reactivity of tetrazine-derived library members from scaffolds 42 in DMSO, these compounds were not submitted for biological evaluation.
The 132-membered library prepared from the triazine cycloadducts were screened for biological activity in a variety of assays as part of the CMLD-BU small molecule collection. Three different assays showed “Hits” from this unnatural alkaloid library, as defined by the collaborators performing the assay (Figure 7). These assays were: (1) inhibition of the dimerization of the capsid protein of the hepatitis C virus (HCV), known as “core”;25 (2) proliferation inhibition of several strains of the malaria-inducing Plasmodium falciparum;26 and (3) inhibition of replication of the vesicular stomatitis virus (VSV), a negative strand RNA virus.27 All hits were validated by resubmission of fresh samples to the assay. The active compounds were specific to the particular assay; thus, there was no cross-over of hits from one assay to the other at the tested concentrations.
THE BIOASSAYS AND THE HITS
Hepatitis C, a major cause of liver disease, infects over 130 million people worldwide. The causative agent is a positive sense, single strand RNA virus, which was first reported in 1989.28 Core is one of ten proteins encoded by the HCV RNA genome, and is required for viral assembly.29 Inhibition of core dimerization has been demonstrated to prevent HCV proliferation in infected liver cells,25,30 and as such core represents an attractive non-enzymatic target for the development of potential HCV treatment. Our collaborator in this work was Professor Donny Strosberg and co-workers of the Scripps Research Institute who have developed the core dimerization inhibition assay.25 Compound 50 from the library showed activity (IC50 5.7 – 9.3 µM) in this assay (Figure 7A). Given the single hit with an IC50 < 10 µM, it was difficult to develop an SAR assessment, other than the fact that only N-14 acylated analogues showed any activity at all, N-sulfonylated analogues within this library were invariably inactive.
Malaria is a pernicious disease that has ravaged human civilization, and continues to devastate underdeveloped nations where the impact can be difficult to fully define.31 Indeed, this disease is, at least in part, responsible for many regions of the world continued underdeveloped state as it imposes an enormous societal burden on populations economically ill-equipped to deal with its consequences.32 Strains of the malaria-inducing protozoan Plasmodium falciparum resistant to the traditional treatment with Cinchona alkaloids and their derivatives have emerged. James Inglese and his colleagues at the NIH’s Chemical Genomics Center and NIAID have established a quantitative high-throughput screening (qHTS) platform examining five strains of P. falciparum in an effort to discover new leads against malaria, and also identify differential activities among the strains, thereby enabling determination of the genetic locus mediating the activity when patterns of inheritance are mapped from recombinant progeny. Three compounds from this library (51 – 53) showed activity (EC50) against several strains of P. falciparum in the single digit µM range or below (Figure 7B).33
Preliminary SAR analysis suggested that, in contrast to the anti-HCV compounds, only N-14 sulfonylated library members were active against the malarial strains. Moreover, only analogues derived from the N-8 benzyl scaffold showed activity at the sub-micromolar level. Following this observation, 40 additional analogues were synthesized using the inverse electron demand Diels-Alder strategy, and screened for anti-malarial activity, varying the N-8 benzyl substituents and the N-14 sulfonamide groups.33 Those compounds with o-substituted benzyl substituents at N-8 were found to be the most active, with the most effective substituent being the trifluoromethyl group. Two new cycloadducts (59 and 60) were discovered form this focused library with single digit micromolar or sub-micromolar activity against five strains of P. falciparum (IC50 0.6 – 0.7 µM for 59, 0.6 – 0.8 M for 60, depending on the strain).33
Different library members were also identified as hits in a screen for antiviral compounds that target vesicular stomatitis virus (VSV). Vesicular stomatitis virus is a veterinary virus used to determine compounds with potential activity against human pathogenic viruses. The screen itself was a cell-based assay for the inhibition of viral replication, which was developed by Professor John Connor of the Boston University School of Medicine, who has previously used VSV to identify broad spectrum antiviral agents.27,34 The assay design was a “dual screen” that simultaneously identified compounds that (1) inhibited the replication of virus, and (2) were not cytotoxic to cells.35 In this assay, compounds that protected cells from virus-mediated apoptosis and did not cause cell death were deemed active. Five compounds, 54 – 58, from the library were found to be active with EC50’s in the 12.5 – 50 µM range (Figure 7C). As with the HCV activity, all of these compounds were derived from N-14 acylation. Furthermore, none of these anti-VSV hits were active against HCV core dimerization.
