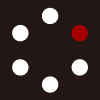
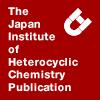
HETEROCYCLES
An International Journal for Reviews and Communications in Heterocyclic ChemistryWeb Edition ISSN: 1881-0942
Published online by The Japan Institute of Heterocyclic Chemistry
e-Journal
Full Text HTML
Received, 7th June, 2011, Accepted, 8th July, 2011, Published online, 19th July, 2011.
DOI: 10.3987/REV-11-SR(P)2
■ Absolute Configurational Elucidation of Monoterpene Indole Alkaloids by Circular Dichroism
Alfarius Eko Nugroho and Hiroshi Morita*
Faculty of Pharmaceutical Sciences, Hoshi University, 2-4-41 Ebara, Shinagawa-ku, Tokyo 142-8501, Japan
Abstract
Monoterpene indole alkaloids comprise a group of naturally occurring compounds, exhibiting a range of biological activities. These compounds have attracted great attention because of their interesting biosynthetic route and also as a challenging target for total synthesis. For such synthetic approach and structure – activity relationship studies, the knowledge about their absolute stereostructures is essential. This review summarizes the presently available results of studies on the determination of the absolute configuration of indole alkaloids by the use of circular dichroism (CD).INTRODUCTION
Monoterpene indole alkaloids are a group of naturally occurring compounds which exhibit a range of biological activities such as vasorelaxant,1 antiplasmodial,2-4 antileishmanial,5 antitumor,6 anti-HIV,7 and anti-melanogenic activities.8 The compounds of this group have also attracted great attention because of their interesting biosynthetic route and also as a target for total synthesis. Strychnine, for example, is considered to be one of the most complex natural products for its molecular size because it possesses 7 fused rings and six asymmetric center.9, 10 For the structure - activity relationship studies, biosynthetic elucidation and also for total synthesis of these monoterpene indole alkaloids, the knowledge or easy identification of their absolute configuration (AC) is essential. The AC of monoterpene indole alkaloids have often been determined by the X-ray crystallography, synthetic method, optical rotatory dispersion (ORD) or circular dichroism (CD) spectroscopy. Of these presently available methods, in this review, we will summarize the studies on the AC determination of monoterpene indole alkaloids through the use of CD spectroscopy. When reports on the use of CD for AC assignment are not available or is quite limited, but when reports on the use of ORD for the AC assignment are available, the use of ORD will also be discussed.
A. CORYNANTHEINES AND YOHIMBINES
For corynantheines and yohimbines, when the molecules contain indole moeity as the sole chromophore group, the use of CD [i.e. use of the sign of observed Cotton effect (CE) between 250 and 280 nm] is now an established method for the determination of C-3 AC. Klyne and coworker11 reported the first attempt to correlates the ORD and the AC heteroyohimbines but didn’t give a definite conclusion because among the heteroyohimbines they worked on were those having an α,β-unsaturated ester moiety. Subsequent reports by Klyne and coworker12 on the ORD of yohimbines and corynantheines, and one by Bartlett and coworker13 on the CD and ORD of yohimbines and its 17-keto derivatives established the correlation between the AC of C-3 and the sign of the CD CE in the 250 – 280 nm region. The basic skeleton of corynantheine, yohimbine and heteroyohimbine shown in Figure 1 should show a positive CE when the configuration of C-3 was S whereas a negative should be observed when it is R. In 1983, Seguin and coworker14 reported the CD analysis results of several heteroyohimbines with an α,β-unsaturated ester group (1–6) and their derivatives (7–19) which clearly demonstrated (see Table 1) the need to transform their α,β-unsaturated ester group into its corresponding non α,β-unsaturated ester derivatives to use the CE sign in the region of 250 – 280 nm for the determination of the AC.
