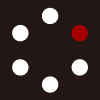
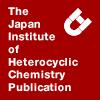
HETEROCYCLES
An International Journal for Reviews and Communications in Heterocyclic ChemistryWeb Edition ISSN: 1881-0942
Published online by The Japan Institute of Heterocyclic Chemistry
e-Journal
Full Text HTML
Received, 28th June, 2012, Accepted, 13th August, 2012, Published online, 20th August, 2012.
DOI: 10.3987/COM-12-S(N)68
■ A 2-AMINO-6-METHYLPYRIDIN-5-YL NUCLEOBASE FOR GC BASE PAIR RECOGNITION IN THE PARALLEL TRIPLEX DNA
Motoi Nakahara, Yoshiyuki Hari,* and Satoshi Obika
Graduate School of Pharmaceutical Science, Osaka University, 1-6 Yamadaoka, Suita, Osaka 560-0871, Japan
Abstract
DNA triple helices containing consecutive C+H•GC triplets are unstable at physiological pH because protonations of cytosines into the third strand are necessary. Previously, a 2-aminopyridin-5-yl nucleobase (P) was developed and has been widely used as a substitute for the cytosine nucleobase. In this work, we designed a 2-amino-6-methylpyridin-5-yl nucleobase (PMe), which could preferentially adopt an anti-orientation by the 6-methyl group. This might lead to formation of a stable base triplet with a GC base pair compared to P. We also synthesized 15-mer triplex-forming oligonucleotides (TFOs) containing PMe by the standard solid-phase method and evaluated the triplex stability of the TFOs at physiological pH by UV melting experiments.INTRODUCTION
Sequence specific binding of triplex-forming oligonucleotides (TFOs) to double-stranded DNA (dsDNA) can selectively regulate gene expression and can be applied to genetic diagnostics.1 Pyrimidine-rich TFOs bind to the major groove of dsDNA with homopurine tracts by Hoogsteen hydrogen bonding. A thymine (T) and a protonated cytosine (C+H) in the third strand recognize an adenine (A) of an AT base pair (T•AT) and a guanine (G) of a GC base pair (C+H•GC), respectively. However, the C residues in TFOs prevent stable triple helix formation at neutral pH. In particular, when TFOs containing contiguous cytosine residues are used, formation of triplex with target DNA is quite difficult. The development of non-natural nucleobases which form a stable triplet with a GC base pair in triplex DNA has been attempted to overcome this problem.2,3 For example, replacement of cytosine by 5-methylcytosine in TFOs leads to an increase in the stability of triple helix formation.2 However, in the case of target dsDNA containing contiguous GC base pairs, even TFO using 5-methylcytosine can show inadequate stability for triple helix formation with the dsDNA.4 In 1996, Leumann’s group and Niedle’s group independently reported that 2-aminopyridin-5-yl nucleobases (P4,5 and MeP4) as cytosine analogues were more basic than cytosine, and these nucleobases were useful for GC recognition of target dsDNA6 and 15-mer TFO bearing four contiguous MeP residues could form a stable triple helix at neutral conditions (Figure 1).
The glycosidic bonds of P and MeP can freely rotate. If the rotation was restricted to the anti-orientation, appropriate for recognition of a GC base pair, an increase of the affinity to a GC base pair was considered (Figure 2).7 Thus, we designed a 2-amino-6-methylpyridin-5-yl nucleobase (PMe) to restrict the glycosidic bond in the anti-orientation by steric repulsion between the 6-methyl group and the furanose ring. In this paper, we describe the synthesis of oligonucleotides containing PMe and P, as well as evaluation of their binding affinity to dsDNA at neutral pH.
RESULTS AND DISCUSSION
The syntheses of C-nucleosides with PMe and P are shown in Scheme 1. According to a previous report by Leumann’s group, relatively hard conditions, i.e., 40% aqueous methylamine (70 °C, 26 h) was required to efficiently deprotect oligonucleotides containing the benzoyl-protected P.4 Thus, we decided to protect the exocyclic amino function in PMe and P using the phenoxyacetyl (Pac) group which could be removed under mild and/or standard conditions. C-Nucleosides 1a and 1b were synthesized as previously reported by McLaughlin’s group.8,9 After protection of the 3’- and 5’-hydroxyl groups of 1a using TBDMS groups (2a; 82%), the protection of amino group was performed with PacCl (3a; 98%) and desilylation of 3a with tetra-n-butylammonium fluoride (TBAF) gave the desired C-nucleoside 4a in 89% yield. As we expected, it was clarified that the nucleobase moiety of 4a preferentially exists in anti-orientation by the NOE correlation between H5’ in the sugar moiety and H4, not 6-methyl group, in the nucleobase (Figure 3). The phosphoramidite building block 6a was obtained by dimethoxytritylation of 4a with 4,4’-dimethoxytrityl chloride (5a; 94%) followed by phosphitylation of 5a with 2-cyanoethyl N,N-diisopropylchlorophosphoramidite (6a; 83%). The phosphoramidite 6b was synthesized in the same way. Using 6a and 6b, the 15-mer TFO-1–TFO-8 oligonucleotides listed in Figure 4 were synthesized under standard conditions on an automated DNA synthesizer.