SECOND GENERATION LIBRARIES
With these hits confirmed, two additional libraries were prepared, focusing on the HCV and anti-malarial activities. One library expanded the D-ring with the formation of a δ–lactam ring (61), initially focusing on two points of diversion R1 and R2. The second library 62 was prepared by converting the two triazine-derived ester groups of 47 and 48 into a cyclic imide.
Construction of the δ-lactam library 61 began with the preparation of N-alkylated-3-indolylpropionic acid derivatives 63 (Scheme 8).20 N-Methylation of indolylpropionic acid to 63a proceeded through N,O-dimethylation with excess methyl iodide under basic conditions, followed by basic hydrolysis of the methyl ester. Other N-alkyl derivatives 63b-e were prepared from the methyl ester of indolylpropionic acid by N-alkylation with the appropriate alkyl halides following KH deprotonation, then basic ester hydrolysis.
The cycloaddition precursors, the N-substituted indole-3-propionic acid derived amides 67, were then prepared by acylation of various 3-aminotriazines 66 with the propionic acid derivatives. To this end, the 3-chloro-1,2,4-triazine 65, prepared from thiomethyl triazine 13 upon treatment with chlorine, was reacted with various primary amines in the presence of di-iso-propylethylamine (DIPEA) to produce the corresponding aminotriazines 66 in near quantitative yields by SNAr displacements. Linking the diene/dienophile pairs was accomplished by reacting 66 with N-substituted indole-3-propionic acid chlorides 64a-e using PS-DMAP as the base promoter. Filtration through a short silica gel column removed unreacted acid chloride to give the amides 67 bearing the tethered dienophile/diene pairs in nearly quantitative yields. Heating these amides at 120 oC in o-dichlorobenzene for 2h resulted in the inverse electron demand intramolecular Diels-Alder reactions to give the desired cycloadducts 61, also in near quantitative yields. The products were purified by mass-directed preparative LC-MS. Eighty-two library members, all in racemic state, were synthesized in straightforward fashion using these protocols.
Screening of this new δ-lactam library in the core dimerization inhibition assay to identify new anti-HCV candidates revealed several additional hits with single digit µM activity (IC50’s, Chart 1). Three of these new active compounds (61a–c) were validated by resynthesis, and their biological activities were further probed. All three were approximately twice as potent as the original lead compound 50. Furthermore, 61a and 61c showed no cytotoxicity against Huh7-5 hepatoma cells at concentrations up to 125 µM, and also significantly inhibited HCV viral propagation in infected hepatoma cells with EC50 < 1 µM.19 None of the members of this δ-lactam library were active against malaria; these compounds were not tested against VSV.
With the improved anti-HCV activity of the δ-lactam hits, additional analogues were prepared, focusing on the tethering of a second heterocycle to the N-8 position. The rationale for designing new candidates with a second heterocyclic ring tethered to the initial hit(s) was the hope that this entity would find a second “hot spot” on the surface of core, resulting in stronger binding. Such an approach is not uncommon when seeking to inhibit a protein-protein interaction.36 As recently reported,37 two of these new compounds, 68 and 69, showed significantly improved IC50’s against core dimerization (68, 98 nM, 69 341 nM), with cytotoxicity levels > 100 µM. Work is continuing to build an additional library of tethered triazoles around these results.
