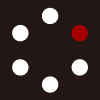
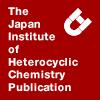
HETEROCYCLES
An International Journal for Reviews and Communications in Heterocyclic ChemistryWeb Edition ISSN: 1881-0942
Published online by The Japan Institute of Heterocyclic Chemistry
e-Journal
Full Text HTML
Received, 29th June, 2012, Accepted, 6th September, 2012, Published online, 12th October, 2012.
DOI: 10.3987/REV-12-SR(N)4
■ Asymmetric Reactions of a Series of Aromatic Azines with Nucleophiles
Ilya N. Egorov,* Tatyana A. Tseitler, Grigory V. Zyryanov, Vladimir L. Rusinov, and Oleg N. Chupakhin
Department of Organic Chemistry, Ural Federal University, Mira St. 19, Ekaterinburg 620002 , Russia
Abstract
Current review is devoted to the reactions of nucleophilic addition in azine series, leading to the stereoselective formation of optically active products. Three types of reactions are reviewed: the reactions of achiral nucleophiles with chiral azines; the reactions of chiral nucleophiles with achiral azines; reactions of achiral nucleophiles with achiral azines in the presence of chiral catalysts.INTRODUCTION 2
I. Asymmetric induction mediated by the attack of achiral nucleophile on the chiral azine 2
1. Interaction of azines, containing a chiral substituent next to the nitrogen atom 3
1.1. Addition reactions of trimethylsylil cyanide (TMSCN) 3
1.2. Addition reactions of organo-Li, organo-Mg and organo-Zn compounds as C-nucleophiles 6
1.3. Addition reactions of metalloenolates 24
1.4. Addition reactions of Sn-organic compounds 26
1.5. Addition reactions of Si-organic compounds 26
1.6. Addition reactions of N-nucleophiles 27
1.7. Addition reactions of CH-active compounds 29
2. Interaction of azines, containing a chiral substituent next to the cyclic carbon atom 36
II. Stereoselective addition of an optically active nucleophile to an achiral azine 49
III. Asymmetric induction in the attack of an achiral nucleophile at an achiral azine 52
CONCLUSION 64
INTRODUCTION
Reactions of nucleophilic substitution of hydrogen (SNH) in a series of π-deficient (hetero)aromatic compounds, azines in particular, are known to belong to a wide class of two-stage processes, proceeding via addition-elimination sequence.1 The general feature of these reactions is the initial formation of nucleophilic addition adducts, i.e. σH-adducts, as a result of direct attack of С-, N-, O-, P-, S- and other nucleophiles at the unsubstituted cyclic carbon (Scheme 1). During the formation of σH-adducts the prochiral sp2-atom of carbon at C=N bond of an azine cycle becomes sp3-hybridizated and thus the whole molecule becomes chiral. In the presence of a chiral catalyst the reaction can give the addition products, enriched by one stereoisomer. When chiral carbon atom presents either in substrate or in nucleophile the reaction also can proceed stereoselectively to afford preferably only one (dia)stereoisomer (Scheme 1). The detailed study of approaches towards azine containing chiral elements is of great practical interest for obtaining enantiomerically pure alkaloids or their analogs.2
The review describes reactions of nucleophilic addition in azine series, afforded the enantiomerically/diastereomerically enriched reaction products. In the first part of the review we report about interactions of achiral nucleophiles with chiral azines, the second part describes interactions of chiral nucleophiles with achiral azines, and the third part describes interactions of achiral nucleophiles and achiral azines, catalyzed by chiral catalysts.
We have considerably modified our previous review3 published in 2005 with the data described in the literature in last twenty years. In addition, the review summarizes and completes the data, reported in review,4 which describes in details reactions of pyridinium salts, as well as review,5 concerning the Reissert reactions, mediated by chiral catalysts.
I. ASYMMETRIC INDUCTION MEDIATED BY THE ATTACK OF ACHIRAL NUCLEOPHILE ON THE CHIRAL AZINE
Pyridine-type nitrogen atom increases the π-deficiency of an azine heterocycles, however only few examples of direct introducing of residues of neutral C-nucleophiles into non-activated azine cycle are known.1 To induce the chirality during the nucleophilic attack an activated azine substrates, i.e. azinium cations, and/or highly reactive nucleophiles, i.e. organometallic compounds, are commonly used. In current part some typical examples of interactions of azines and other C-nucleophiles (i.e. cyanides, enolates and indoles) and Si-nucleophiles are described.
1. Interaction of azines, containing a chiral substituent next to the nitrogen atom
The reactions of C-nucleophiles with azinium cations containing nitrogen atoms quaternised with chiral substituents have been studied pretty well. The corresponding salts are usually synthesized by alkylation or acylation (often in situ) of an azine substrate with an optically active reagent (Scheme 2).
The fragments of chiral hydrocarbons, alcohols (including natural ones), amino acids and heterocyclic compounds may be used as the asymmetric inductors. The data in this Section are presented according to the type of the nucleophilic agent and in the order of increasing of complexity of the azine substrate.
1.1. Addition reactions of trimethylsylil cyanide (TMSCN)
In publication6 stereoselective additions of cyanide anion to chiral isoquinolinium salts have been studied. The parent azinium salts were generated in situ by the acylation of isoquinoline (1a) by chiral amino acids fluoroanhydrides (2a,b,3,4) in the presence of Lewis acids. Trimethylsylil cyanide (TMSCN) was utilized as a C-nucleophile.6 As a result the stereoselective formation of Reissert compounds 6а-d was observed. The diastereomeric ratio of the reaction products depends on the structure of the original amino acid (Scheme 3).
Chiral acylazinium salts 9 or 11 were synthesized by N-acylation of quinoline (7a) or isoquinoline (1a) using (S)-4-benzyl-2-oxooxazolydine-3-carbonyl chloride (8). The reaction of the salts 9 or 11 with cyanide-anions, generated in situ from trimethylsilyl cyanide (TMSCN), has resulted in Reissert compounds 10, 12 in moderate degree of diastereoselectivity (Schemes 4, 5).7 According to the authors the possible steric hindrance from the one side of hetarene cation plane, caused by π-π-interaction with benzyl moiety of chiral substituent is the main reason for the observed diastereoselectivity. The triflate anion of acylazinium salt was noticed to facilitate the π-π-interactions in the acylazinium intermediate due to the weaker interaction with the iminium part of the molecule than in the case of the chloride anion. As a consequence, the stereoselectivity of the addition increases.7
Acylation of isoquinoline (1а) by (–)-(R)-menthyl chloroformate (13a), or by its analogues 13b–d in DCM followed by the addition of TMSCN has been shown to afford products 14a–d as racemic mixtures. Low degree of diastereoselectivity was observed only when cholesterol chloroformate was applied (13d).8
1.2. Addition of organo-Li-, organo-Mg- and organo-Zn compounds as С-nucleophiles
Reaction of 4-phenylpyridine (15) and (1S,4R)-4,7,7-trimethyl-3-oxo-2-thia¬bicyclo[2.2.1]heptan-1-carbonylchloride (16) in situ gave the N-acylazinium salt 17, the latter reacted with phenylmagnesium bromide to form compound 18 in 50% yield. No diastereoselectivity was observed (Scheme 7).9
Isoquinoline (1a), acylated by (S)-α-Cbz-aminoacyl fluorides 19a,b in reactions with arylmagnesium bromides resulted in the compounds 20a,b in various degree of diastereoselectivity (Scheme 8).10
Interaction of isoquinoline (1a) with (–)-(R)-menthyl chloroformate (13a) was shown to give isoquinolinium salt, which further reacted with Mg- or Zn-organic arenes to form products 21a–c in moderate diastereoselectivity (Scheme 9).10
In order to explain the diastereoselectivity in the reaction of isoqinolines with metalloorganic compounds the authors have proposed the plausible pathway for the formation of 21. According to the pathway R–M interacts with oxygen atom of in situ formed N-acylazinium salt to result in rigid intermediate state, blocking the unfavorable formation of rotamers along the N–CO bond (Figure 1).10
Azines can be quaternised not only by chiral acyl groups, but by alkyl groups as well. Thus chiral N-alkylpyridinium salts 22a,b show a high degree of diastereoselectivity in reactions with benzyl-type Grignard reagents.11 The reduction of the products with sodium borohydride leads to the diastereomeric tetrahydropyridines 23a,b (Scheme 10).
