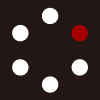
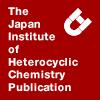
HETEROCYCLES
An International Journal for Reviews and Communications in Heterocyclic ChemistryWeb Edition ISSN: 1881-0942
Published online by The Japan Institute of Heterocyclic Chemistry
e-Journal
Full Text HTML
Received, 20th May, 2013, Accepted, 2nd July, 2013, Published online, 11th July, 2013.
DOI: 10.3987/REV-13-SR(S)1
■ Synthesis of Heterocycles via Nucleophilic Substitution of Hydrogen in Nitroarenes
Mieczysław Mąkosza* and Krzysztof Wojciechowski
Institute of Organic Chemistry, Polish Academy of Sciences, ul. Kasprzaka 44/52, P.O.Box 58, PL-01-224 Warszawa 42, Poland
Abstract
The main message of this review is that nucleophilic substitution of hydrogen in nitroarenes and other electron-deficient arenes is primary general process of wide scope and great value in organic synthesis, whereas conventional nucleophilic substitution of halogens, SNAr reaction, is just a secondary process. Nucleophilic substitution of hydrogen in electron-deficient arenes is a powerful tool in organic synthesis, effectively applicable to the synthesis of heteroaromatic compounds.Contents
1. Introduction
2. Basic Principles and Pathways of Nucleophilic Substitution of Hydrogen
2.1. Oxidative Nucleophilic Substitution of Hydrogen
2.2. Vicarious Nucleophilic Substitution of Hydrogen
2.3. Conversion of the σH adducts into Nitrosoarenes
3. Construction of Heterocyclic Rings via Nucleophilic Substitution of Hydrogen
3.1. Indoles
3.2. Quinolines
3.3. Heterocycles from 2-Nitrosodiphenylamines
4. Conclusions
5. References
1. INTRODUCTION
One of the major approaches to synthesis of benzannulated heterocyclic systems consists in introduction of carbon and heteroatom substituents into aromatic rings, followed by appropriate transformations. Particularly valuable for this purpose are reactions of arenes, activated via H-Li exchange, with mild electrophilic reactants. This approach, particularly -directed ortho metalation (DoM)1 is an efficient tool for synthesis of arenes containing functionalized substituents in ortho relation, versatile educts in synthesis of heterocycles. Another valuable and versatile tool for introduction substituents into aromatic rings is nucleophilic substitution of hydrogen.2,3 Although this process is limited to electron-deficient arenes, particularly nitroarenes, presence of the nitro group is highly beneficial for further synthesis of nitrogen heterocycles.4 In this short review we will present the basic principles and pathways of nucleophilic substitution of hydrogen and use of these processes for synthesis of indoles and some other nitrogen-heteroarenes.
2. BASIC PRINCIPLES AND PATHWAYS OF NUCLEOPHILIC SUBSTITUTION OF HYDROGEN
Reactions of nucleophiles with nitroarenes, also those containing halogens in activated ortho and para positions, proceed via fast and reversible addition in positions occupied by hydrogen to form σH adducts. Since this process is connected with dearomatization and hydride anion is unable to depart from anionic σH adduct spontaneously, these adducts usually dissociate. Slower addition in positions occupied by halogens X results in formation of σX adducts, subsequent fast departure of anions X− gives products of conventional SNAr reaction. This situation is presented in the Scheme 1.
Initially formed σH adducts of nucleophiles to nitroarenes can be converted into products of nucleophilic substitution of hydrogen in a variety of ways such as oxidation, vicarious substitution (VNS), intramolecular redox processes giving nitrosoarenes etc. Proper selection of nucleophilic agents and reaction conditions can assure fast conversion of the σH adducts hence the nucleophilic substitution of hydrogen becomes primary dominating process, whereas SNAr is just a secondary reaction.2,4-6
2.1. OXIDATIVE NUCLEOPHILIC SUBSTITUTION OF HYDROGEN
The most obvious way of conversion of anionic σH adducts into products of substitution of hydrogen appears to be abstraction of the hydride anion by external oxidants in a process known as oxidative nucleophilic substitution of hydrogen (ONSH).5 This way suffers, however severe limitations, because generally nucleophiles, particularly carbanions, are sensitive to oxidation, thus, due to reversibility of the addition, the oxidation of nucleophiles could dominate. Because of that oxidative nucleophilic substitution of hydrogen can proceed when nucleophiles are resistant toward oxidation or when the addition is complete due to the nature of the reactants and reaction conditions.