B. SARPAGINE
When compared with the corynantheine skeleton, the sarpagine skeleton (Figure 2) possessing an additional ring which is formed by the linkage between C-5 and C-16, has an increased rigidity of the whole molecule, which is considered to affect the observed CD pattern of the compounds. Blaha and coworker15 investigated the effect of the additional ring on the observed CD and found that the additional ring only increased the rotatory strength and that it didn’t change the sign of the CE in the longer wavelength region (the Kauzmann-Eyring effect.16)
C. VOBASINE
There is no report on the relationship between the CD and the AC of vobasine type (Figure 2), whose skeleton is related to that of sarpagine but has no bonding between N-4 and C-3 and accordingly have different rigidity in the molecule. Blaha and coworker17 investigated the trans-annular interaction between C-3 and N-4 in the molecule by analyzing the CD data of several vobasine-type alkaloids of known AC. They found that there is no trans-annular interaction in the analyzed vobasine derivatives.
D. EBURNANE – VINCANE TYPE
As in the case of corynantheine and the yohimbine type compounds, the relationship between the AC of C-21 and the sign of the CE in the region of 250 – 310 nm has been established for eburnane - vincane type compounds. An early report on the relationship between ORD and the AC of the eburnane series by Blaha and coworker18 noted that the CEs in the longer wavelength region (>250 nm) are related to the AC of C-21, as in the case of yohimbine type compounds (C-3), whereas the CEs at the shorter wavelength region (<250 nm) are affected by the configuration of both C-16 and C-21. The relationship between CD and the AC of C-21 was further confirmed by Snatzke and coworker,19 according to which the CE was positive when C-21 was S and it was negative CE when C-21 was R. Snatzke and coworker19 also noted a strong effect of the C-16 substituents on the CE at about 230 nm: it is positive when the substituent was α and is negative when the substituent was β. One of the latest additions to the CD of eburnane - vincane alkaloids is by Caccamesse and coworker20 who reported the CD of all 8 isomers of vincamine (20–27) in ethanol (Figure 4).
E. IBOGA
Blaha and coworker21, 22 and also Klyne and coworker23 reported the existence of two enantiomeric series of iboga-type alkaloids. The former showed in 1974,21 that the CD of iboga-type alkaloids with a methoxycarbonyl group at C-16, (29) and those with no substituent at C-16 (28) are the same. Furthermore, they observed that though the sign of the first CE is affected by the substitution pattern of the indole ring, the signs of the second (around 280 nm), third (230 – 250 nm) and fourth (around 220 nm) CEs for one enantiomeric series are always negative, positive and negative, respectively, and for the other series a reversed CD profile. They related the observed pattern to the chirality of C-16, i.e. a negative 2nd CE is to be observed for the 16R enantiomer of 28 and the 16S enantiomer of 29.
F. INDOLINE AND INDOLENINE
In 1965, Klyne and coworker reported the correlation between ORD and the AC of some aspidospermine alkaloids.24 They noted that these compounds of 1st series (30 – 33) having 7S configuration showed a positive CE in the 240 – 290 nm region and those having 7R configuration, a negative CE. In the compounds of 2nd series (34 – 39), the CEs in the 270 – 340 nm region and at around 225 nm were shown to correspond to the configuration of C-7 : a positive CE in the 270 – 340 nm region and a negative CE at around 225 nm for the compounds with 7S configuration. Further studies by Klyne and coworker,25 extended the use of ORD for the AC assignment of N-acylindoline compounds (40 – 43). In these compounds (40 – 43), though the sign of the CE at a higher wavelength region (above 290 nm) is affected by the substitution on the indole ring, the signs of the CEs in the 230 – 270 nm region are not, and thus the CEs in the region of 230 – 270 nm may be usable for the AC assignment. In 40 – 43, a negative CE followed by a positive one were observed in the region of 230 – 270 nm when the compounds were of the 7R configuration and antipodal curves were observed when the compounds were of the 7S configuration. Subsequent report by Klyne and coworker26 further supports the previously proposed correlation between the CE signs in the 230 – 270 nm region and the AC of C-7.
G. QUEBRACHAMINE AND CLEAVAMINE
Studies on the ORD of some alkaloids structurally related to quebrachamine (44) related alkaloids by Klyne and coworker in 1967,12 show that when the quebrachamine derivatives have no substituent at C-16, the ORD can be related to the configuration of C-20. However, in C-16 substituted quebrachamines derivatives such as 45 the ORD (between 210 – 240 nm in particular) is affected by the configuration of both C-5 and C-16. Klyne and coworker assumed that the change of configuration at C-16 would cause a conformational change of the 9-membered ring resulting in the changes of the profiles of the observed ORD.