Such a relatively high selectivity was rather unexpected for the authors,11 because the chiral alkyl group in the starting salt 22a,b is quite mobile and relatively freely rotates around the N-С bond.
The structures A and B, in which the С-N bond of the chiral group is coplanar to the plane of the pyridine ring, are probably the most energetically favorable conformations of the salts 22a,b. In such a case, there are several possible directions of the nucleophilic attack: pathways a and b for the conformation A and pathways с and d for the conformation B. But only the pathway с leads effectively to the stereoisomers 23, because in this case the attack of the nucleophile to the pyridine ring is less hindered by the small- (H atom) and medium-sized (methyl group) substituents of the chiral alkyl fragment (Scheme 11).11
The reaction of the chiral isoquinolinium salts 24 with the Grignard reagents afforded unstable 1-substituted 1,2-dihydroisoquinolines 25 and 26 in good yields (90-95%) (Scheme 12).11 However, the observed diastereoselectivity of this reaction was lower than that in the case of 3,4-disubstituted pyridinium salts.
In a similar reaction of the Grignard reagents with the isoquinolinium salt 27, containing a chiral hydroxy(phenyl)ethyl group at the nitrogen atom, the 1,2-dihydroisoquinoline intermediates 28 cyclised spontaneously upon hydrolysis.12 The main reaction products were tetrahydrooxazoloisoquinolines 29 and 30. Small amounts of dihydroisoquinolines 31 were also isolated (Scheme 13).
Presumably,12 the destabilizing steric interactions between the phenyl groups of the oxazolidine ring and the substituent R at C(5) are responsible for the preferable formation of the oxazolidines 29 rather than of their stereoisomers 30.
It has been shown, that interaction of the pyridinium salt 32 with Grignard reagents results in unstable addition products 33a, hydrolyzing further to the compounds 34, which were reduced to 35 (Scheme 14). There are no data for diastereomeric purity for compounds 34a,b. Their reduction products 35a were reported to have de = 54 - 80.13
An example of PrnMgBr addition to chiral pyridinium ylide 36. Reaction proceeds regioselectively and with high degree of diastereoselectivity (dr = 84 : 16) (Scheme 15).14
An example of diastereoselective addition of C-nucleophile to isoquinoline 38, activated by a chiral rhenium complex, has been described.15 The reactions of compound 38 with organometallic compounds occur under very mild conditions and start at temperature as low as -100 °C. The metallocomplex 39 is the main reaction product (Scheme 16).
The reaction with (CH3)3SiCH2MgCl proceeds much slower in comparison with (CH3)3SiCH2Li. In the first case the diastereoselectivity decreases when the temperature increases. Thus, Grignard reagent (CH3)2CHMgCl intensively reacts with 38 even at -100 °С. It should be noted that CH3MgCl was the less active nucleophile in this reaction.15
A. B. Charette and co-authors have published a wide scope of works concerning the investigation of reactions of chiral N-imidoylpyridinium salts with Grignard reagents. Thus, salt generated from pyridine and (S)-valynol amide 42 reacted with Grignard reagents forming the addition products 44 in high degree of diastereoselectivity (dr > 95:5).16 In reactions with PhMgBr the yield and the diastereoselectivity were improved by adding LiBr, and diethyl ether as a solvent (89%, dr > 98:2) (Scheme 17).17
A vacant electron pair of nitrogen atom in imidate 43 effectively governs the nucleophilic attack at the position 2 of pyridine ring. The stereoselective addition to unsubstituted pyridinium salts is complicated by existence of four directions of nucleophilic attack at the pyridine ring (Figure 2). The bulky substituent at R1 prevents the nucleophilic attack at position 2’. However, neither this substituent, nor the substituent at the imidate nitrogen atom can prevent the formation of (E)-imidate. The authors have demonstrated, that the introduction of phenyl group as R1, as well as the introduction of bidentate auxiliary reagent, derived from valinole, provides excellent regio- and stereoselectivity upon the addition of nucleoplies.16
Imidates formation was utilized in a synthesis of derivative of chiral phenylphosphonium salts. As a result the following addition products 47a,b were obtained with high diastereoselectivity (Scheme 18).18
In addition, some reactions of nucleophilic addition to imidates 48 derived from 4-methoxypyridine 41a and (S)-valinole amide 42 were described. It was shown that N-imidoylpyridinium salt 48 was attacked by nucleophiles leading to 49 with high diastereoselectivity (Scheme 19).19
J. Streith and co-authors have studied the reaction of 4-methoxypyridine (15) with Grignard reagents. They found that the introduction of chiral oxazoline into 4-methoxypyridine and the following addition of nucleophiles leads to the products 51a,b with high diastereoselectivity (Scheme 20).20
A series of papers studying the influence of chiral substituents at the nitrogen atom of azine substrates on the stereoselectivity of the reactions with organometallic reagents have been published by the Comins group. Thus 1-acylpyridinium salts 53 containing a residue of an optically active alcohol (R*OH) have been studied. 21-25 These salts have been synthesized from 4-methoxy-3-(triisopropylsilyl)pyridine (52) and the corresponding chiral chloroformates. The salts 53 react with organomagnesium compounds under very mild conditions.21 Acid hydrolysis of the reaction mixture yields mainly dihydropyridones 54. The stereoselectivity of the reaction is relatively high, stereoisomers 55 are the minor products.
The effect of chiral acyl groups on the stereoselectivity of the addition reaction may be increased by introducing of aromatic substituents into the alcohol residue.23,24 Thus the stereoselectivities of the reactions of trans-2-(6-cumyl)cyclohexyl derivatives of the salts 53c-k with p-tolyl- and cyclohexylmagnesium halides were equal to 83-93%. Acylpyridinium salts 53i-k, containing an isopropyl group in a similar chiral substituent, appeared to be slightly more effective as asymmetric inductors in this stereoselective synthesis (Scheme 21, Table 1).21
Presumably, it is the steric shielding of one side of the pyridinium ring of salt 53 by bulky chiral acyl groups that determines the predominant direction of the nucleophilic attack. The process is enhanced by aryl substituents in these groups. The π-π-donor-acceptor interaction (further in the text, π-π-interaction) between the substituent and the pyridinium cation stabilizes the staggered conformation.27,28 The positive effect of the isopropyl group of the 8-arylmenthyl moiety on the stereoselectivity of the process is more difficult to rationalize. It was suggested24,27 that the isopropyl group might affect the ratio of the possible rotamers leading to the optimum π-π-interaction between the aromatic rings (Figure 3).
The absence of stereoselectivity in the reaction of phenylmagnesium bromide with the salt 53l where vic-substituents in the cyclohexane ring adopt a cis-configuration may be explained by the same reason.
Pyridines are able to attach nucleophiles at various positions. Therefore in order to provide the high regioselectivity Comins and co-authors utilized the pyridine derivatives, substituted in a position C(4) by methoxygroup and in a position C(3) by triisopropylsylil group.
The most detailed investigation of the effect of the character and size of the 3-substituent in the pyridine ring on the diastereoselectivity of the addition of the Grignard reagents was carried out by Comins et al.24 The authors studied the reactions of a series of optically active 4-methoxy-N-[(-)-menthyloxycarbonyl]pyridinium salts 53a,b,m-v with arylmagnesium chlorides leading to the 6-substituted compounds 54 and 55 (Scheme 22, Table 2).
The original idea23 was to increase the regioselectivity of the reaction by introducing of a 3-substituent into the pyridinium ring, which would prevent nucleophilic attack on C(2). However, the substituent has been shown to affect the stereochemistry. Although no rigorous regularities were established, some conclusions could be drawn. The best diastereoselectivity (de 94%) was obtained in the reaction of 4-methoxy-3-(triisopropylsilyl)pyridinium salt 53b with phenylmagnesium chloride. The use of pyridinium salts with less bulky 3-alkylsilyl substituents in the reactions with aromatic Grignard reagents resulted in a decrease in de (in the range of 34-40%). In the case of triphenylsilyl substituent the diastereoselectivity of the process fell down up to 18%.23
In the most of his works Comins and co-authors utilize the pyridine derivatives, substituted in position C(4) by methoxy group and in position C(3) by triisopropylsylil group, while using the (+)/(-)-TCC as a chiral auxiliary group. Thus, acylation of pyridine 52 by chloroformate of (+)-trans-2-(6-cumyl)cyclohexanole ((+)-TCC) resulted in acylpyridinium salt 53g, which reacted with Grignard reagents RX to form the addition products 56-60 (Scheme 23).29-33 In the same manner some semi-products for the synthesis of benzomorphane alkaloids,29 (+)-luciduline,30 indolyzidin alkaloids (-)-205A, (-)-207A, и (-)-235B32 and trans-decahydroquinoline alkaloid (+)-219A33 were obtained.