The reaction of potassium hydroxide with p-chloronitrobenzene is an excellent example of the ONSH with nucleophiles resistant towards oxidation and illustration of the relation of rates of nucleophilic addition in various positions of halonitroarenes. In every textbook on organic chemistry “hydrolysis” of p-chloronitrobenzene to p-nitrophenol with a solution of KOH at elevated temperature proceeding via SNAr is described. On the other hand treatment of p-chloronitrobenzene with a solution of KOH and oxygen in liquid ammonia at -40 ºC results in the ONSH of hydrogen.7
These results confirm that formation of σH adducts proceeds much faster than isomeric σCl adducts. Since KMnO4 forms stable solutions in liquid ammonia, whereas it can oxidize σH adducts, such solutions are excellent aminating agents of electron-deficient arenes and heteroarenes.8 For instance, 1-chloro-2,4-dinitrobenzene dissolved in this solution gave exclusively 5-chloro-2,4-dinitroaniline - product of ONSH, the SNAr of chlorine does not proceed under these conditions (Scheme 3).9
ONSH can proceed efficiently also with nucleophiles sensitive to oxidation such as the Grignard reagents and stabilized carbanions provided that the addition is complete. In the case of the former nucleophiles the addition proceeds irreversible hence σH adducts, once formed, can be oxidized by subsequently added external oxidants. Thus addition of the Grignard reagents to nitroarenes followed by oxidation of the formed σH adduct is an effective way of alkylation of nitroarenes (Scheme 4).10,11
On the other hand addition of carbanions to nitroarenes is a reversible process, but the equilibrium of the addition can be shifted towards σH adducts due to high nucleophilicity of the carbanions and low temperature.12 Secondary (methylenic) carbanions add in the ortho and para positions to the nitro group,13 whereas tertiary (methinic) carbanions add preferentially in the para position12 (Scheme 5 and 6).
The σH adducts such formed can be oxidized by external oxidants such as KMnO4, DDQ, etc. It should be stressed that these reactions should be carried out at temperature below -40 ºC, hence due to the entropy factor dissociation of the σH adducts is disfavoured. Oxidation of the σH adducts with dimethyldioxirane results in replacement of the nitro group by the hydroxy one, thus enabling synthesis of substituted phenols directly from the nitroarenes (Scheme 6).14
2.2. VICARIOUS NUCLEOPHILIC SUBSTITUTION OF HYDROGEN
Much more general and vertsatile variant of nucleophilic substitution of hydrogen is the vicarious nucleophilic substitution (VNS) with nucleophiles that contain a nucleofugal group L located at the nucleophilic center, such as for instance α-chlorocarbanions.15 The σH adducts of α-chlorocarbanions to nitroarenes undergo base-induced β-elimination of HCl on the expense of the ring hydrogen to form ortho and/or para nitrobenzylic carbanions, that upon protonation give products of the VNS.16 This substitution of hydrogen in o- and p-halonitrobenzenes proceeds faster than the conventional SNAr of halogen, thus confirms the relation of rates of nucleophilic addition mentioned earlier (Scheme 1). The reaction is of general character in respect to nitroarenes and other electron deficient arenes (azines, azulenes etc.) as well as carbanions that should contain halogens or other nucleofugal groups L (e.g. PhO, MeO, PhS, MeS), able to be eliminated as HL from the σH adducts. Thus when para-fluoronitrobenzene was reacted with carbanion of chloromethyl phenyl sulfone generated in the presence of an excess of t-BuOK in DMF at low temperature, hydrogen in the ortho position to the nitro group was replaced with phenylsulfonylmethyl substituent.17 Under these conditions disfavouring equilibration the conventional replacement of fluorine was not observed. On the other hand under the conditions that favour equilibration and disfavour the β-elimination (low concentration of carbanion, room temp.) SNAr of fluorine can be the major process (Scheme 7).
It should be stressed that under the conditions disfavouring the equilibration of the σH adduct such α-halocarbanions react even with 1-fluoro-2,4-dinitrobenzene (the Sanger reagent) exclusively along the VNS pathway (Scheme 8).18
Similarly VNS reaction takes place with a variety of other nitroarenes. Since products of the VNS are formed as nitrobenzylic carbanions, the reaction proceeds selectively as monosubstitution. However m-dinitrobenzene can form products of mono- and di-substitution in the reaction with, respectively, equimolar quantity and excess of carbanion of chloromethyl phenyl sulfone and base (Scheme 9).19 In 1,3,5-trinitrobenzene replacement of all three hydrogen atoms is possible.19
Some other examples of carbanion precursors that enter the VNS reaction are: ClCH2COOtBu,20,21 Cl2CHCOOtBu,22,23 ArOCH2CN,24-28 CHCl3,29-33 Ph2CHSPh34 etc. It should be mentioned that orientation of the substitution can be controlled by the structure of the reactants and the reaction conditions. Taking into account wide scope of this reaction in respect to nitroarenes and carbanions, the VNS is a general, efficient and versatile tool for introduction of functionalized carbon substituents into nitroarenes and their heteroanalogues. Some representative examples of the VNS are shown in Scheme 10.