The dsDNA binding properties of the synthesized TFOs were examined under neutral conditions by means of thermal denaturation experiments. We used hairpin-loop dsDNA linked to a hexa(ethyleneglycol) unit (C18-spacer) as target dsDNA. Because the hairpin-loop structure increased the thermal stability of the duplex, it could prevent the transition of dsDNA to ssDNA from overlapping with that of triplex to duplex. The results obtained by UV melting experiments are summarized in Table 1. The Tm value of the triplex formed between the complementary dsDNA and TFO-1 containing single PMe modification was 41 °C, comparable to that of TFO-2 (X = P) and 4 (X = C) and slightly higher than that of TFO-3 (X = C). On the other hand, in the case of TFO-5 bearing three contiguous PMe modifications, the stability (Tm = 37 °C) was significantly decreased compared with that (Tm = 42 °C) of the P-modified TFO-6, though TFO-5 showed a similar Tm value to the C-modified TFO-8. The UV-melting curves using TFOs containing PMe or P are shown in Figure 5. The hyperchromicities of triplex by singly modified TFO-1 and TFO-2 were almost same. However, in the case of three contiguous modifications (TFO-5 and TFO-6), the hyperchromicities significantly decreased and TFO-5 clearly showed smaller hyperchromicity than TFO-6. This suggests that PMe+H•GC triplet may not have enough stacking interaction with adjacent triplets due to the steric bulkiness of the methyl group of PMe. Eventually, this may cause a decrease in stability of triplex with contiguous PMe modifications compared to that with P.
In conclusion, we synthesized TFOs containing PMe and P and evaluated their triplex-forming abilities. We found that the affinity of PMe to a GC base pair was the same degree as that of 5-methylcytosine, but lower than that of P. Moreover, it was suggested that the 6-methyl group of PMe might disturb enough stacking interaction in triplex DNA.
EXPERIMENTAL
All moisture-sensitive reactions were carried out in well-dried glassware under N2 atmosphere. 1H, 13C, and 31P spectra were recorded on JEOL JNM-EX300 or JEOL JNM-EX400 spectrometers. Chemical shifts are reported in parts per million referenced to tetramethylsilane (δ = 0.00 ppm) for 1H NMR spectra, CDCl3 (δ = 77.0 ppm) and CD3OD (δ = 49.0 ppm) for 13C NMR spectra, and phosphoric acid (δ = 0.00 ppm) for 31P NMR spectra. IR spectra were recorded on a JASCO FT/IR-4200 spectrometer. Optical rotations were recorded on a JASCO P-2200 polarimeter. FAB mass spectra were measured on JEOL JMS-600 or JEOL JMS-700 mass spectrometers. MALDI-TOF mass spectra were recorded on a Bruker Daltonics Autoflex II TOF/TOF mass spectrometer. Fuji Silysia silica gel PSQ-60B (0.060 mm) and FL-60D (0.060 mm) were used for flash column chromatography. For HPLC, SHIMADZU LC-10ATVP, SHIMADZU SPD-10AVP and SHIMADZU CTO-10VP instruments were used.
2-Amino-5-[3’,5’-di-O-tert-butyldimethylsilyl-(2’-deoxy-D-ribofuranosyl)]-6-methylpyridine (2a)
Under an N2 atmosphere, imidazole (62 mg, 0.914 mmol) and TBDMSCl (66 mg, 0.439 mmol) were added to a solution of 1a (41 mg, 0.183 mmol) in anhydrous DMF (3 mL) at 0 °C and the mixture was stirred at room temperature for 11 h. After the addition of water at 0 °C, the reaction mixture was diluted with Et2O, washed with water and brine, dried over Na2SO4, and concentrated. The crude product was purified by flash column chromatography (2:1 Hexane-EtOAc) to give 2a (68 mg, 82%); Yellow oil; [α]D28 34.6 (c 1.03, CHCl3); IR νmax (KBr) 1610, 1545, 1485, 1389, 1275 cm-1; 1H-NMR (CDCl3, 400 MHz) δ: 0.08 (6H, s), 0.09 (6H, s), 0.91 (9H, s), 0.91 (9H, s), 1.75 (1H, ddd, J = 5.5, 10.5, 12.5 Hz), 2.08 (1H, ddd, J = 2.0, 5.5, 12.5 Hz), 2.37 (3H, s), 3.65 (1H, dd, J = 5.5, 11.0 Hz), 3.76 (1H, dd, J = 4.0, 11.0 Hz), 3.91 (1H, ddd, J = 2.0, 4.0, 5.5 Hz), 4.33 (2H, brs), 4.41 (1H, ddd, J = 2.0, 2.0, 5.5 Hz), 5.24 (1H, dd, J = 5.5, 10.5 Hz), 6.34 (1H, d, J = 8.5 Hz), 7.59 (1H, d, J = 8.5 Hz); 13C-NMR (CDCl3, 101 MHz) δ: -5.5, -5.4, -4.7, -4.6, 18.0, 18.3, 21.5, 25.8, 25.9, 42.9, 63.6, 74.2, 76.1, 87.5, 106.1, 125.4, 135.8, 153.1, 156.7; MS (FAB) m/z 453 [M+H]+; HRMS (FAB) m/z Calcd for C23H45N2O3Si2 [M+H]+: 453.2963. Found 453.2967.