Acylation of pyridine 43 chloroformate of (-)-trans-2-(6-cumyl)cyclohexanole ((-)-TCC) gave pyridinium salt 44h, which reacted with Grignard reagents RX to afford the addition products 52-54, utilized further for the synthesis of such alkaloids, as (−)-septicine,34 (−)-tylophorine34 and phlegmarines35 (Scheme 24).
Unsubstituted indoles proved not to react with 1-(benzyloxycarbonyl)-4-methoxy-3- (triisopropylsylil)pyridine. Organo-Mg derivatives of indole easily reacted with 1-acylpyridinium salts 53h,g (R* = (+)/(-)-trans-2-(α-cumyl)cyclohexyl, (+)/(-)-TCC) to form the addition products 64-66a,b with moderate diastereoselectivity (Scheme 25).36
Application of Li acetylenides instead of alkenylmagnesium bromides results the addition products with the same stereoconfiguration. Thus, treatment of salt 53g with Li-derivative of ethyl propiolate leads to dyhydropyridone 68 (Scheme 26).37
Introduction of lithium ethyl propiolate in reaction with pyridinium salt 69 results in dyhydropyridone 70, which was successfully utilized in a synthesis of (+)-allopumiliotoxin 267A (Scheme 27).38
The reaction of 3-(triisopropylsilyl)pyridine (71) with chiral bicyclic acyl bromide 72a in pyridine yields solid N-acylpyridinium salt 73a.39 The addition of 2-(l,3-dioxolan-2-yl)ethylmagnesium bromide 74 at -90 °C to this heterogeneous system affords 6-substituted N-acyl-l,6-dihydropyridine 75. The reaction is regio- and diastereoselective (yield 41%, de = 100%). An analogous reaction was carried out with acyl chloride 72b under homogeneous conditions, which are more suitable for this reaction. In the presence of trimethylsilyl triflate (TMSOTf), the addition product 75 is formed via intermediate salt 73b. The yield of the product increased to 62%, but the diastereoselectivity of the process dropped down to 88% (Scheme 28).39
The asymmetric induction in the considered processes may be explained by preliminary complex formation. The reaction mechanism includes the initial coordination and addition of organometallic reagent to the carbonyl group of the chiral acyl substituent with formation of complex (Figure 4).39 Judging from the stereochemistry of the addition product 75, the attack of the nucleophilic agent on the iminium moiety proceeds as follows:
Acylation of pyridines 15, 76 with chloroanhydride of bicyclolactonecarboxylic acid 77 also results in the formation of the corresponding N-acylazinium salts 78. The salts were unreactive to PhMgBr, therefore an additives of various triflates were utilized in order to replace the chloride-anion in 78. The reactions with Grignard reagents afford the addition products 79-82 in moderate yields and stereoselectivity. The replacement of Grignard regents by organocuprates increases the yield but decreases the diastereoselectivity of the reaction. Introduction of Pri3SiOTf instead of Me3SiOTf slightly increases the reaction yield. Along with that the increasing of the concentration of salt 78 from 0.1 to 0.2М causes the noticeable growing of the reaction yield either (Scheme 29, Table 3).
Reaction of 4-methoxypyridine 41a with sultam chloroanhydride 83 generates in situ N-acylazinium salt 84, which further reacts with Grignard reagents to afford compounds 85–88 (Scheme 30).41
1.3. Addition reactions of metalloenolates
The addition reactions of prochiral metalloenolates of ketones and lactones with salts 53h are highly stereoselective and result in the formation of two new chiral centers. The 2R,2'S-diastereomer 89 was the main reaction product, while (R,R)-, (S,R)- and (S,S)-stereoisomers were obtained in minor amounts (Scheme 31).42
Interestingly, the reaction with the enol form of 2,2-dimethyl-l,3-dioxan-5-one (E-isomer) proved to yield compound 90 with (2S,2'S)-configurations of chiral centers. Compound (2S,2'R)-91 with the opposite configuration is formed as the main product in the similar reaction with l,3-bis(benzyloxy)propan-2-one in the enol form, which exists as Z-isomer due to the formation of chelate. Dihydropyridones 90 and 91 are formed with enolate facial selectivities of 9/1 and 4/1, respectively (Scheme 32).42
The selectivity of the addition of E-enolate can be explained by existence of the transition state (Figure 5).42
This transition state is the most probable due to the noncovalent interaction between the pyridinium ring and the phenyl ring of the chiral acyl group (π-π-interaction) and also due to the electrostatic attraction between the positively charged nitrogen atom and the negatively charged enolate oxygen atom.42
Similar reaction of N-acylpyridinium salt 53g and acetone Zn enolate leads to N-acyldyhydropyridone 92 in 72% yield (Scheme 33).43
Also the formation of the enantiomerically pure N-acylpyridone 94 by the reaction of pyridinium salt 94g with Zn enolate 93 was described. The N-acylpyridone 94 was used as a semi-product in the synthesis of (+)-cannabisativine (Scheme 34).44,45
1.4. Addition reactions of Sn-organic compounds
During the development of asymmetric synthesis of 1-substituted tetrahydro-β-carbolynes the interaction of 3,4-dyhydro-β-carboline 95a and allylbuthyltin was investigated. As a result the addition product 96a was isolated in high yield, but in low degree of stereoselectiviry. Upon the introduction of phenyloxycarbonyl moiety at the position C(9) of carbolyne the stereoselectivity of the reaction was improved, but the yield fell down (Scheme 35).47
1.5. Addition reactions of organo-Si compounds
The reaction of triphenylsilylmagnesium bromide (Si-nucleophile) with chiral N-acyl-4-methoxypyridinium salts 53 at -78 °C is stereoselective and results in diastereomerically pure N-acyl-2-(triphenylsilyl)-2,3-dihydropyridin-4-ones 97 (Scheme 36). The absolute configuration of the newly formed chiral center C(2) of dihydropyridones 97a-f appeared to be opposite to that of the main reaction product of pyridinium salts 53 with arylmagnesium chlorides as C-nucleophiles (see above21,23-25,34,35).48
The preferential formation of S-isomer is explained48 by the interaction of the magnesium atom of the Grignard reagent with the carbonyl group of the acyl fragment (Figure 6). This coordination results in the alteration of the direction of the nucleophilic attack, which was confirmed by quantum-chemical calculations.
1.6. Addition reactions of N-nucleophiles
Acylation of azaheterocycles by carboxylic acids derivatives affords the corresponding N-acylazinium salts. Acylation of isoquinoline 1a by amino acid fluoroanhydrides 98a,b, 99 in the presence of catalytic amounts of AlCl3 and TMSCl leads to the expected N-acylation, but the corresponding N-(β-aminoacyl)iminium salts do not form. Instead the further cyclisation occurs by the nucleophilic attack of NH-group at the iminium carbon atom, i.e. at the position C(1) of isoquinolinium ring thus to give 1,10-β-dyhydroimidazo[2,1-a]isoquinolin-3(2Н)-ones 100a,b, 101 as enantiopure products (Scheme 37).49
Acylation of isoquinolines 1a,b by means of fluoroanhydrides of β-sulphonylamino acids also postulates the formation of acylisoquinolinium intermediates and the following nucleophilic attack is sterically hindered from one side of an azine cycle. Despite the presence of TMSCN, i.e. competitive nucleophile, in the reaction mixture the intramolecular nucleophilic attack of amino group at the position C(1) of isoquinoline generates only products 103a-i. Only upon the introduction of bulky fluoroanhydride of pentamethylbenzofurylsulphonylalanine, unable to form the cyclization products, the corresponding cyanide-anion addition product 102 was isolated. Substituents at the position C(3), especially at the position C(1) of isoquinoline cycle, cause steric hindrances for the formation of dihydroimidazoisoquinoline 103a–i. Thus, 3-methylisoquinoline 1b forms the corresponding reaction product 103i in lower yield (12%), and 1-methylisoquinolin or 6,7-dimethoxy-1-methylisoquinoline were unable to react at the same conditions (Scheme 38).50
Investigations of some Lewis acids, utilized for the activation of amino acid fluoroanhydrides in this reaction, demonstrated the higher efficiency of AlCl3 in comparison with ZnCl2, ZnF2 or Et2AlCl.50
1.7. Addition reactions of CH-active compounds
N-Acylazinium salts generated in reactions of aromatic azaheterocycles and chiral carboxylic acids are able to react with CH-active compounds, such as anilines, indoles, pyrroles, thiophenes.