Recent use of CD for the AC determination of quebrachamine derivative was reported by Takayama and coworker.27 They assigned the AC of a natural product Kopsiyunnanine D by comparing its CD profile with those of a synthetic product derived from a compound of known AC.
Cleavamine derivatives are structurally related to quebrachamines. Parish and coworker stated in their report on the CD of vinblastine type bisindole compounds also of the CD of cleavamine derivatives 47 – 53.28 They observed that the sign of the first CE (c.a. 276 – 294 nm) can be correlated with the configuration of C-14 and that all the C-14S isomers, showed a negative CE.
H. OXINDOLE
The use of CD for the determination of the AC of C-7 of various types of oxindoles is well known. In 1966,29 Pousset and coworker reported the study of CD and its relation to the structure of oxindoles. They noted that the first and fourth CEs (in the ranges of 280 – 310 nm and 210 – 230 nm, respectively) and the C-7 configuration were correlated in those oxindoles with 3S configuration (those with 7R configuration gave a positive first and a negative fourth CEs). They also noted the relationship between the sign of the second CE (250 – 270 nm) and the configuration of C-3, and reported that those compounds where C-3 was R showed a positive CE. The correlation between the observed CD and the AC of C-7 and C-3 was further supported by a subsequent paper by Beecham and coworker.30 The study of rhynchophylline type compounds by Beckett and coworker reveals exceptions to the use of the previously proposed relationships.31 They observed that in the case of 9-hydroxy and 9-methoxy oxindole derivatives, the CEs have a different sign to the predicted one.
I. PSEUDOINDOXYL
The first observation of the correlation between the sign of the CD band around 400 nm and the AC of the spiroindoxyl center (C-2) was reported by Williams and coworkers. In their studies on the biogenesis of brevianamides A and B,32 they noted the relation between the CEs (250 – 400 nm) and the AC of the spyroindoxyl stereogenic center. Natural brevianamides A and B with an R configuration at the spyroindoxyl stereogenic center showed a positive CE followed by a negative CE in the region of 250 – 400 nm.32
Subsequent studies on the AC of pseudoindoxyl alkaloids by Takayama and coworker,33 reported the configuration assignment of some mitragynine related pseudoindoxyl alkaloids, i.e. mitragynine pseudoindoxyl (64), yohimbine pseudoindoxyl (65) and β-yohimbine pseudoindoxyl (66), by chemical correlation studies using indole alkaloids of known AC. They reported that the CD of the synthesized pseudoindoxyl compounds having 2S configuration gave a negative first CE (c.a. 400 nm) and a positive second CE, and also that the CD of a known pseudoindoxyl compound fluorocarpamine (67), interestingly, showed a CD curve antipodal to mitragynine pseudoindoxyl (64).
J. 3-HYDROXYOXINDOLE
Compared to the studies on the CD – AC relations of monoterpene indole alkaloids discussed above, the studies of 3-hydroxyoxindole AC were reported only recently. In 1999, Aimi and coworker reported the determination of C-7 AC of 3-oxo-7-hydroxy-3,7-secorhynchophylline (68 and 69) by comparing their CD with the CD of 3-hydroxytryptophan diastereomers of known ACs.34 L-Tryptophan derivative with 3S configuration (71) showed two negative CEs between 260 and 300 nm and a positive CE in the 220 – 260 nm region, whereas the one having 3R configuration (70) gave an entirely antipodal curve.