2-Amino-5-[3’,5’-di-O-tert-butyldimethylsilyl-(2’-deoxy-D-ribofuranosyl)]pyridine (2b)
Under an N2 atmosphere, imidazole (210 mg, 3.08 mmol) and TBDMSCl (215 mg, 1.43 mmol) were added to a solution of 1b (125 mg, 0.595 mmol) in anhydrous DMF (6 mL) at 0 °C and the mixture was stirred at room temperature for 13 h. After the addition of water at 0 °C, the reaction mixture was diluted with Et2O, washed with water and brine, dried over Na2SO4, and concentrated. The crude product was purified by flash column chromatography (1:1 Hexane-EtOAc) to give 2b (208 mg, 80%); Yellow oil; [α]D26 15.4 (c 1.02, CHCl3); IR νmax (KBr) 2954, 2929, 2886, 2857, 1622, 1503, 1471, 1360, 1255, 1089, 1031 cm-1; 1H-NMR (CDCl3, 400 MHz) δ: 0.07 (6H, s), 0.09 (6H, s), 0.91 (9H, s), 0.91 (9H, s), 1.90 (1H, ddd, J = 5.5, 10.5, 12.5 Hz), 2.03 (1H, ddd, J = 1.5, 5.0, 12.5 Hz), 3.59 (1H, dd, J = 6.0, 10.5 Hz), 3.74 (1H, dd, J = 4.0, 10.5 Hz), 3.92 (1H, ddd, J = 2.0, 4.0, 6.0 Hz), 4.39 (2H, brs), 4.42 (1H, ddd, J = 1.5, 2.0, 5.5 Hz), 5.03 (1H, dd, J = 5.0, 10.5 Hz), 6.48 (1H, d, J = 9.0 Hz), 7.49 (1H, dd, J = 2.0, 9.0 Hz), 8.03 (1H, d, J = 2.0 Hz); 13C-NMR (CDCl3, 101 MHz) δ: -5.5, -5.4, -4.7, -4.7, 18.0, 18.4, 25.8, 25.9, 43.7, 63.9, 74.5, 77.9, 87.9, 108.4, 127.3, 136.3, 146.4, 158.0; MS (EI) m/z 438 (M+, 100); HRMS (EI) m/z Calcd for C22H42N2O3Si2: 438.2734. Found 438.2732; Anal. Calcd for C22H42N2O3Si2: C, 60.22; H, 9.65; N, 6.38. Found: C, 60.13; H, 9.82; N, 6.21.
5-[3’,5’-Di-O-tert-butyldimethylsilyl-(2’-deoxy-D-ribofuranosyl)]-6-methyl-2-phenoxyacetylaminopyridine (3a)
Under an N2 atmosphere, PacCl (46 µL, 0.331 mmol) was added to a solution of 2a (125 mg, 0.276 mmol) in anhydrous pyridine (4 mL) at 0 °C and the mixture was stirred at room temperature for 10 h. After the addition of water at 0 °C, the reaction mixture was diluted with EtOAc, washed with water, saturated aqueous NaHCO3 and brine, dried over Na2SO4, and concentrated. The crude product was purified by flash column chromatography (15:1 Hexane-EtOAc) to give 3a (159 mg, 98%); Colorless oil; [α]D27 14.9 (c 1.02, CHCl3); IR νmax (KBr) 2952, 2930, 1702, 1591, 1523, 1496, 1461, 1393, 1361, 1252, 1084, 1034 cm-1; 1H-NMR (CDCl3, 400 MHz) δ: 0.09 (6H, s), 0.10 (6H, s), 0.91 (9H, s), 0.92 (9H, s), 1.76 (1H, ddd, J = 6.0, 10.0, 12.5 Hz), 2.15 (1H, ddd, J = 2.0, 5.5, 12.5 Hz), 2.45 (3H, s), 3.68 (1H, dd, J = 5.5, 10.5 Hz), 3.79 (1H, dd, J = 3.5, 10.5 Hz), 3.95 (1H, ddd, J = 2.0, 3.5, 5.5 Hz), 4.43 (1H, ddd, J = 2.0, 2.0, 6.0 Hz), 4.61(2H, s), 5.28 (1H, dd, J = 5.5, 10.0 Hz), 7.01 (2H, d, J = 8.5 Hz), 7.05 (1H, t, J = 8.5 Hz), 7.34 (2H, t, J = 8.5 Hz), 7.89 (1H, d, J = 8.5 Hz), 8.06 (1H, d, J = 8.5 Hz), 8.81 (1H, brs); 13C-NMR (CDCl3, 101 MHz) δ: -5.5, -5.4, -4.7, -4.7, 18.0, 18.3, 21.5, 25.8, 25.9, 42.9, 63.5, 67.5, 74.1, 76.0, 87.8, 111.6, 114.9, 122.3, 129.8, 132.6, 135.9, 148.4, 153.3, 157.0, 166.7; MS (EI) m/z 586 (M+, 40); HRMS (EI) m/z Calcd for C31H50N2O5Si2: 586.3258. Found 586.3265; Anal. Calcd for C31H50N2O5Si2: C, 63.44; H, 8.59; N, 4.77. Found: C, 63.04; H, 8.74; N, 4.58.