The library of cyclic imides 62 was prepared utilizing the six cycloadduct scaffolds 70a-f shown in Chart 2. These scaffolds were prepared beginning with the N-BOC L-tryptophan methyl ester (43a, Scheme 9). Alkylation of the indole nitrogen following standard procedures produced 43b-c (Scheme 9, RX = CH3I, BnBr). Reduction of the methyl ester to the primary alcohol, followed by removal of the BOC group, then tethering of the triazine 13 through base promoted SNAr displacement gave the tethered triazines 71a-b. Protection of the primary alcohol as the TBS ether, then cycloaddition by refluxing in dioxane in the presence of trifluoroacetic anhydride (TFAA), yielded 72a-b.
Completion of the cyclic imide scaffolds was accomplished by basic hydrolysis of both esters, then imide formation with primary amines (Scheme 10).
Diversification of scaffold 70a by acylation and sulfonylation using ten acyl chlorides and nine sulfonyl chlorides, then desilylation with TBAF gave the first subset of 85 cyclic imide library members 62. Sixty of these members were further acetylated at the Chart 3. Examples of the Cyclic Imide Sublibraryprimary alcohol as well (73), to produce a total number of 151 cyclic imides, which included the scaffolds 70a - 70f. Library members were purified by mass-directed LC-MS; representative examples are shown in Chart 3. All members of this library are single enantiomers with the chirality determined by that of the starting tryptophan.
Screening of the cyclic imide library against HCV core dimerization did not reveal any members with single digit micromolar IC50 values, though several were identified with double digit micromolar activities. All members of the cyclic imide library were inactive against malaria. Preliminary screening of this library against VSV revealed two hits, 74 and 75; IC50 values have not as yet been determine for 74 and 75.
Recently, a third generation library was generated through cross-coupling chemistry employing members of the original cycloadduct library 41, as well as the δ-lactam library 61. Iodination of scaffold 41a as well as select library members from the δ-lactam library 61 with NIS in acetonitrile occurred at rt (30 min for 41a, Scheme 11, Eq 1 10 hr for 61, Scheme 11, Eq 2).38 In all cases, exclusive regioselectivity was observed, with iodination occurring solely at the position para to the original indole nitrogen. The iodinations were successful on a multigram scale.
With the aryl iodides 76 and 78 in hand, various Pd-catalyzed cross-couplings were examined (Table 2). Both Suzuki39 and Sonogashira40 couplings proceeded smoothly under standard conditions, thereby setting the protocols for an expanded library using eight iodinated scaffolds with ten terminal alkynes in Sonogashira cross-couplings (Chart 4). An eighty-membered library has been completed, with the compounds purified by mass-directed preparative HPLC. These compounds are awaiting screening results; representative members are shown in Table 2.
SUMMARY
The intramolecular inverse electron demand Diels-Alder reaction between the electron rich dienophilic double bond of trytamine, tryptophan and related indolic analogues, with tethered electron deficient heteroaromatic azadienes yields indoline alkaloid-type scaffolds which can be used in library synthesis. These natural product-like core structures have been diversified to yield library members with antiviral activities against hepatitis C virus as well as vesicular stomatitis virus. In addition, compounds active against the malaria inducing P. falciparum have also been discovered. The readily prepared cycloadduct scaffolds, 8,12,14-triazatetracyclo[7.7.0.1,13.02,7]hexadeca-2,4,6,10,12-pentaene (39), 8,11,12,14-tetraaza- tetracyclo[7.7.0.01,13.02,7]hexadeca-2,4,6,10,12-pentaene (40), and 5,7,11-triazatetracyclo- [8.7.0.01,6.012,17]heptadeca-6,8,12,14,16-pentaen-4-one (61) have thereby proven to be promising structures for library generation and hit discovery in the search for new biological probes and drug candidates.
ACKNOWLEDGEMENTS
We thank National Science Foundation (NSF CHE-9501069), the NIGMS CMLD initiative (P50 GM067041), and the Boston University Undergraduate Research Opportunities Program for financial support. We are also grateful to the NSF for the purchase of the NMR (CHE 0619339) and HRMS spectrometers (CHE 0443618) used in this work.