Besides of being valuable method of organic synthesis, VNS was used for evaluation of effect of substituents on electrophilic activity of electron-deficient arenes. Effect of substituents on electrophilic substitution in arenes, in other words nucleophilicity of the aromatic rings, was thoroughly studied and discussed in basic text-books of organic chemistry. On the other hand there are no reliable data concerning influence of substituents on the electrophilicity of the aromatic rings and their effect on nucleophilic aromatic substitution. The data obtained on the basis of SNAr reactions38 are not reliable because SNAr is a secondary process. We have shown that VNS can serve as an efficient tool for such evaluation.39,40
VNS is not limited to introduction of carbon substituents into electron-deficient rings. Similar process takes place with oxygen and nitrogen nucleophiles, provided they contain leaving group at the nucleophilic center. Thus nitroarenes can be efficiently hydroxylated in the reaction with t-butyl and cumyl hydroperoxides carried out in the presence of an excess of base.41 Replacement of hydrogen in nitroarenes for hydroxyl group in the reaction with these hydroperoxide anions proceeds faster than SNAr of chlorine. For instance, treatment of 2,4-dinitrochlorobenzene with these peroxides and potassium t-butoxide gave 2,4-dinitro-5-chlorophenol in excellent yield (Scheme 11).41
A few VNS aminating agents were developed – derivatives of hydrazine and hydroxylamine such as 4-amino-1,2,4-triazole,42 1,1,1-trimethylhydrazonium iodide,43 arylsulfenamides,44 methoxyamine,45 etc (Scheme 12). Treatment of nitroarenes with these aminating agents in the presence of strong base, usually potassium t-butoxide, results in synthesis of nitroanilines. Also this process proceeds faster than conventional replacement of halogen.
2.3. CONVERSION OF THE σH ADDUCTS INTO NITROSOARENES
The third interesting way of conversion of anionic σH aducts of nucleophiles to nitroarenes into products of nucleophilic substitution of hydrogen consists in formal elimination of OH− anions to produce substituted nitrosoarenes. This process can be induced by O-protonation of the σH adducts with protic solvents, association with Lewis acids, O-silylation etc. Overall reaction proceeds according to an intramolecular redox stoichiometry. Since nitrosoarenes and particularly nitroso group itself, are active electrophiles the initially formed nitrosoarenes usually cannot be isolated but undergo further transformations. Their fate depends on kind of the reactants and the reaction conditions. For instance in protic solvents carbanion of phenylacetonitrile reacts with para-chloronitrobenzene to form benzisoxazole,46 whereas similar reaction with ortho-chloronitrobenzene gave the nitroso compound isolated in its tautomeric form of a quinone oxime (Scheme 13).47 Both of these products are valuable starting materials in manufacturing of substituted 2-aminobenzophenones48,49 and heterocycles.50-53
Conversion of the σH adducts of some carbanions into substituted nitrosoarenes is a key step in synthesis of condensed six-membered heterocycles as exemplified by the reaction of 2-nitrothiophene that upon treatment with allyl tolyl sulfone in the presence of DBU, bis-trimethylsilylacetamide (BSA) and MgCl2 is transformed into thieno[2,3-b]pyridine (Scheme 14).54
In recent years it was reported that anilines react with nitroarenes in the presence of strong base, potassium t-butoxide, to form σH aducts that are converted into 2-nitrosodiarylamines.55-57 Particularly interesting and important is this reaction with p-halonitrobenzenes to produce halonitrosodiarylamines, products of nucleophilic substitution of hydrogen. This reaction proceeds efficiently even with p-fluoronitrobenzene (Scheme 15), being faster than known, common SNAr of fluorine.58 This simple and general reaction opens wide possibilities in synthesis of condensed heterocycles,59,60 particularly phenazines.56,57
Reactions of 1-nitronaphthalene and nitroquinolines with dimethylphosphite anion result in formation of benzazepines via conversion of σH adduct into nitroso compound, deoxygenation of the nitroso group to nitrene and the ring expansion (Scheme 16).61
Detailed discussion of various ways of conversion of anionic σH adducts into products of nucleophilic substiution of hydrogen and relation of the rates of competing reactions is in our recent reviews.2,4-6,62
3. CONSTRUCTION OF HETEROCYCLIC RINGS VIA NUCLEOPHILIC SUBSTITUTION OF HYDROGEN
3.1. INDOLES
The indole ring system can be constructed via nucleophilic substitution of hydrogen on two major ways. The first one consists in use of m-nitroanilines as the educts, hence the nitrogen of the amino group is in the produced heterocyclic ring. Introduction of functionalized carbon substituents into meta-nitroanilines ortho- or para to the nitro group and ortho to the amino group followed by intramolecular reactions gives indoles. Thus the simplest and the most efficient construction of the indole ring is direct reaction between m-nitroaniline, that can contain additional substituents, and enolate anions of ketones. For instance, treatment of an aerated solution of m-nitroaniline and acetone, cyclohexanone or other enolizable ketone in DMSO with t-BuOK results in direct formation of 4- and/or 6-nitroindoles, substituted in the indole ring as exemplified in the Scheme 17.63