5-[3’,5’-Di-O-tert-butyldimethylsilyl-(2’-deoxy-D-ribofuranosyl)]-2-phenoxyacetylaminopyridine (3b)
Under an N2 atmosphere, PacCl (15 µL, 0.109 mmol) was added to a solution of 2b (32 mg, 0.0729 mmol) in anhydrous pyridine (2 mL) at 0 °C and the mixture was stirred at room temperature for 10 h. After the addition of water at 0 °C, the reaction mixture was diluted with EtOAc, washed with water, saturated aqueous NaHCO3 and brine, dried over Na2SO4, and concentrated. The crude product was purified by flash column chromatography (8:1 Hexane-EtOAc) to give 3b (41 mg, 98%); Colorless oil; [α]D28 9.52 (c 1.05, CHCl3); IR νmax (KBr) 2953, 2929, 2857, 1704, 1521, 1496, 1253, 1093, 1032 cm-1; 1H-NMR (CDCl3, 400 MHz) δ : 0.08 (6H, s), 0.10 (6H, s), 0.91 (9H, s), 0.92 (9H, s), 1.89 (1H, ddd, J = 5.5, 10.5, 12.5 Hz), 2.12 (1H, ddd, J = 1.5, 5.0 12.5 Hz), 3.63 (1H, dd, J = 5.5, 11.0 Hz), 3.76 (1H, dd, J = 4.0, 11.0 Hz), 3.97 (1H, ddd, J = 2.0, 4.0, 5.5 Hz), 4.44 (1H, ddd, J = 1.5, 2.0, 5.5 Hz), 4.63(2H, s), 5.14 (1H, dd, J = 5.0, 10.5 Hz), 7.01 (2H, d, J = 7.5 Hz), 7.05 (1H, t, J = 7.5 Hz), 7.34 (2H, t, J = 7.5 Hz), 7.77 (1H, dd, J = 2.0, 9.0 Hz), 8.24 (1H, d, J = 9.0 Hz), 8.29 (1H, d, J = 2.0 Hz), 8.93 (1H, brs); 13C-NMR (CDCl3, 101 MHz) δ : -5.5, -5.3, -4.7, -4.6, 18.0, 18.4, 25.8, 25.9, 44.2, 63.8, 67.4, 74.4, 77.6, 88.3, 113.8, 114.9, 122.4, 129.9, 134.4, 136.5, 146.0, 149.8, 156.9, 166.8; MS (FAB) m/z 573 [M+H]+; HRMS (FAB) m/z Calcd for C30H49N2O5Si2 [M+H]+: 573.3175. Found 573.3184; Anal. Calcd for C30H48N2O5Si2: C, 62.90; H, 8.45; N, 4.89. Found: C, 62.52; H, 8.42; N, 4.66.
5-(2’-Deoxy-D-ribofuranosyl)-6-methyl-2-phenoxyacetylaminopyridine (4a)
Under an N2 atmosphere, 1 M tetra-n-butylammonium fluoride in THF (1.23 mL, 1.23 mmol) was added to a solution of 3a (235 mg, 0.410 mmol) in anhydrous THF (5 mL) at 0 ºC and the mixture was stirred at room temperature for 12 h. The reaction mixture was concentrated under reduced pressure and the residue was purified by flash column chromatography (1:10 Hexane-EtOAc) to give 4a (131 mg, 89%); Colorless crystals; mp 149-152 °C; [α]D28 48.5 (c 1.00, CH3OH); IR νmax (KBr): 3381, 2935, 1694, 1595, 1532, 1495, 1461, 1394, 1295, 1241, 1083, 1049 cm-1; 1H-NMR (CD3OD, 400 MHz) δ: 1.84 (1H, ddd J = 6.5, 10.5, 13.0 Hz), 2.27 (1H, ddd, J = 1.5, 5.0, 13.0 Hz), 2.46 (3H, s), 3.69 (2H, d, J = 5.0 Hz), 3.94 (1H, dt, J = 2.5, 5.0 Hz), 4.33 (1H, ddd, J = 1.5, 2.5, 6.5 Hz), 4.68(2H, s), 5.29 (1H, dd, J = 5.0, 10.5 Hz), 7.01 (1H, t, J = 7.5 Hz), 7.05 (2H, d, J = 9.0 Hz), 7.33 (2H, dd, J = 7.5, 9.0 Hz), 7.98 (1H, d, J = 8.5 Hz), 8.00 (1H, d, J = 8.5 Hz); 13C-NMR (CD3OD, 101 MHz) δ: 21.6, 43.3, 63.8, 68.3, 74.3, 77.3, 89.0, 112.9, 115.9, 123.0, 130.7, 133.7, 137.4, 150.2, 154.9, 158.9, 169.3; MS (EI) m/z 358 (M+, 100); HRMS (EI) m/z Calcd for C19H22N2O5: 358.1529. Found 358.1524; Anal. Calcd for C19H22N2O5: C, 63.67 H, 6.19; N, 7.82. Found: C, 63.26; H, 6.13; N, 7.79.