References
1. For a review of indole as a dienophile in inverse electron demand Diels-Alder reactions: (a) L. Lee and J. K. Snyder, ‘Advances in Cycloadditions’, Vol 6, ed. by M. Harmata, JAI Press: Stamford, CT, 1999, pp. 119-171; For publications from our group since this review: (b) S. C. Benson, L. Lee, L. Yang, and J. K. Snyder, Tetrahedron, 2000, 56, 1165; CrossRef (c) R. Nomak and J. K. Snyder, Tetrahedron Lett., 2001, 42, 7929. CrossRef
2. J.-H. Li and J. K. Snyder, J. Org. Chem., 1993, 58, 516. CrossRef
3. (a) Z.-K. Wan and J. K. Snyder, Tetrahedron Lett., 1997, 38, 7495; CrossRef (b) C. E. Neipp, P. B. Ranslow, Z. Wan, and J. K. Snyder, Tetrahedron Lett., 1997, 38, 7499; CrossRef (c) Z.-K. Wan, G. H. C. Woo, and J. K. Snyder, Tetrahedron, 2001, 57, 5497; CrossRef (d) B. R. Lahue, Z.-K. Wan, and J. K. Snyder, J. Org. Chem., 2003, 68, 4345; CrossRef (e) B. R. Lahue, S.-M. Lo, Z.-K. Wan, G. H. C. Woo, and J. K. Snyder, J. Org. Chem., 2004, 69, 7171. CrossRef
4. (a) A. Padwa, Y. Gareau; B. Harrison, and A. Rodriguez, J. Org. Chem., 1992, 57, 3540; CrossRef (b) A. Padwa, D. L. Hertzog, and W. R. Nadler, J. Org. Chem., 1994, 59, 7072; CrossRef (c) A. Padwa and A. T. Price, J. Org. Chem., 1995, 60, 6258; CrossRef (d) A. Padwa and M. A. Semones, Tetrahedron Lett., 1996, 37, 335; CrossRef (e) A. Padwa, S. R. Harring, and M. A. Semones, J. Org. Chem., 1998, 63, 44; CrossRef (f) A. Padwa and A. T. Price, J. Org. Chem., 1998, 63, 556; CrossRef (g) A. Padwa, M. A. Brodney, and M. Dimitroff, J. Org. Chem., 1998, 63, 5304; CrossRef (h) A. Padwa, M. A. Brodney, S. M. Lynch, P. Rashatasakhon, Q. Wang, and H. Zhang, J. Org. Chem., 2004, 69, 3735; CrossRef (i) J. M. Mejia-Oneto and A. Padwa, Org. Lett., 2004, 6, 3241; CrossRef (j) J. M. Mejia-Oneto and A. Padwa, Org. Lett., 2006, 8, 3275; CrossRef (k) X. Hong, S. France, J. M. Mejia-Oneto, and A. Padwa, Org. Lett., 2006, 8, 5141; CrossRef (l) X. Hong, J. M. Mejia-Oneto, S. France, and A. Padwa, Synlett, 2007, 775; CrossRef (m) X. Hong, S. France, and A. Padwa, Tetrahedron, 2007, 63, 5962; CrossRef (n) H. Zhang, J. Boonsombat, and A. Padwa, Org. Lett., 2007, 9, 279; CrossRef (o) J. Boonsombat, H. Zhang, M. J. Chughtai, J. Hartung, and A. Padwa, J. Org. Chem., 2008, 73, 3539; CrossRef (p) J. M. Mejia-Oneto, and A. Padwa, Helv. Chim. Acta, 2008, 91, 285. CrossRef
5. (a) S. C. Benson ’I. Indole as a Dienophile in Inverse Electron Demand Diels-Alder Reactions. II. The Synthesis of Optically Pure Sulfoxides.’ Ph.D. Dissertation, Boston University, 1991; (b) L. Lee ‘Inverse Electron Demand Diels-Alder Reactions of 3-Substituted Indoles: Studies Directed Toward the Synthesis of the Aspidosperma Alkaloidal Skeleta.’ Ph.D. Dissertation, Boston University, 1999.