K. BISINDILE ALKALOIDS
In the case of bisindole alkaloids, having two indole chromophores, their CD curves are expected to have CEs related to the exciton coupling of the two chromophores. In the case of vinblastine (72) type bisindole alkaloids (indole – indoline chromophore), the result of the coupling is observed as an intense split CEs of opposite signs in the region of 200 – 230 nm and the sign of this split CEs may be used for the determination of the configuration of C-16’.28,35-36 In the case of bisnicalaterine A (73) (indole – indole chromophore), the coupling of the two indole chromophores results in the appearance of an intense split CEs of opposite signs with the split center at 230 nm.37
L. CIRCULAR DICHROISM CALCULATION AND INDOLE ALKALOIDS ABSOLUTE CONFIGURATION
CD calculations has been used for the determination of the ACs of natural products for decades, as seen in the π-SCF studies of CD by Mason and coworker.38 However, its use has been limited until the major advancements in the CD calculations by time-dependent density functional theory (TDDFT), which resulted in a good compromise between computational costs and accuracy, in the past decade came to pass.39-48 The principle of the determination of the ACs of natural products by CD calculation is relatively simple. It is basically to compare the calculated and experimental CD spectra to assign the ACs. If the two data closely match, assignment of higher reliability is to be obtained.
CD calculations generally involve two steps, a conformational analysis to obtain the conformer(s) and the UV/CD TDDFT calculation of the conformer(s). The conformational analysis is often done by Monte Carlo methods using molecular mechanics (MMFF94, etc.) and/or semi-empirical methods (AM1, etc.) for the relative energy evaluation of the conformers. The obtained conformers are then further optimized by using density functional theory (DFT) method before submitting it to the TDDFT calculations of the UV/CD using program such as Gaussian,49 TURBOMOLE,50 NWChem,51 or Spartan.52
The calculated UV/CD spectra of the conformers are Boltzmann averaged to obtain the UV/CD spectra of the isomers. The averaged UV spectrum is then shifted to conform to the experimental UV spectrum and the same shift is also applied to the calculated CD spectra before comparing the calculated CD spectra with the experimental CD of the natural product.
The accuracy of the TDDFT calculations itself mainly depends on the basis set and functional used for the calculations. Larger basis set generally increases the accuracy but also increases the computational time length. In the CD calculations, basis sets with polarization and diffuse functions are commonly used, for example 6-31G* or aug-cc-pVDZ, whereas the B3LYP functional is commonly used and performs well in general.53
Monoterpene indole alkaloids whose ACs were assigned recently by TDDFT calculated CD include actinophyllic acid (74),54 schizozygine (75),55 isoschizogaline (76),56 isoschizogamine (77),56 bisnicalaterines B and C (78 – 79),1 alsmaphorazine B (80)57 and eucophylline (81).58
ACKNOWLEDGMENTS