5-(2’-Deoxy-D-ribofuranosyl)-2-phenoxyacetylaminopyridine (4b)
Under an N2 atmosphere, 1 M tetra-n-butylammonium fluoride in THF (0.630 mL, 0.630 mmol) was added to a solution of 3b (145 mg, 0.253 mmol) in anhydrous THF (2 mL) at 0 °C and the mixture was stirred at room temperature for 8 h. The reaction mixture was concentrated under reduced pressure and the residue was purified by flash column chromatography (1:10 Hexane-EtOAc) to give 4b (73 mg, 84%); Colorless crystals; mp 121-124 °C; [α]D25 32.3 (c 1.04, CH3OH); IR νmax (KBr) 3374, 1702, 1600, 1523, 1495, 1411, 1343, 1306, 1242, 1087,1052 cm-1; 1H-NMR (CD3OD, 400 MHz) δ: 1.96 (1H, ddd, J = 6.0, 10.5, 13.0 Hz), 2.22 (1H, ddd, J = 1.5, 5.5, 13.0 Hz), 3.67 (1H, d, J = 5.0 Hz), 3.94 (1H, m), 4.34 (1H, m), 4.69 (2H, s), 5.13 (1H, dd, J = 5.5, 10.5 Hz), 7.00 (1H, t, J = 8.0 Hz), 7.04 (2H, d, J = 8.0 Hz), 7.31 (2H, t, J = 8.0 Hz), 7.86 (1H, dd, J = 1.5, 9.0 Hz), 8.14 (1H, d, J = 9.0 Hz), 8.32 (1H, d, J = 1.5 Hz); 13C-NMR (CD3OD, 101 MHz) δ: 44.7, 63.9, 68.3, 74.4, 79.0, 89.4, 115.2, 115.9, 123.0, 130.7, 135.6, 137.9, 147.1, 151.6, 159.0, 169.4; MS (EI) m/z 344 (M+, 100); HRMS (EI) m/z Calcd for C18H20N2O5: 344.1372. Found 344.1386; Anal. Calcd for C18H20N2O5: C, 62.78; H, 5.85; N, 8.13. Found: C, 62.49; H, 5.81; N, 8.04.
5-(5’-O-Dimethoxytrityl-2’-deoxy-D-ribofuranosyl)-6-methyl-2-phenoxyacetylaminopyridine (5a)
Under an N2 atmosphere, DMTrCl (33 mg, 0.0971 mmol) was added to a solution of 4a (29 mg, 0.0809 mmol) in anhydrous pyridine (1.5 mL) at 0 °C and the mixture was stirred at room temperature for 6 h. After the addition of water at 0 °C, the reaction mixture was concentrated under reduced pressure and the residue was purified by flash column chromatography (100:1 CH2Cl2-CH3OH) to give 5a (50 mg, 94%); White powder; mp 62-63 °C; [α]D28 12.9 (c 1.02, CHCl3); IR νmax (KBr): 1699, 1600, 1516, 1461, 1394, 1297, 1245, 1178, 1081, 1035 cm-1. 1H-NMR (CDCl3, 400 MHz) δ: 1.89 (1H, ddd J = 6.5, 10.0, 13.0 Hz), 2.27 (1H, ddd, J = 2.5, 5.5, 13.0 Hz), 2.43 (3H, s), 3.28 (1H, dd, J = 5.5, 10.0 Hz), 3.40 (1H, dd, J = 5.0, 10.0 Hz), 3.80 (6H, s), 3.93 (1H, ddd, J = 3.0, 5.0, 5.5 Hz), 4.42 (1H, ddd, J = 2.5, 3.0, 6.5 Hz), 4.69 (2H, s), 5.29 (1H, dd, J = 5.5, 10.0 Hz), 6.80-6.85 (4H, m), 7.22-7.46 (14H, m), 7.79 (1H, d, J = 9.0 Hz), 7.86 (1H, brs), 7.96 (1H, d, J = 9.0 Hz); 13C-NMR (CDCl3, 101 MHz) δ: 21.1, 43.6, 55.2, 64.3, 67.4, 74.6, 77.6, 86.3, 86.5, 113.2, 113.8, 114.9, 122.4, 126.9, 127.8, 128.1, 129.8, 130.1, 133.5, 135.9, 136.4, 144.7, 146.0, 149.9, 156.9, 158.4, 166.8; MS (FAB) m/z 661 [M+H]+; HRMS (FAB) m/z Calcd for C40H41N2O7 [M+H]+: 661.2908. Found 661.2917.