Thus, in reactions of isoquinoline (1a), acylated by (S)-6-Cbz-aminoacyl fluorides, and π-excessive hetarenes a mixture of 1-aryl-2-acyl-1,2-dyhydroisoquinolines 104a–g and imidazoisoquinolines 105 was obtained. In most cases the reaction proceeds in high degree of diastereoselectivity (dr 2:1 – 6:1 according to NMR 1H data) (Scheme 39, Table 4).10
Interaction of isoquinoline (1a) with (–)-(R)-menthyl chloroformate 13a followed by the addition of π-excessive hetarenes such as anilines, indoles, pyrroles, thiophenes leads to a corresponding 2-acyl-1-aryl-1,2-dihydroisoquinolines 106 in yields 33–97%. No diastereoselectivity was observed in these reactions (Scheme 40).10
Acylation of isoquinoline (1a) by chloroanhydride of N-phthaloyl-(S)-valine and followed reaction with furan affords compounds 107a–d. The absolute configuration was not determined by authors (Scheme 41).51
During the investigations of 1,2,4-triazinone as substrate for an asymmetric synthesis it was found that 3-Ar-1,2,4-triazin-5-one 108 reacted with indoles after acylation by N-protected α-amino acids.21 The interaction results in the addition products 109a-p in high diastereoselectivity. The preferable formation of one pair of diastereoisomers can be explained by the possible steric hindrances for the nucleophilic attack of triazine ring by indole, caused by bulky substituents at the α-carbon of amino acid. Besides the steric hindrances is the main reason for low yields in this reaction (Scheme 42, Table 5).52
It is worth mentioning, that 3-aryl-1,2,4-triazin-5(4H)-ones 108, in contrast to pyridines, quinolines or isoquinolines, which were mentioned above, are able to form the addition products with C-nucleophiles not only in the presence of acylation agents, but in the presence of acids. Authors suppose that from two possible pathways for the formation of compounds 109, the reaction runs diastereoselectively only in case В (Scheme 43).52
In reactions of triazines 108 and C-nucleophiles at the presence of chloroanhydrides of N-Ts-L-valine or N-Ts-L-leucine some 6-aryl-1-(2-tosylamino)acyl-3,4-dyhydro-1,2,4-triazin-5(4H)-one 110a–g were obtained in yields from 15 to 77% (Scheme 44). If N-Ts-L-valine was utilized as an acylating agent the diastereoselectivity of the reaction was the highest. According to NMR 1H the content of SS-isomer 110c,f in reaction mixtures was 90–95% (Scheme 44, Table 6).53
The asymmetric induction can be explained by the formation of N-acylazinium salt А, there is the dipole-dipole interaction between the nitrogen atom of amino group of amino acid and the electrophilic carbon atom of heterocycle. This interaction forms sterical hindrances for the nucleophilic attack from one side of heterocyclic ring plane (Scheme 45).53
In reactions of triazinone 108 with substituted phenols in the presence of chloroanhydride of N-Ts-L-valine the formation of products 110 does not occur, the reaction results in the water addition products at the position C(6) of triazine.54
In reaction of 6-phenyl-1,2,4-triazin-5(4H)-one 112 with C-nucleophiles in the presence of amino acids chloroanhydrides the formation of some 3-substituted 2-(2-tosylamino)acyl-6-phenyl-3,4-dihydro- 1,2,4-triazin-5(4H)-ones 113a-d occurs in moderate yields and in low diastereoselectivity (Scheme 46).53
Reaction of 6-phenyl-1,2,4-triazin-5(4H)-one 112 with C-nucleophiles in the presence of N-substituted L-amino acids and ethyl chloroformate leads to 3-substituted 2-(2-acylamino)acyl-6-phenyl-3,4-dihydro- 1,2,4-triazin-5(4H)-ones 113e–i obtained as a pair of (SS,RR)-diasterereomers in moderate yields, but in high diastereoselectivity (Scheme 47).55
Activation of amino acids via generation of esters leads to racemization therefore the reaction products 113e–i are racemic mixtures even if enantiopure amino acids are utilized in the reaction. Upon the introduction of naproxen 114 instead of amino acids the reaction affords product 115 (dr = 98:2 according to HPLC) (Scheme 48).55
2. Interaction of azines, containing a chiral substituent next to the cyclic carbon atom
The main factor determining the diastereomeric ratio of products of nucleophilic attack at the carbon atom of C=N of an azine, is the presence of any substituents in the azine ring, which can coordinate with the attacking nucleophile. If R* bears chirality it can direct the reaction by the way favoring the formation of one or another possible diastereomeric products (Scheme 49).
One of the first reported reactions of diastereoselective nucleophilic addition to pyridinium and quinolinium salts with initial complexation is the reaction of chiral 2-(3-pyridyl)- (116a) and 2-(3-quinolyl)oxazolines (116b) with organolithium and organomagnesium reagents followed by treatment with methyl chloroformate. This reaction affords the 1,4-dihydro-derivatives 117a-d and 118a-d in good yields and high diastereomer ratio (Scheme 50, Table 7).56-59
The high stereoselectivity of the addition was explained by initial coordination of an organometallic compound (RM or RMX) to the nitrogen atom and the methoxy group of oxazoline, which leads to the formation of complex A followed by the attack of the group R on the position C(4) of the pyridine ring. The addition of RM (or RMX) from different sides of the plane of the ring (paths a and b) results in products with different stereochemistry. High stereoselectivity of the studied reactions implies that the most common reaction pathway is the path a. It was suggested that lithium or magnesium derivatives B, formed upon addition of organometallic compound RM (or RMX) to pyridine 116a, are acylated with methyl chloroformate to afford compound 117 (Scheme 51).56
Pyridinium salts 119a,b reacted with lithium organocuprates. After acylation of the reaction mixture with trichloroacetic acid anhydride the expected adducts 120a,b and 121a,b were isolated (Scheme 52).61 In addition authors consider the possibility of utilizing of such CH-active compound, as 1-Me-2-acetylindole as a nucleophile in this reaction. This indole attacks the position C(4) of pyridine ring by the acetyl group to form the addition product in low yield and diastereoselectivity (yield 30%, dr = 2.1:1).61
Comins and co-authors have studied the behavior of nicotine 122, activated by pivaloyl chloride in reactions with nucleophiles. After the addition of organocuprates the addition products 123a-h were isolated (Scheme 53).62
Pyridines 124 bearing the chiral aminal in position C(3) are able to react with organocuprate with various stereoselectivities. Reaction affords the mixture of 1,4- and 1,2-addition products 125 and 126 respectively (Scheme 54, Table 8).63,64
In order to explain the observed regio- and stereoselectivity authors supposed the existence of hypothetic transition state in which the organocuprate is linked with either acetyl chloride or methyl chloroformate (Figure 7).63
One more example of regio- and stereoselective addition of allylic organometallic compounds to pyridinium or quinolinium π-complexes was described. Allylation of pyridinium or quinolinium salts by allylindium or allyltin reagents affords 1,2- or 1,4-adducts with high regio- or stereoselectivity. Pyridine 127 acylation and following attack by allylic nucleophile results in a mixture of 1,2-, 1,4- and 1,6-addition products a, b and c correspondingly (Scheme 55, Table 9). It was demonstrated that Zn- and Sn-containing nucleophiles leads to the preferable formation of 1,2- and 1,6-addition products, at the same time organoindium compounds preferably result in 1,4-addition products.68
Authors suspect, that π-π-interaction between the aromatic system of azine and its aromatic substituent should block one face of heterocyclic ring plane for nucleophilic attack therefore products of nucleophilic addition will form in a certain stereoselectivity. By means of theoretical calculations it was demonstrated that in the equilibrium between isomers I and II the formation conformer II is preferable (Scheme 56).68
Diastereoselective addition of (trimethylsilyl)ethynylmagnesium bromide to optically active quinolines 133 in the presence of alkyl (or aryl) chloroformates produces compounds 134 and 135 in good yields and moderate stereoselectivity.69 This is the result of steric hindrance created by bulky substituents at position C(4) of the quinolone ring (Scheme 57).