Our isolation work, including structure elucidation, was partly supported by a Grant-in-Aid for Scientific Research from the Ministry of Education, Culture, Sports, Science, and Technology of Japan, and a grant from The Open Research Center Project.
References
1. Y. Hirasawa, M. Hara, A. E. Nugroho, M. Sugai, K. Zaima, N. Kawahara, Y. Goda, K. Awang, A. H. A. Hadi, M. Litaudon, and H. Morita, J. Org. Chem., 2010, 75, 4218. CrossRef
2. M. Frederich, M. Tits, and L. Angenot, Trans. R. Soc. Trop. Med. Hyg., 2008, 102, 11. CrossRef
3. A. C. Mitaine-Offer, M. Sauvain, A. Valentin, J. Callapa, M. Mallié, and M. Zèches-Hanrot, Phytomedicine, 2002, 9, 142. CrossRef
4. A. E. Nugroho, M. Sugai, Y. Hirasawa, T. Hosoya, K. Awang, A. H. A. Hadi, W. Ekasari, A. Widyawaruyanti, and H. Morita, Bioorg. Med. Chem. Lett., 2011, 21, 3417. CrossRef
5. J. C. A. Tanaka, C. C. da Silva, I. C. P. Ferreira, G. M. C. Machado, L. L. Leon, and A. J. B. de Oliveira, Phytomedicine, 2007, 14, 377. CrossRef
6. G. A. Cordell, M. L. Quinn-Beattie, and N. R. Farnsworth, Phytother. Res., 2001, 15, 183. CrossRef
7. E. M. Silva, C. C. Cirne-Santos, I. C. P. P. Frugulhetti, B. Galvão-Castro, E. M. B. Saraiva, M. E. Kuehne, and D. C. Bou-Habib, Planta Med., 2004, 70, 808. CrossRef
8. K. Koyama, Y. Hirasawa, T. Hosoya, T. C. Hoe, K.-L. Chan, and H. Morita, Bioorg. Med. Chem., 2010, 18, 4415. CrossRef
9. C. Beemelmanns and H.-U. Reissig, Angew. Chem. Int. Ed., 2010, 49, 8021. CrossRef
10. R. B. Woodward, M. P. Cava, W. D. Ollis, A. Hunger, H. U. Daeniker, and K. Schenker, J. Am. Chem. Soc., 1954, 76, 4749. CrossRef
11. N. Finch, W. I. Taylor, T. R. Emerson, W. Klyne, and R. J. Swan, Tetrahedron, 1966, 22, 1327. CrossRef
12. W. Klyne, R. J. Swan, N. J. Dastoor, A. A. Gorman, and H. Schmid, Helv. Chim. Acta, 1967, 50, 115. CrossRef
13. L. Bartlett, W. Klyne, N. J. Dastoor, H. Schmid, J. Hrbek, and G. Snatzkec, Helv. Chim. Acta, 1971, 54, 1238. CrossRef
14. E. Seguin, M. Koch, A. Ahond, J. Gulhem, C. Poupat, and P. Potier, Helv. Chim. Acta, 1983, 66, 2059. CrossRef
15. K. Blaha, Z. Koblicova, and J. Trojanek, Collect. Czech. Chem. Commun., 1974, 39, 3168.
16. W. Kauzmann and H. Eyring, J. Chem. Phys., 1941, 9, 41. CrossRef
17. K. Blaha and J. Trojanek, Collect. Czech. Chem. Commun., 1973, 38, 929.
18. K. Blaha, K. Kavkoka, Z. Koblicova, and J. Trojanek, Collect. Czech. Chem. Commun., 1968, 33, 3833.
19. G. Toth, O. Clauder, K. Gesztes, S. S. Yemul, and G. Snatzke, J. Chem. Soc., Perkin Trans. 2, 1980, 701. CrossRef
20. S. Caccamese and G. Principato, J. Chromatogr., 2000, 893, 47.
21. K. Blaha, Z. Koblicova, and J. Trojanek, Collect. Czech. Chem. Commun., 1974, 39, 2258.
22. K. Bláha, Z. Koblicová, and J. Trojánek, Tetrahedron Lett., 1972, 13, 2763. CrossRef
23. J. P. Kutney, K. Fuji, A. M. Treasurywala, J. Fayos, J. Clardy, A. I. Scott, and C. C. Wei, J. Am. Chem. Soc., 1973, 95, 5407. CrossRef
24. W. Klyne, R. J. Swan, B. W. Bycroft, D. Schumann, and H. Schmid, Helv. Chim. Acta, 1965, 48, 443. CrossRef
25. W. Klyne, R. J. Swan, B. W. Bycroft, and H. Schmid, Helv. Chim. Acta, 1966, 49, 833. CrossRef
26. W. Klyne, R. J. Swan, A. A. Gorman, A. Guggisberg, and H. Schmid, Helv. Chim. Acta, 1968, 51, 1168. CrossRef