6. E. Pretsch, T. Clerc, J. Seibl, and W. Simon, ‘Tables of Spectral Data for Structure Determination of Organic Compounds’ Springer-Verlag, New York, 1983, p. H5.
7. (a) P. H. von Dreele and I. A. Stenhouse, J. Am. Chem. Soc., 1974, 96, 7546; CrossRef (b) A. K. Wong, A. M. Finch, G. K. Pierens, D. J. Craik, S. M. Taylor, and D. P. Fairlie, J. Med. Chem., 1998, 41, 3417; CrossRef (c) P. Fita, N. Urbanska, C. Radzewicz, and J. Waluk, Z. Phys. Chem., 2008, 222, 1165. CrossRef
8. (a) R. H. Contreras, C. G. Giribet, M. A. Natiello, J. Perez, I. D. Rae, and J. A. Weigold, Aust. J. Chem., 1985, 38, 1779. Examples of five bind fluorine-carbon coupling; CrossRef (b) R. J. Spear, D. A. Forsyth, and G. A. Olah, J. Am. Chem. Soc., 1976, 98, 2493; CrossRef (c) J. L. Alderfer, R. E. Loomis, and T. J. Zielinski, Biochemistry, 1982, 21, 2738. CrossRef
9. (a) G. I. Elliott, J. R. Fuchs, B. S. J. Blagg, H. Ishikawa, H. Tao, Z.-Q. Yuan, and D. L. Boger, J. Am. Chem. Soc., 2006, 128, 10589; CrossRef (b) Z.-Q. Yuan, H. Ishikawa, and D. L. Boger, Org. Lett., 2005, 7, 741. CrossRef
10. E. Campbell, A. M. Zuhl, C. M. Liu, and D. L. Boger, J. Am. Chem. Soc., 2010, 132, 3009. CrossRef
11. G. I. Elliott, J. Velcicky, H. Ishikawa, Y. Li, and D. L. Boger, Angew. Chem. Int. Ed., 2006, 45, 620. CrossRef
12. (a) H. Ishikawa, G. I. Elliott, J. Velcicky, Y. Choi, and D. L. Boger, J. Am. Chem. Soc., 2006, 128, 10596; CrossRef (b) D. Kato, Y. Sasaki, and D. L. Boger, J. Am. Chem. Soc., 2010, 132, 3685; CrossRef (c) Y. Sasaki, D. Kato, and D. L. Boger, J. Am. Chem. Soc., 2010, 132, 13533. CrossRef
13. P. Va, E. L. Campbell, W. M. Robertson, and D. L. Boger, J. Am. Chem. Soc., 2010, 132, 8489. CrossRef
14. For some reviews on DOS: (a) S. L. Schreiber, Science, 2000, 287, 1964; CrossRef (b) D. S. Tan, Nature Chem. Biol., 2005, 1, 74; CrossRef (c) K. Itami and J.-i.Yoshida, Chem. Eur. J., 2006, 12, 3966; CrossRef (d) T. E. Nelson and S. L. Schreiber, Angew. Chem. Int. Ed., 2008, 47, 48; CrossRef (e) R. J. Spandl, A. Bender, and D. R. Spring, Org. Biomol. Chem., 2008, 6, 1149; CrossRef (f) R. A. Bauer, J. M. Wurst, and D. S. Tan, Curr. Opin. Chem. Bio., 2010, 14, 308. CrossRef