During the synthesis of dynemicin A antibiotic it was demonstrated by the example of quinoline 136 that chiral groups at the position C(4) can lead to the stereoselective formation of addition products (Scheme 58, Table 10). Bulky protective groups R1 for instance phenyl chloroformate (instead of methyl chloroformate) and lower temperature were the most effective factors for improving the stereoselectivity of this reaction.70
An example of the reaction of nucleophilic addition to isoquinoline with a chiral substituent at position C(3) is known.71 Diastereoselective addition of (trimethylsilyl)ethynylmagnesium bromide to isoquinolines 140 in the presence of aryl chloroformates yields compounds 141 and 142 with high diastereoselectivities due to the presence of a chiral substituent at position C(3) of the isoquinoline ring (Scheme 59).
β-Carbolines 143 bearing proline chiral moiety at the position C(9) in the presence of chloroformates are able to react with nucleophiles to form the addition products 144 in high stereoselectivity, the following hydrolysis of 144 affords compounds 145. In these dihydro-β-carbolynes (145) the configuration of an asymmetric center depends on a nature of R1 substituent of adduct 143, moreover the size of this substituent does not affect the reaction stereoselectivity of the process (Schemes 60, 61, Tables 11, 12).47,72,73
An example of the complexation effect on the stereoselective addition of nucleophiles to pyridinium salts is documented.74 Reactions of 1-methyl- (147a) and 1-benzylpyridoxazepinone (147b) salts with alkyl-, alkenyl- and phenyl-magnesium bromide result in the products 1,2 and 1,4-addition 148a,b with high stereoselectivity and in good yields. Stereo- and regioisomers 149-151a,b are found in minor amounts. The process becomes less selective when organolithium compounds are used instead of the Grignard reagents (Scheme 62, Table 13).
In this case, the high regio- and stereoselectivity of the reaction is explained by the coordination of the Grignard reagent to the amide oxygen atom, which leads to the formation of complex A. The transfer of the organic fragment from the magnesium atom to C(4) of the pyridine ring results in the enolate B; its hydrolysis leads to compounds 148-151 (Scheme 63).74
Studies on the conformational behavior of compounds 147a,b reveal that the seven-membered ring is relatively rigid and only two energetically favorable conformations are possible (only one is shown) (Figure 8).74
In this conformation, the amide carbonyl group is located above the pyridinium ring plane in an anti-position relative to the hydrogen atom in the chiral center, while in the second conformer the carbonyl group and the pyridinium ring are coplanar. It is remarkable that the conformations of compounds 137 where the carbonyl amide group is arranged under the pyridinium ring are energetically unfavorable. Therefore, the intermolecular shift of the group R1 of the complex A through a six-membered transition state can lead predominantly to the isomer B (Scheme 63).74
In the total synthesis of antibiotic dynemycin A, alkyne radicals were introduced into a substituted tetrahydrophenanthridine containing chiral centers in the cyclohexane moiety.75 A mixture of diastereomers in the ratio of 9:1 was obtained upon treatment of compound 152 with chloroformate in the presence of the Grignard reagent, (triisopropylsilyl)ethynylmagnesium bromide. Compound 153 was the major isomer, which was formed as a result of cis-addition (relative to the 4- and 7-substituents) of the alkyne fragment to the pyridinium C(2) atom (Scheme 64).75
The starting compound 152 is sterically severely hindered for the attack along the path b; therefore, the addition is stereoselective and proceeds with predominant formation of compound 153 (path a) (Scheme 65).75
Tetrahydrophenanthridines 154a,b react similarly with phenylethynylmagnesium bromide in the presence of methyl chloroformate. It was found76 that the non-protected compound 154a forms predominantly a cis-addition product 155a (relative to the methyl group), the ratio 155b:156b = 11:1, while TBS-protected analogue 154b produces a diastereomer mixture in which trans-addition product 156b is dominant (ratio 155b:156b = 1:1.5) (Scheme 66).76
This fact was explained by a half-chair conformation of the intermediate alkoxide formed from compound 154a in which the magnesium counter ion is chelated by one or two oxygen atoms of the methoxy groups. This leads to pseudo-equatorial orientation of the methyl substituent in compound 155a. As a result, the axial addition of acetylide at the cis-position relative to this Me group is facilitated (Figure 9).76
II. Stereoselective addition of an optically active nucleophile to an achiral azine
In all previously discussed cases, optically active azine substrates reacted with nucleophilic agents. Steric factors caused by the presence of a chiral azine substituent effectively determined the asymmetric character of the synthesis. Another variant of obtaining of optically active azine derivatives where a chiral nucleophile reacts with an achiral amine is rather interesting and much less investigated. In this case, the direction of a nucleophilic attack mostly depends on the structure of the attacking species. It is possible to achieve stereoselective formation of products with the desired configuration by varying the reaction conditions.
Yamaguchi and co-authors77 have performed the enantioselective addition of chiral (R)-3-phenyl-3-trimethylsilylprop-l-ene (157) with N-acylisoquinolinium ions obtained from isoquinolines la,с,d. The reaction leaded to formation of (S)-1-[(E)-3-phenylprop-2-enyl]-1,2- dihydroisoquinolines 158a-с with high enantioselectivities (Scheme 68).
The authors suggest68 that this addition proceeds with formation of an antiperiplanar transition state A, which leads to the (S)-adduct. The transition state B, which is a precursor of the (R)-adduct, is less energetically favorable (Figure 10).
Stereoselective addition of chiral allylsilane 157 to activated quinoline has been described.78 As a result of the reaction with quinolinium salt 159, an optically active 2-substituted 1,2-dihydroquinoline 160 (ее 77%) is formed (Scheme 69).
Several possible reaction pathways have been proposed.78 The most preferential is believed to be that including the formation of intermediate 159A (Scheme 70). Elimination of the trimethylsilyl group yields the product with (S)-configuration of the chiral center.
No reactions of stereoselective addition of chiral C-nucleophiles to triazines have been described yet; however, a reaction with an optically active O-nucleophile, viz., a natural alcohol L-menthol is known. Thus the addition of enantiomerically pure L-menthol at the unsubstituted carbon atom of 3-phenyl-1,2,4-triazin-5(4H)-one (108) activated by acid anhydrides yields a mixture of diastereomers 161 and 162.79 The diastereomer 161 is the major product. It was also established that the diastereoselectivity of the reaction depends on the size of the acylating agent. In the case of acetic anhydride (R = Me), the diastereomer ratio 161:162 is equal to 60:40, while with the more sterically hindered isobutyric anhydride (R = Pri) this ratio increases to 85:15 (Scheme 71). The predominant formation of stereoisomers 161 is explained79 by steric factors.
The addition of chiral alcohols like cholesterol, dihydrocholesterol, borneol and isoborneol to 3-phenyl-1,2,4-triazin-5(4H)-one (108) in the presence of acetic and isobutyric acid anhydrides runs without diastereoselectivity.80
III. Asymmetric induction in the attack of an achiral nucleophile at an achiral azine
Asymmetric induction in a reaction of an achiral nucleophile with an achiral azine substrate is possible only if optically active solvents or catalysts are used (Scheme 72).