27. Y. Wu, M. Suehiro, M. Kitajima, T. Matsuzaki, S. Hashimoto, M. Nagaoka, R. Zhang, and H. Takayama, J. Nat. Prod., 2009, 72, 204. CrossRef
28. C. A. Parish, J.-G. Dong, W. G. Bornmann, J. Chang, K. Nakanishi, and N. Berova, Tetrahedron, 1998, 54, 15739. CrossRef
29. J. L. Pousset, J. Poisson, and M. Legrand, Tetrahedron Lett., 1966, 7, 6283. CrossRef
30. A. F. Beecham, N. K. Hart, S. R. Johns, and J. A. Lamberton, Aust. J. Chem., 1968, 21, 491. CrossRef
31. W. F. Trager, C. M. Lee, J. D. Phillipson, R. E. Haddock, D. Dwuma-Badu, and A. H. Beckett, Tetrahedron, 1968, 24, 523. CrossRef
32. R. M. Williams, E. Kwast, H. Coffman, and T. Glinka, J. Am. Chem. Soc., 1989, 111, 3064. CrossRef
33. H. Takayama, M. Kurihara, S. Subhadhirasakul, M. Kitajima, N. Aimi, and S. Sakai, Heterocycles, 1996, 42, 87. CrossRef
34. H. Takayama, T. Shimizu, H. Sada, Y. Harada, M. Kitajima, and N. Aimi, Tetrahedron, 1999, 55, 6841. CrossRef
35. J. P. Kutney, D. E. Gregonis, R. Imhof, I. Itoh, E. Jahngen, A. I. Scott, and W. K. Chan, J. Am. Chem. Soc., 1975, 97, 5013. CrossRef
36. J.-G. Dong, W. Bornmann, K. Nakanishi, and N. Berova, Phytochemistry, 1995, 40, 1821. CrossRef
37. A. E. Nugroho, Y. Hirasawa, N. Kawahara, Y. Goda, K. Awang, A. H. A. Hadi, and H. Morita, J. Nat. Prod., 2009, 72, 1502. CrossRef
38. W. S. Brickell, S. F. Mason, and D. R. Roberts, J. Chem. Soc. B, 1971, 691. CrossRef
39. X.-C. Li, D. Ferreira, and Y. Ding, Curr. Org. Chem., 2010, 14, 1678. CrossRef
40. C. Diedrich and S. Grimme, J. Phys. Chem. A, 2003, 107, 2524. CrossRef
41. P. J. Stephens, D. M. McCann, E. Butkus, S. Stončius, J. R. Cheeseman, and M. J. Frisch, J. Org. Chem., 2004, 69, 1948. CrossRef
42. P. J. Stephens, D. M. McCann, F. J. Devlin, J. R. Cheeseman, and M. J. Frisch, J. Am. Chem. Soc., 2004, 126, 7514. CrossRef
43. W. Schühly, S. L. Crockett, and W. M. F. Fabian, Chirality, 2005, 17, 250. CrossRef
44. D. M. McCann and P. J. Stephens, J. Org. Chem., 2006, 71, 6074. CrossRef
45. T. D. Crawford, M. C. Tam, and M. L. Abrams, J. Phys. Chem. A, 2007, 111, 12057. CrossRef
46. P. J. Stephens, F. J. Devlin, F. Gasparrini, A. Ciogli, D. Spinelli, and B. Cosimelli, J. Org. Chem., 2007, 72, 4707. CrossRef
47. Y. Ding, X.-C. Li, and D. Ferreira, J. Org. Chem., 2007, 72, 9010. CrossRef
48. G. Bringmann, T. Bruhn, K. Maksimenka, and Y. Hemberger, Eur. J. Org. Chem., 2009, 2717. CrossRef
49. Gaussian09. http://www.gaussian.com.
50. TURBOMOLE 6.3. http://www.turbomole.com.
51. NWChem 6.0. http://www.nwchem-sw.org.
52. Spartan'10. http://www.wavefun.com.
53. N. Berova, L. D. Bari, and G. Pescitelli, Chem. Soc. Rev., 2007, 36, 914. CrossRef
54. T. Taniguchi, C. L. Martin, K. Monde, K. Nakanishi, N. Berova, and L. E. Overman, J. Nat. Prod., 2009, 72, 430. CrossRef
55. P. J. Stephens, J.-J. Pan, F. J. Devlin, M. Urbanová, and J. Hájíček, J. Org. Chem., 2007, 72, 2508. CrossRef
56. P. J. Stephens, J.-J. Pan, F. J. Devlin, M. Urbanová, O. Julínek, and J. Hájíček, Chirality, 2008, 20, 454. CrossRef
57. K. Koyama, Y. Hirasawa, A. E. Nugroho, T. Hosoya, T. C. Hoe, K.-L. Chan, and H. Morita, Org. Lett., 2010, 12, 4188. CrossRef
58. J. Deguchi, T. Shoji, A. E. Nugroho, Y. Hirasawa, T. Hosoya, O. Shirota, K. Awang, A. H. A. Hadi, and H. Morita, J. Nat. Prod., 2010, 73, 1727. CrossRef