15. For reviews: (a) Y. Liao, Y. Hu, J. Wu, Q. Zhu, M. Donovan, R. Fathi, and Z. Yang, Curr. Med. Chem., 2003, 10, 2285; CrossRef (b) P. Arya, R. Joseph, Z. Gan, and B. Rakic, Chem. Biol., 2005, 12, 163; CrossRef (c) M. Kaiser, S. Wetzel, K. Kumar, and H. Waldmann, Cell. Mol, Life Sci., 2008, 65, 1186. CrossRef
16. (a) Y. Miyazaki and S. Kobayashi, J. Comb. Chem., 2008, 10, 355; CrossRef (b) S. A. Worlikar, B. Neuenswander, G. H. Lushington, and R. C. Larock, J. Comb. Chem., 2009, 11, 875. CrossRef
17. (a) C. W. Lindsley, D. D. Wisnoski, Y. Wang, W. H. Leister, and Z. Zhao, Tetrahedron Lett., 2003, 44, 4495; CrossRef (b) D. Fokas, L. Yu, and C. M. Baldino, Mol. Div., 2005, 9, 81; CrossRef (c) M. P. Castaldi, D. M. Troast, and J. A. Porco, Jr., Org. Lett., 2009, 11, 3362; CrossRef (d) Y. Zhang and J. S. Panek, Org. Lett., 2009, 11, 3366; CrossRef (e) K. Jadidi, R. Ghahremanzadeh, and A. Bazgir, J. Comb. Chem., 2009, 11, 341; CrossRef (f) V. Singh, S. Hutait, S. Biswas, and S. Batra, Eur. J. Org. Chem., 2010, 531; CrossRef For a review: (g) S. A. Patil, R. Patil, and D. D. Miller, Curr. Med. Chem., 2009, 16, 2531. CrossRef
18. (a) C. W. Lindsley, M. J. Bogusky, W. H. Laister, R. T. McClain, R. G. Robinson, S. F. Barnett, D. Defeo-Jones, C. W. Ross, III, and G. D. Hartman, Tetrahedron Lett., 2005, 46, 2779; CrossRef (b) S. Pasquini, C. Mugnaini, A. Brizzi, A. Ligresti, V. Di Marzo, C. Ghiron, and F. Corelli, J. Comb. Chem., 2009, 11, 795; CrossRef (c) M. J. Thompson, V. Borsenberger, J. C. Louth, K. E. Judd, and B. Chen, J. Med. Chem., 2009, 52, 7503. CrossRef
19. http://cmld.bu.edu.
20. W. Wei, C. Cai, S. Kota, V. Takahashi, F. Ni, A. D. Strosberg, and J. K. Snyder, Bioorg. Med. Chem., 2009, 19, 6926. CrossRef
21. Y. Shai, K. A. Jacobson, and A. Patchornik, J. Am. Chem Soc., 1985, 119, 4249. CrossRef
22. R. J. Both and J. C. Hodges, J. Am. Chem. Soc., 1997, 119, 4882. CrossRef
23. Analogously, N-nitrosoureas are known to decompose to form isocyanates: (a) J. A. Montgomery, R. James, G. S. McCaleb, and T. P. Johnston, J. Med. Chem., 1967, 10, 668; CrossRef Decomposition of labile ureas to form isocyanates has been reported often with arylurea herbicides and imidazole-containing ureas: (b) J. K. Tolson, H. A. Moye, and J. P. Toth, J. Agric. Food Chem., 1999, 47, 1217. CrossRef