Pyridinium salts 163a,b in the presence of chiral ligands 164a-e react with ethyl propiolate diastereoselectively. The addition products 165a,b are useful building blocks for the elaboration of a wide range of polysubstituted piperidines and indolizidines (Scheme 73, Table 14).81
The best enantioselectivity was achieved when Pri2NPrn base and 164e ligand were used. They were used in the synthesis of addition products of some propiolates (Scheme 74). It was shown, that the substituent size of propiolate dramatically exert on stereoselectivity. The highest ee value was recorded using 1-pentyn-3-one (product 165f). With increasing of the chain length of the ynones, the enantioselectivity gradually dropped. The removing of the carbonyl group leads to practically complete loss of ee (compounds 165j-l) (Scheme 74).81
Pyridines, quinolines, and isoquinolines in the presence of ethyl chloroformate participate in copper(I) - catalyzed reactions of nucleophilic addition with alkynes leading to compounds 167 (Schemes 75, 76, Tables 15, 16). This multicomponent reactions running in the presence of CuCl provide a straightforward and regioselective pathway to cyclic propargylcarbamates, widely used as chiral building blocks for synthesis of alkaloids and other biologically relevant molecules.82
As shown in table 15, the use of several commercially available chiral phosphorus- and nitrogen-donor ligands in this reaction unfortunately resulted in low to zero enantioselectivity with phenylacetylene. The best result was achieved with (R)-QUINAP and the authors tried similar PINAP ligands 166a-e. The best of used PINAP ligands in investigated type of reactions turned out ligand 166e.
Shibasaki and co-authors83-86 studied the synthesis of Reissert compounds 170 and 171 derived from quinolines 168 or isoquinolines 169, using bifunctional naphthyl catalysts 172. Authors suggested that enantioselectivity of this reaction depended on the nature of R3 subsequent at acyl chloride. For example, the more electron-rich and therefore less reactive 2-furoyl chloride gave slightly better enantioselectivity than acetyl chloride. The effect of the solvent’s polarity on enantioselectivity is significant. Studding the reaction of quinoline with more reactive benzoyl chloride, it was found that, when the polarity of the solvent was decreased by adding a less polar solvent, the ee increased to 78% from 71%, although the yield of the product became much lower. In contrast, the ee decreased to 37% when the more polar acetonitrile was used as a solvent. If changing the Z fragment of catalyst 161 from P(O)Ph2 to P(O)(o-Tol)2, it can give more reactive Lewis base, which increases both ee and the yield of the product (Scheme 77, Table 17).
Although a detailed reaction mechanism is still not clear at this moment, the reaction should be promoted by the dual activation of catalyst 172 the N-acyl quinolinium or N-acyl isoquinolinium ion and TMSCN by the Lewis acid (Al) and the Lewis base (oxygen atom of the phosphine oxide) moieties of the catalyst 172, respectively. The results using the control catalyst 172 contained diphenylmethyl groups providing only steric bulkiness, leading to decrease of ee. Thus, the reaction of quinolinium with TMSCN in the presence of benzoyl chloride and catalyst 172 (Z = CHPh2), which is not the Lewis base, afforded to 1-benzoyl-1,2-dihydroquinolinium-2-carbonitrile with the configuration (S) predominantly (24% ee in 73% yield).
Authors83-86 postulated the working model for the catalytic cycle (by the example of substituted quinolinium) (Scheme 78). The first step should be the formation of the reactive N-acyl quinolinium intermediate 173 by the reaction of quinoline with the acid chloride. The acyl quinolinium ion should be activated by complexation of the amide oxygen to the Lewis acid (Al atom of chiral catalyst). The two conformers of the amide bond s-trans-174 and s-cis-174 would exist in equilibrium. However, when TMSCN is activated by the Lewis base moiety of the catalyst 172, the reaction via s-trans-174 would be more favorable than via s-cis-174. In the cis-isomer the distance between the activated TMSCN and the electrophilic carbon would be too far for catalysis. The hypothetical transition state s-trans-174 could explain the absolute configuration R of the product (Scheme 78).
Later, the asymmetric bifunctional catalyst 172 was modified, that allow using pyridine derivatives 175a-c for this reaction. Thus the reaction of pyridine 175b with TMSCN, catalyzed by chiral binaphthyl 172 (Z = P(O)Ph2), in the presence of acyl chlorides, leads to the products of cyanide ion addition in positions C(2) and C(6) of pyridine ring in proportion 2.3:1 with low enantioselectivity (ee < 9%). The using of catalyst 176 increased regioselectivity. 2-Cyanosubstituted pyridines 177a-c with (S)-configuration were obtained predominantly (ratio of 2- and 6-products changed depending on substituting group R1 from 12:1 to 50:1) (Scheme 79).86
In 2007 was described the catalytic enantioselective variant of the Petasis transformation of quinolines. On the base of mechanism of Petasis reaction authors created newly designed catalysts that have a chelating functionality, which could activate the boronic acids and direct the stereochemical outcome, as shown for A on the Scheme 80.87
For the stereoselective addition of alkynes to quinolines catalysts 178 based on thiourea are useful. In the concept of the authors thiourea moiety could activate N-acylated quinolinium salts as a Brønsted acid. Authors firstly probe the utility of new functionalized catalysts in the reactions of quinoline with vinylboronic acid 181A (Scheme 81, Table 18).87
Catalyst 178b gives better enantioselectivity and was used for the synthesis of compounds 182bA-182aF (Scheme 82, Table 19).87
Enantioselective addition of organolithium reagents to the isoquinoline in soft conditions in the presence of chiral ligand (−)-sparteine (185) is described.88 The reaction results in the mixture of chiral dihidroisoquinolines 183, 184 (for R = Me, Bun), or only product 183 (R = Ph) (Scheme 83).
Hereafter, authors89,90 studied in detail the reaction of azines with organolithium reagents, using unsubstituted quinoline (7) as substrate. They varied the conditions (temperature, solvent) and used the wide range of chiral catalysts, such as sparteine (185), bisoxazolines 186, chiral diether 187, and also symmetric (188) and nonsymmetric (189) 1,2-diamines.
The addition of methyl formate to reaction mixture leads to dihydroisoquinolines 190 or 191, moreover the configuration of the asymmetrical center depends on the type of chiral catalyst and the nature of R radical (Scheme 85, Table 20).
In conclusion, these different studies have shown that (R,R)-dimethoxybiphenylethane (187) as chiral catalyst was not effective: dihydroquinolines 190 were obtained with moderate yields, but the enantioselectivity was low (ee 2-26%). Catalysis with (-)-sparteine (185) gave dihydroquinolines 191, having the opposite chiral center’s configuration, herewith the enantioselectivity of the process raised (especially for the reaction with phenyllithium), and the yield of the product was about 99% for R = Bun. Bisoxazolines as chiral catalysts allow to get best selectivity for the reaction of quinoline with methyllithium and catalyst 186a (ee 63%), resulting in (S)-configuration of dihydroquinolines.89,90
N,N`-Tetramethylcyclohexane-1,2-diamine 188a (TMCDA) was the best ligand for the addition of 1-naphthyllithium since the yield of dihydroquinoline 191d (R = 1-naphtyl) was 75% (ee 64%). Replacement of methyl substituents at N-atom of catalyst 188a to more bulky alkyl groups (compounds 188b-d) led to the decreasing of yields and diastereoselectivity of reaction (Scheme 1).89,90
The experiments on of N,N’-tetramethylethane-1,2-diamines 189a-c as chiral catalyst have brought authors to some findings. The catalysis with diamine 189a led to almost racemic product. The reaction with diamine 189c, which has axis of symmetry С2, resulted in the addition products with medium enantioselectivity. And, finally, the catalyst 189b operates as diamine with pseudo-C2-symmetry, because the results with this catalyst are similar to those obtained with the diamine 189c.89,90
CONCLUSION
Comparable analysis of various types of chirality induction in nucleophilic addition reactions in heterocycles indicates that the most common way for the chirality generation based on the introduction of chiral auxiliary groups into azines, the less popular way is the using of various types of chiral catalysts. Possibility for the generation of chirality by the asymmetric center of nucleophile is used very seldom.
The advantage of the method of introduction of chiral auxiliary groups is its generality. Introduction of the same group into various azines is commonly results in generation of asymmetric induction not depending on the type of used nucleophile. The drawback of this method is in difficulty of removing of the auxiliary group afterwards.
The obvious advantage for chiral catalysts methods is in less reaction steps for obtaining the target compound. Moreover as it can be followed in current review in most cases the proper chiral catalyst can be selected only after the screening.