5-(5’-O-Dimethoxytrityl-2’-deoxy-D-ribofuranosyl)-2-phenoxyacetylaminopyridine (5b)
Under an N2 atmosphere, DMTrCl (64 mg, 0.290 mmol) was added to a solution of 4b (50 mg, 0.145 mmol) in anhydrous pyridine (2 mL) at 0 °C and the mixture was stirred at room temperature for 10 h. After the addition of water at 0 ºC, the reaction mixture was concentrated under reduced pressure and the residue was purified by flash column chromatography (100:1 CH2Cl2-CH3OH) to give 5b (93 mg, 98%); White powder; mp 60-61 °C; [α]D24 10.0 (c 1.01, CHCl3); IR νmax (KBr): 1606, 1510, 1496, 1303, 1250, 1176, 1083, 1035 cm-1; 1H-NMR (CDCl3, 400 MHz) δ: 2.04 (1H, ddd J = 6.5, 10.0, 13.0 Hz), 2.26 (1H, ddd, J = 2.5, 6.0, 13.0 Hz), 3.26 (1H, dd, J = 6.0, 10.0 Hz), 3.36 (1H, dd, J = 4.5, 10.0 Hz), 3.79 (6H, s), 4.07 (1H, ddd, J = 3.0, 4.5, 6.0 Hz), 4.46 (1H, ddd, J = 2.5, 3.0, 6.5 Hz), 4.69 (2H, s), 5.18 (1H, dd, J = 6.0, 10.0 Hz), 6.81-6.85 (4H, m), 6.99 (2H, d, J = 7.5 Hz), 7.05 (1H, t, J = 7.5 Hz), 7.20-7.37 (9H, m), 7.44 (2H, d, J = 7.5 Hz), 7.75 (1H, dd, J = 2.0, 8.5 Hz), 8.24 (1H, d, J = 8.5 Hz), 8.30 (1H, d, J = 2.0 Hz), 8.93 (1H, brs); 13C-NMR (CDCl3, 101 MHz) δ: 43.6, 55.1, 64.3, 67.3, 74.4, 77.5, 86.2, 86.5, 113.1, 113.8, 114.7, 122.3, 126.8, 127.8, 128.1, 129.8, 130.0, 133.9, 135.8, 136.4, 144.7, 145.8, 149.8, 156.9, 158.4, 166.8; MS (FAB) m/z 647 [M+H]+; HRMS (FAB) m/z Calcd for C39H39N2O7 [M+H]+: 647.2752. Found 647.2761; Anal. Calcd for C39H38N2O7: C, 72.43 H, 5.92; N, 4.33. Found: C, 72.23; H, 6.22; N, 4.13.
5-{5’-O-Dimethoxytrityl-3’-O-2-cyanoethoxy(diisopropylamino)phosphino-2’-deoxy-D-ribofuranosyl}-6-methyl-2-phenoxyacetylaminopyridine (6a)
Under an N2 atmosphere, DIPEA (53 µL, 0.303 mmol) and 2-cyanoethyl N,N-diisopropylchlorophosphoramidite (20 µL, 0.0908 mmol) were added to a solution of 5a (40 mg, 0.0605 mmol) in anhydrous CH2Cl2 (1.5 mL) at 0 °C and the mixture was stirred at room temperature for 12h. After the addition of saturated aqueous NaHCO3 at 0 °C, the reaction mixture was stirred at room temperature for 1h and diluted with EtOAc, washed with saturated aqueous NaHCO3, water and brine, dried over Na2SO4, and concentrated. The crude product was purified by flash column chromatography (4:1 Hexane-EtOAc) to give 6a (43 mg, 83%); White amorphous; mp 41-43 °C; 1H-NMR (CDCl3, 400 MHz) δ: 1.08-1.29 (12H, m), 1.86-1.89 (1H, m), 2.25-2.40 (1H, m), 2.44 (3H, s), 2.41-2.63 (2H, m), 3.15-3.90 (12H, m), 4.18-4.22 (1H, m), 4.42-4.48 (1H, m), 4.60-4.61 (2H, m), 5.25-5.31 (1H, m), 6.78-6.85 (4H, m), 6.98-7.08 (3H, m), 7.17-7.38 (11H, m), 7.85-7.92 (1H, m), 8.03-8.08 (1H, m), 8.84 (1H, brs); 31P-NMR (CDCl3, 162 MHz) δ: 148.6, 149.0; MS (FAB) m/z 861 [M+H]+; HRMS (FAB) m/z Calcd for C49H58N4O8P [M+H]+: 861.3987. Found 861.3967.