24. W. G. Wan and J. K. Snyder, Unreported results.
25. S. Kota, L. Scampavia, T. Spicer, A. B. Beeler, V. Takahashi, J. K. Snyder, J. A. Porco, Jr., P. Hodder, and A. D. Strosberg, ASSAY Drug Dev. Tech., 2010, 8, 96. CrossRef
26. J. Yuan, R. L. Johnson, R. Huang, J. Wichterman, H. Jiang, K. Hayton, D. A. Fidock, T. E. Wellems, J. Inglese, C. P. Austin, and X.-z. Su, Nature: Chem. Bio., 2009, 5, 765. CrossRef
27. (a) J. H. Connor, M. O. McKenzie, G. D. Parks, and D. S. Lyles, Virology, 2007, 362, 109; CrossRef (b) E. F. Dunn, R. Fearns, and J. H. Connor, J. Virol., 2009, 83, 11665; CrossRef (c) D. R. Smith, S. McCarthy, A. Chrovian, G. Olinger, A. Stossel. T. W. Geisbert, L. E. Hensley, and J. H. Connor, Antiviral Res., 2010, 87, 187. CrossRef
28. (a) Q. L. Choo, G. Kuo, A. J. Weiner, L. R. Overby, D. W. Bradley, and M. Houghton, Science, 1989, 244, 359; CrossRef (b) G. Kuo, Q. L. Choo, H. J. Alter, G. L. Litnick, A. G. Redeker, R. H. Purcell, T. Miyamura, J. L. Dienstag, M. J. Alter, C. E. Stevens, G. E. Tegtmeier, F. Bonino, M. Colombo, W.-S. Lee, C. Kuo, K. Berger, J. R. Shuster, L. R. Overby, W. Bradley, and M. Houghton, Science, 1989, 244, 362; CrossRef (c) P. Simmonds, J. Gen. Virol., 2004, 85, 3173. CrossRef
29. (a) A. Shavinsky, S. Boulant, F. Penin, J. McLauchlan, and R. Bartenschlager, J. Biol. Chem., 2007, 282, 37158; CrossRef (b) F. Penin, J. Dubuisson, F. A. Rey, D. Moradpour, and J. M. Pawlotsky, Hepatology, 2004, 39, 5. CrossRef
30. A. D. Strosberg, S. Kota, V. Takahashi, J. K. Snyder, and G. Mousseau, Viruses, 2010, 2, 1734. CrossRef
31. R. J. Chima, C. A. Goodman, and A. Mills, Health Pol., 2003, 63, 17. CrossRef
32. (a) J. Sachs and P. Malaney, Nature, 2002, 415, 680; CrossRef (b) D. Gollin and C. Zimmermann, IZA DP No. 2997, 2007: http://ftp.iza.org/dp2997.pdf.
33. L. E. Brown, K. C.-C. Cheng, W.-G. Wei, P. Yuan, P. Dai, R. Trilles, F. Ni, J. Yuan, R. MacArthur, R. Guha, R. Johnson, X.-Z. Su, M. M. Dominguez, J. K. Snyder, A. B. Beeler, S. E. Schaus, J. Inglese, and J. A. Porco, Jr., Proc. Natl. Acad. Sci., 2011, 6775. CrossRef
34. R. W. Sidwell, J. H. Huffman, G. P. Khare, L. B. Allen, J. T. Witkowski, and R. K. Robins, Science, 1972, 177, 705. CrossRef
35. J. H. Connor, Manuscript in preparation.
36. (a) W. M. Cholody, L. Hernandez, L. Hassner, D. A. Scudiero, D. B. Djurickovic, and C. J. Michejda, J. Med. Chem., 1995, 38, 3043; CrossRef (b) C. Melchiorre, V. Andrisano, M. L. Bolognesi, R. Budriesi, A. Cavalli, V. Cavrini, M. Rosini, V. Tumiatti, and M. Recanatini, J. Med. Chem., 1998, 41, 4186; CrossRef (c) A. Cavalli, M. L. Bolognesi, S. Capsoni, V. Andrisano, M. Bartolini, E. Margotti, A. Cattaneo, M. Recanatini, and C. Melchiorre, Angew. Chem. Int. Ed., 2007, 46, 3689; CrossRef (d) M. K. Hadden and B. S. Blagg, Bioorg. Med. Chem. Lett., 2007, 17, 5063; CrossRef For a review: (e) M. K. Hadden and B. S. Blagg, Anticanc. Agt. Med. Chem., 2008, 8, 807.
37. F. Ni, S. Kota, V. Takahashi, A. D. Strosberg, and J. K. Snyder, Bioorg. Med. Chem. Lett., 2011, 21, 2198. CrossRef
38. M. Feketa, P. Kolonits, and L. Novak, Heterocycles, 2005, 65, 165. CrossRef
39. (a) N. Miyaura and A. Suzuki, Chem. Rev., 1995, 95, 2457; CrossRef (b) F. Bellina, A. Carpita, and R. Rossi, Synthesis, 2004, 2419. CrossRef
40. R. Chinchilla and C. Najera, Chem Rev., 2007, 107, 874. CrossRef