Chiral nucleophiles are extremely short of use in diastereoselective nucleophilic additions, most probably due to their low availability. Therefore investigators prefer to introduce the chirality elements into heterocycles by using another synthetic ways.
References
1. (a) O. N. Chupakhin, V. N. Charushin, and H. C. van der Plas, Nucleophilic Aromatic Substitution of Hydrogen, Academic Press, San-Diego, 1994, P. 367; (b) V. N. Charushin and O. N. Chupakhin, Mendeleev Commun., 2007, 17, 249. CrossRef
2. (a) D. L. Comins and S. P. Joseph, Advances in Nitrogen Heterocycles, Vol. 2, ed. by C. J. Moody, JAI Press Inc., Greenwich, CT, 1996, pp. 251–294; CrossRef (b) D. L. Comins and S. P. Joseph, ed. by A. McKillop, Comprehensive Heterocyclic Chemistry, 2nd ed., Pergamon Press, Oxford, England, 1996, Vol. 5, pp. 37–89; (c) D. L. Comins, J. Heterocycl. Chem., 1999, 36, 1491; CrossRef (d) D. L. Comins, J. T. Kuethe, T. M. Miller, F. C. Février, and C. A. Brooks, J. Org. Chem., 2005, 70, 5221; CrossRef (e) K. W. Bentley, Nat. Prod. Rep., 2005, 22, 249; CrossRef (f) M. Somei and F. Yamada, Nat. Prod. Rep., 2005, 22, 73; CrossRef (g) J. P. Michael, Nat. Prod. Rep., 2004, 21, 625; CrossRef (h) J. P. Michael, Nat. Prod. Rep., 2004, 21, 650; CrossRef (i) J. P. Michael, Nat. Prod. Rep., 2008, 25, 166. CrossRef
3. I. N. Egorov, G. V. Zyryanov, V. L. Rusinov, and O. N. Chupakhin, Russ. Chem. Rev., 2005, 74, 1073. CrossRef
4. J. A. Bull, J. J. Mousseau, G. Pelletier, and A. B. Charette, Chem. Rev., 2012, 112, 2642. CrossRef
5. M. Ahamed and M. H. Todd, Eur. J. Org. Chem., 2010, 5935. CrossRef
6. O. Sieck, S. Schaller, S. Grimme, and J. Liebscher, Synlett, 2003, 337. CrossRef
7. M. Pauvert, S. C. Collet, M.-J. Bertrand, A. Y. Guingant, and M. Evain, Tetrahedron Lett., 2005, 46, 2983. CrossRef
8. (a) H. W. Gibson, M. A. G. Berg, J. C. Dickson, P. R. Lecavalier, H. Wang, and J. S. Merola, J. Org. Chem., 2007, 72, 5759; CrossRef (b) C. Fuchs, C. Bender, B. Ziemer, and J. Liebscher, J. Heterocycl. Chem., 2008, 45, 1651. CrossRef
9. J. Bräckow, J. Pabel, P. Mayer, K. Polborn, and K. T. Wanner, Tetrahedron, 2010, 66, 7279. CrossRef
10. C. Bender and J. Liebscher, ARKIVOC, 2009, vi, 111.
11. Y. Genisson, C. Marazano, and B. C. Das, J. Org. Chem., 1993, 58, 2052. CrossRef
12. D. Barbier, C. Marazano, C. Riche, B. C. Das, and P. Potier, J. Org. Chem., 1998, 63, 1767. CrossRef
13. B. Guilloteau-Bertin, D. Compère, L. Gil, C. Marazano, and B. C. Das, Eur. J. Org. Chem., 2000, 1391. CrossRef
14. C. Legault and A. B. Charette, J. Am. Chem. Soc., 2003, 125, 6360. CrossRef
15. G. B. Richter-Addo, D. A. Knight, M. A. Dewey, A. M. Arif, and J. A. Gladysz, J. Am. Chem. Soc., 1993, 115, 11863. CrossRef
16. A. B. Charette, M. Grenon, A. Lemire, M. Pourashraf, and J. Martel, J. Am. Chem. Soc., 2001, 123, 11829. CrossRef
17. A. Lemire and A. B. Charette, J. Org. Chem., 2010, 75, 2077. CrossRef
18. F. Stazi, D. Marcoux, J.-C. Poupon, D. Latassa, and A. B. Charette, Angew. Chem. Int. Ed., 2007, 46, 5011. CrossRef
19. T. Focken and A. B. Charette, Org. Lett., 2006, 8, 2985. CrossRef
20. (a) J. Streith, A. Boiron, T. Sifferlen, C. Strehler, and T. Tschamber, Tetrahedron Lett., 1994, 35, 3927; CrossRef (b) J. Streith, A. Boiron, J.-L. Paillaud, E.-M. Rodriguez-Perez, C. Strehler, T. Tschamber, and M. Zehnde, Helv. Chim. Acta, 1995, 78, 61. CrossRef
21. D. L. Comins, R. R. Goehring, S. P. Joseph, and S. O’Connor, J. Org. Chem., 1990, 55, 2574. CrossRef
22. D. L. Comins and A. Dehghani, J. Chem. Soc., Chem. Commun., 1993, 1838. CrossRef
23. D. L. Comins, S. P. Joseph, and R. R. Goehring, J. Am. Chem. Soc., 1994, 116, 4719. CrossRef
24. D. L. Comins and L. Guerra-Weltzien, Tetrahedron Lett., 1996, 37, 3807. CrossRef
25. D. L. Comins, Y. Zhang, and X. Zheng, Chem. Commun., 1998, 22, 2509. CrossRef
26. M. M. Martínez, L. A. Sarandeses, and J. P. Sestelo, Tetrahedron Lett., 2007, 48, 8536. CrossRef
27. P. Singh, D. L. Comins, and S. P. Joseph, Acta Crystallogr., 1994, C50, 25.
28. G. B. Jones, Tetrahedron, 2001, 57, 7999. CrossRef
29. D. L. Comins, Y. Zhang, and S. P. Joseph, Org. Lett., 1999, 1, 657. CrossRef
30. D. L. Comins, C. A. Brooks, R. S. Al-awar, and R. R. Goehring, Org. Lett., 1999, 1, 229. CrossRef
31. D. B. Gotchev and D. L. Comins, J. Org. Chem., 2006, 71, 9393. CrossRef
32. D. L. Comins, D. H. LaMunyon, and X. Chen, J. Org. Chem., 1997, 62, 8182. CrossRef
33. D. L. Comins and A. Dehghani, J. Org. Chem., 1995, 60, 794. CrossRef
34. D. L. Comins, X. Chen, and L. A. Morgan, J. Org. Chem., 1997, 62, 7435. CrossRef
35. B. H. Wolfe, A. H. Libby, R. S. Al-awar, C. J. Foti, and D. L. Comins, J. Org. Chem., 2010, 75, 8564. CrossRef
36. J. T. Kuethe and D. L. Comins, J. Org. Chem., 2004, 69, 2863. CrossRef
37. D. L. Comins, D. A. Stolze, P. Thakker, and C. L. McArdle, Tetrahedron Lett., 1998, 39, 5693. CrossRef
38. D. L. Comins, S. Huang, C. L. McArdle, and C. L. Ingalls, Org. Lett., 2001, 3, 469. CrossRef
39. C. E. Hoesl, J. Pabel, K. Polborn, and K. T. Wanner, Heterocycles, 2002, 58, 383. CrossRef
40. C. E. Hoesl, M. Maurus, J. Pabel, K. Polborn, and K. T. Wanner, Tetrahedron, 2002, 58, 6757. CrossRef
41. J. Bräckow, J. Pabel, P. Mayer, K. Polborn, and K. T. Wanner, Tetrahedron, 2010, 66, 7279. CrossRef
42. D. L. Comins, J. T. Kuethe, H. Hong, and F. J. Lakner, J. Am. Chem. Soc., 1999, 121, 2651. CrossRef
43. D. L. Comins and J. J. Sahn, Org. Lett., 2005, 7, 5227. CrossRef
44. S. Huang and D. L. Comins, Chem. Commun., 2000, 569. CrossRef