5-{5’-O-Dimethoxytrityl-3’-O-2-cyanoethoxy(diisopropylamino)phosphino-2’-deoxy-D-ribofuranosyl}-2-phenoxyacetylaminopyridine (6b)
Under an N2 atmosphere, DIPEA (36 µL, 0.209 mmol) and 2-cyanoethyl N,N-diisopropylchlorophosphoramidite (14 µL, 0.0626 mmol) were added to a solution of 5b (27 mg, 0.0417 mmol) in anhydrous CH2Cl2 (2 mL) at 0 °C and the mixture was stirred at room temperature for 3.5 h. After the addition of saturated aqueous NaHCO3 at 0 °C, the reaction mixture was stirred at room temperature for 1 h and diluted with EtOAc, washed with saturated aqueous NaHCO3, water and brine, dried over Na2SO4, and concentrated. The crude product was purified by flash column chromatography (4:1 Hexane-EtOAc) to give 6b (30 mg, 86%); White amorphous; mp 40-42 °C; 1H-NMR (CDCl3, 400 MHz) δ: 1.09-1.20 (12H, m), 1.98-2.07 (1H, m), 2.31-2.48 (2H, m), 2.62 (1H, t, J = 6.5 Hz), 3.23-3.34 (2H, m), 3.54-3.87 (10H, m), 4.20-4.24 (1H, m), 4.51-4.57 (1H, m), 4.63 (2H, s), 5.14-5.18 (1H, m), 6.80-6.83 (4H, m), 6.99-7.07 (3H, m), 7.20-7.46 (11H, m), 7.76-7.79 (1H, m), 8.23-8.25 (1H, m), 8.32-8.34 (1H, m), 8.93 (1H, brs); 31P-NMR (CDCl3, 162 MHz) δ: 148.0, 148.1; MS (FAB) m/z 847 [M+H]+; HRMS (FAB) m/z Calcd for C48H56N4O8P [M+H]+: 847.3830. Found 847.3836.
Synthesis of oligonucleotides
TFO-1–TFO-8 were synthesized on an automated DNA synthesizer (Applied Biosystems ExpediteTM 8909) using a standard phosphoramidite protocol (DMTr-ON mode).10 Syntheses were performed on 0.2 or 1.0 µmol scale. TFOs were cleaved from the CPG supports and deprotected by treatment with ca. 1 mL 28% ammonium aqueous solution (room temperature, 2 h to 55 °C, 24-48 h). After removal of ammonia in vacuo, the obtained crude TFOs were purified with Sep-Pak® Plus C18 cartridges (Waters) followed by reversed-phase HPLC (Waters XBridge® MS C18 2.5 µm, 10 x 50 mm). The composition of the TFOs was confirmed by MALDI-TOF mass analysis. MALDI-TOF mass data ([M-H]-) for TFO-1–TFO-8; TFO-1, found 4478.62 (calcd 4478.01); TFO-2, found 4463.73 (calcd 4463.99); TFO-3, found 4479.54 (calcd 4480.98); TFO-4, found 4494.27 (calcd 4495.00); TFO-5, found 4442.21 (calcd 4443.05); TFO-6, found 4400.50 (calcd 4400.97); TFO-7, found 4451.99 (calcd 4451.94); TFO-8, found 4494.35 (calcd 4494.02).
UV-Melting experiments
UV-Melting experiments were carried out on SHIMADZU UV-1650 and SHIMADZU UV-1800 spectrometers equipped with Tm analysis accessory. Equimolecular amounts of the target hairpin-loop dsDNA and TFOs were dissolved in 10 mM sodium cacodylate buffer (pH 6.8) containing 100 mM KCl and 50 mM MgCl2 to give a final each strand concentration of 1.89 µM. The samples were annealed by heating at 100 °C followed by slow cooling to 5 °C. The melting profiles were recorded at 260 nm from 5 ºC to 90 °C at a scan rate of 0.5 °C/min.
ACKNOWLEDGEMENT
This work was supported in part by the Program to Disseminate Tenure Tracking System of the Ministry of Education, Culture, Sports, Science and Technology, Japan (MEXT), a Grand-in-Aid for Scientific Research on Innovative Areas (No. 22136006) from MEXT and a Grant-in-Aid for JSPS Fellows of Japan Society for the Promotion of Science (JSPS).