45. J. T. Kuethe and D. L. Comins, Org. Lett., 2000, 2, 855. CrossRef
46. J. T. Kuethe and D. L. Comins, J. Org. Chem., 2004, 69, 5219. CrossRef
47. T. Itoh, Y. Matsuya, Y. Enomoto, and A. Ohsawa, Tetrahedron, 2001, 57, 7277. CrossRef
48. (a) D. L. Comins and M. O. Killpack, J. Am. Chem. Soc., 1992, 114, 10972; CrossRef (b) D. L. Comins, M. O. Killpack, E. Despagnet, and E. Zeller, Heterocycles, 2002, 58, 505. CrossRef
49. O. Surygina, M. Ehwald, and J. Liebscher, Tetrahedron Lett., 2000, 41, 5479. CrossRef
50. O. Sieck, M. Ehwald, and J. Liebscher, Eur. J. Org. Chem., 2005, 663. CrossRef
51. P. Hermange, M. E. T. H. Dau, P. Retailleau, and R. H. Dodd, Org. Lett., 2009, 11, 4044. CrossRef
52. I. N. Egorov, G. V. Zyryanov, E. N. Ulomsky, V. L. Rusinov, and O. N. Chupakhin, Tetrahedron Lett., 2006, 47, 7485. CrossRef
53. O. N. Chupakhin, I. N. Egorov, V. L. Rusinov, and P. A. Slepukhin, Russ. Chem. Bull., 2010, 59, 991. CrossRef
54. I. N. Egorov, O. N. Chupakhin, M. V. Berezin, G. L. Rusinov, V. L. Rusinov, E. V. Buravlev, I. Y. Chukicheva, and A. V. Kuchin, Russ. Chem. Bull., 2011, 60, 914. CrossRef
55. I. N. Egorov, B. König, V. L. Rusinov, and O. N. Chupakhin, Mendeleev Commun., 2008, 18, 99. CrossRef
56. A. I. Meyers, N. R. Natale, D. G. Wettlaufer, S. Rafii, and J. Clardy, Tetrahedron Lett., 1981, 22, 5123. CrossRef
57. A. I. Meyers and T. Oppenlaender, J. Am. Chem. Soc., 1986, 108, 1989. CrossRef
58. A. I. Meyers and T. Oppenlaender, J. Chem. Soc., Chem. Commun., 1986, 920. CrossRef
59. (a) A. I. Meyers and D. G. Wettlaufer, J. Am. Chem. Soc., 1984, 106, 1135; CrossRef (b) A. I. Meyers, J. Org. Chem., 2005, 70, 6137. CrossRef
60. M. Amat, M. D. Coll, and J. Bosch, Tetrahedron, 1995, 51, 10759. CrossRef
61. M.-L. Bennasar, E. Zulaica, Y. Alonso, and J. Bosch, Tetrahedron: Asymmetry, 2003, 14, 469. CrossRef
62. D. L. Comins, L. S. King, E. D. Smith, and F. C. Février, Org. Lett., 2005, 7, 5059. CrossRef
63. R. Gosmini, P. Mangeney, A. Alexakis, M. Commerçon, and J.-F. Normant, Synlett, 1991, 111. CrossRef
64. (a) P. Mangeney, R. Gosmini, and A. Alexakis, Tetrahedron Lett., 1991, 32, 3981; CrossRef (b) A. Alexakis, P. Mangeney, N. Lensen, J.-P. Tranchier, R. Gosmini, and S. Raussou, Pure Appl. Chem., 1996, 68, 531. CrossRef
65. P. Mangeney, R. Gosmini, S. Raussou, M. Commerçon, and A. Alexakis, J. Org. Chem., 1994, 59, 1877. CrossRef
66. S. Raussou, R. Gosmini, P. Mangeney, A. Alexakis, and M. Commerçon, Tetrahedron Lett., 1994, 35, 5433. CrossRef
67. (a) S. Raussou, N. Urbain, P. Mangeney, A. Alexakis, and N. Platzer, N., Tetrahedron Lett., 1996, 37, 1599; CrossRef (b) P. Mangeney, L. Hamon, S. Raussou, N. Urbain, and A. Alexakis, Tetrahedron, 1998, 54, 10349. CrossRef
68. S. Yamada and M. Inoue, Org. Lett., 2007, 9, 147. CrossRef
69. (a) G. Guanti, S. Perrozzi, and R. Riva, Tetrahedron: Asymmetry, 1998, 9, 3923; CrossRef (b) G. Guanti, S. Perrozzi, and R. Riva, Tetrahedron: Asymmetry, 2002, 13, 2703; CrossRef (c) L. Banfi, A. Basso, V. Gandolfo, G. Guanti, and R. Riva, Tetrahedron Lett., 2004, 45, 4221. CrossRef
70. T. Nishikawa, M. Yoshikai, K. Obi, T. Kawai, R. Unno, T. Jomori, and M. Isobe, Tetrahedron, 1995, 51, 9339. CrossRef
71. G. Guanti and R. Riva, Tetrahedron: Asymmetry, 2001, 12, 1185. CrossRef
72. Y. Matsuya, T. Itoh, Y. Enomoto, and A. Ohsawa, Heterocycles, 2000, 53, 2357. CrossRef
73. T. Itoh, M. Miyazaki, S. Ikeda, K. Nagata, M. Yokoya, Y. Matsuya, Y. Enomoto, and A. Ohsawa, Tetrahedron, 2003, 59, 3527. CrossRef
74. A. G. Schultz, L. Flood, and J. P. Springer, J. Org. Chem., 1986, 51, 838. CrossRef
75. M. D. Shair, T. Y. Yoon, K. K. Mosny, T. C. Chou, and S. J. Danishefsky, J. Am. Chem. Soc., 1996, 118, 9509. CrossRef
76. A. G. Myers, N. J. Tom, M. E. Fraley, S. B. Cohen, and D. J. Madar, J. Am. Chem. Soc., 1997, 119, 6072. CrossRef
77. R. Yamaguchi, M. Tanaka, T. Matsuda, and K. Fujita, Chem. Comm., 1999, 2213. CrossRef
78. R. Yamaguchi, M. Tanaka, T. Matsuda, T. Okano, T. Nagura, and K. Fujita, Tetrahedron Lett., 2002, 43, 8871. CrossRef
79. (a) O. N. Chupakhin, G. V. Zyryanov, V. L. Rusinov, V. P. Krasnov, G. L. Levit, M. A. Korolyova, and M. I. Kodess, Tetrahedron Lett., 2001, 42, 2393; CrossRef (b) G. V. Zyryanov, V. L. Rusinov, O. N. Chupakhin, V. P. Krasnov, G. V. Levit, M. I. Kodess, and T. S. Shtukina, Russ. Chem. Bull., 2004, 53, 1290. CrossRef
80. I. N. Egorov, G. V. Zyryanov, P. A. Slepukhin, T. A. Tseitler, V. L. Rusinov, and O. N. Chupakhin, Chem. Het. Comp., 2012, 48, 625.
81. Z. Sun, S. Yu, Z. Ding, and D. Ma, J. Am. Chem. Soc., 2007, 129, 9300. CrossRef
82. D. A. Black, R. E. Beveridge, and B. A. Arndtsen, J. Org. Chem., 2008, 73, 1906. CrossRef
83. M. Takamura, K. Funabashi, M. Kanai, and M. Shibasaki, J. Am. Chem. Soc., 2000, 122, 6327. CrossRef
84. K. Funabashi, H. Ratni, M. Kanai, and M. Shibasaki, J. Am. Chem. Soc., 2001, 123, 10784. CrossRef
85. M. Takamura, K. Funabashi, M. Kanai, and M. Shibasaki, J. Am. Chem. Soc., 123, 6801. CrossRef
86. E. Ichikawa, M. Suzuki, K. Yabu, M. Albert, M. Kanai, and M. Shibasaki, J. Am. Chem. Soc., 2004, 126, 11808. CrossRef
87. Y. Yamaoka, H. Miyabe, and Y. Takemoto, J. Am. Chem. Soc., 2007, 129, 6686. CrossRef
88. A. Alexakis and F. Amiot, Tetrahedron: Asymmetry, 2002, 13, 2117. CrossRef
89. F. Amiot, L. Cointeaux, E. J. Silve, and A. Alexakis, Tetrahedron, 2004, 60, 8221. CrossRef
90. L. Cointeaux and A. Alexakis, Tetrahedron: Asymmetry, 2005, 16, 925 CrossRef