References
1. Reviews: D. Praseuth, A. L. Guieysse, and C. Hélène, Biochim. Biophys. Acta, 1999, 1489, 181; CrossRef M. Faria and C. Giovannangeli, J. Gene Med., 2001, 3, 299; CrossRef M. P. Knauert and P. M. Glazer, Hum. Mol. Genet., 2001, 10, 2243; CrossRef M. M. Seidman and P. M. Glazer, J. Clin. Invest., 2003, 112, 487; J. Y. Kuan and P. M. Glazer, Methods Mol. Biol., 2004, 262, 173; R. I. Mahato, K. Chen, and R. V. Guntaka, Expert Opin. Drug Delivery, 2005, 2, 3; CrossRef J. Y. Chin, E. B. Schleifman, and P. M. Glazer, Front. Biosci., 2007, 12, 4288; CrossRef P. Simon, F. Cannata, J.-P. Concordet, and C. Giovannangeli, Biochimie, 2008, 36, 5123; J. Y. Chin and P. M. Glazer, Mol. Carcinog., 2009, 48, 389; CrossRef A. Mukherjee and K. M. Vasquez, Biochimie, 2011, 93, 1197. CrossRef
2. J. S. Lee, M. L. Woodsworth, L. J. P. Latimer, and A. R. Morgan, Nucleic Acids Res., 1984, 12, 6603; CrossRef T. J. Povsic and P. B. Dervan, J. Am. Chem. Soc., 1989, 111, 3059; CrossRef L. E. Xodo, G. Manzini, F. Quadrifoglio, G. A. van der Marel, and J. H. van Boom, Nucleic Acids Res., 1991, 19, 5625. CrossRef
3. A. Ono, P. O. P. Ts’o, and L. Kan, J. Am. Chem. Soc., 1991, 113, 4032; CrossRef P. S. Miller, P. Bhan, C. D. Cushman, and T. L. Trapane, Biochemistry, 1992, 31, 6788; CrossRef S. H. Krawczyk, J. F. Milligan, S. Wadwani, C. Moulds, B. C. Froehler, and M. D. Matteucci, Proc. Natl. Acad. Sci. U.S.A., 1992, 89, 3761; CrossRef J. S. Koh and P. B. Dervan, J. Am. Chem. Soc., 1992, 114, 1470; CrossRef M. C. Jetter and F. W. Hobbs, Biochemsitry, 1993, 32, 3249; CrossRef I. Radhakrishnan, D. J. Patel, E. S. Priestley, H. M. Nash, and P. B. Dervan, Biochemsitry, 1993, 32, 11228; CrossRef G. Xiang, W. Soussou, and L. W. McLaughlin, J. Am. Chem. Soc., 1994, 116, 11155; CrossRef E. S. Priestley and P. B. Dervan, J. Am. Chem. Soc., 1995, 117, 4761; CrossRef R. Berressem and J. W. Engles, Nucleic Acids Res., 1995, 23, 3465; CrossRef U. von Krosigk and S. A. Benner, J. Am. Chem. Soc., 1995, 117, 5361; CrossRef J. Hubziker, E. S. Priestley, H. Brunar, and P. B. Dervan, J. Am. Chem. Soc., 1995, 117, 2661; CrossRef K. M. Koshlap, P. Schultze, H. Brunar, and P. B. Dervan, Biochemsitry, 1997, 36, 2659; CrossRef G. Xiang, R. Bogacki, and L. W. McLaughlin, Nucleic Acids Res., 1996, 24, 1963; CrossRef E. Bédu, R. Benhida, M. Devys, and J.-L. Fourrey, Tetrahedron Lett., 1999, 40, 835; CrossRef U. Parsch and J. W. Engles, Chem. Eur. J., 2000, 6, 2409; CrossRef K. Miyata, R. Tamamushi, H. Tsunoda, A. Ohkubo, K. Seio, and M. Sekine, Org. Lett., 2009, 11, 605; CrossRef F. Seela, D. Jiang, and S. Budow, ChemBioChem, 2010, 11, 1443; CrossRef S.-Q. Cao, I. Okamoto, H. Tsunoda, A. Ohkubo, K. Seio, and M. Sekine, Tetrahedron Lett., 2011, 52, 407. CrossRef
4. S. Hildbrand, A. Braser, S. P. Parel, and C. J. Leumann, J. Am. Chem. Soc., 1997, 119, 5499. CrossRef
5. S. Hildbrand and C. Leumann, Angew. Chem., Int. Ed. Engl., 1996, 35, 1968; CrossRef P. J. Bates, C. A. Laughton, T. C. Jenkins, D. C. Capaldi, P. D. Roselt, C. B. Reese, and S. Niedle, Nucleic Acids Res., 1996, 24, 4176. CrossRef
6. Fox’s group reported that TFO using four non-natural nucleobases (BAU, MeP, APP, and S) formed a stable triplex to target DNA bearing AT, GC, CG, and TA base pairs. D. A. Rusling, V. E. C. Powers, R. T. Ranasinghe, Y. Wang, S. D. Osborne, T. Brown, and K. R. Fox, Nucleic Acids Res., 2005, 33, 3025. CrossRef
7. Ab initio calculation (HF/6-31G*) gave the result that PMe-anti was 2.24 kcal/mol more stable than PMe-syn.
8. Z. Sun, S. Ahmed, and L. W. McLaughlin, J. Org. Chem., 2006, 71, 2922. CrossRef
9. McLaughlin’s group reported that they synthesized oligonucleotides introducing PMe protected by an amidine group at the exocyclic amino group and evaluated their duplex-forming ability. Z. Sun and L. W. McLaughlin, Biopolymers, 2007, 87, 183. CrossRef
10. Leumann’s group reported that the N-Pac-protected MeP and 2’-O-methyl derivative of P were partially converted into the acetylated congener through oligonucleotide synthesis.4 However, no acetylation of PMe and P was observed in our experiments.