The reaction proceeds via addition of the enolate anions to the aromatic ring in position ortho to the amino group. Further oxidation of the σH adducts with atmospheric oxygen followed by Baeyer type intramolecular condensation of the intermediate o-aminobenzylic ketones gives nitroindoles. This very simple method of synthesis of substituted indoles is of general character and is applicable for large scale operations.64 In a similar way 2-amino-4-(or 6)-nitroindoles can be obtained in the reaction of m-nitroaniline with carbanions of nitriles (Scheme 18).65
Conversion of m-nitroanilines into m-nitrobenzoisonitriles followed by VNS with α-chlorocarbanions results in formation of nitrobenzylic carbanions that contain in the ortho position isonitrile functionality. Subsequent intramolecular addition of the carbanion to isonitrile gives substituted nitroindoles (Scheme 19).66
The second way of construction of the indole ring via nucleophilic substitution of hydrogen consists in introduction of functionalized carbon substituents in position ortho to the nitro group. Further reactions of such products provide indoles in which nitrogen of the nitro group is incorporated into the heterocyclic ring. The simplest variant of this approach is ortho cyanomethylation of nitroarenes via the VNS reaction followed by catalytic hydrogenation of the produced o-nitroarylacetonitriles. Synthesis of indoles via catalytic hydrogenation of o-nitroarylacetonitriles was known from 1955,67 however it was of limited value, because the nitriles were not available. Only, when these nitriles become readily available via VNS reaction24,25 this method gained great practical value.25,26,68-73 Generality and practical value of this way of synthesis of indoles is illustrated by synthesis of all isomeric 4-, 5-, 6-, and 7-methoxyindoles via VNS cyanomethylation of nitro anisoles, optionally containing halogens in the ring (Scheme 20).25
This approach was used for synthesis of natural products, pharmaceuticals and a variety of azaindoles.71,74 In the synthesis of eudistomin C, a marine origin antiviral agents containing 5-methoxyindole fragment, indole units were prepared via VNS with aryloxyacetonitriles (Scheme 21).70 Similarly indoles containing pentafluorosulfanyl substituents were synthesised.75
Synthesis of indoles from ortho-nitroarylacetonitriles can also be executed in another way. Products of allylation or benzylation of such nitriles when treated with a base undergo multistep transformations to produce 1-hydroxyindoles. Thus alkylation of ortho-nitroarylacetonitriles with 3-phenylallylbromide followed by treatment of the product with trimethylamine and chlorotrimethyl silane results in formation of 1-hydroxy-2-phenylvinyl-3-cyanoindoles. The reaction proceeds apparently via O-silylation of the nitronate anions followed by elimination of trimethylsilanol and cyclization of the intermediate nitrosoarenes (Scheme 22).76
o-Nitroarylacetonitriles enter readily the Knoevenagel condensation with aliphatic aldehydes.77-79 The produced unsaturated nitriles, treated with base are converted into N-hydroxy indoles or quinoline-N-oxides depending on reaction conditions (Scheme 23).80
Palladium catalyzed reduction of such unsaturated nitriles with CO when performed under neutral conditions gives indoles while in the presence of base leads to quinolines (Scheme 24).79 Ester of azaindole 3-carboxylic acid is simply prepared from product of VNS in 3-nitropyridine with chloroacetate via analogous condensation with trioxane and Pd catalyzed reduction with carbon monoxide.79
Enolates of α-chloroketones are rather weak nucleophiles thus they enter VNS reaction only with highly electrophilic nitroarenes.81 For instance, chloromethyl t-butyl ketone reacts with m-dinitrobenzene in the presence of DBU to form dinitrobenzyl ketone that is reduced with tin(II) chloride to 6-nitro-N-hydroxyindole (Scheme 25).81
Substituted o-nitrobenzyl phenyl sulfones, products of the VNS reaction of nitroarenes with chloromethyl phenyl sulfones are also versatile educts in synthesis of indoles. Reduction of such sulfones to ortho-aminobenzyl sulfones and conversion of the amino group into imidate82 or imine83 functionality followed by treatment with base results in cyclization to form substituted indoles. This protocol is particularly useful because it is possible to direct the VNS reaction selectively in the ortho position to the nitro group when carried out in THF with t-BuOK.84 This procedure was used for the synthesis of 5- and 7-bromo-3-sulfonylindoles that were subsequently converted into norepinephrine reuptake inhibitors and 5-HT2A receptor antagonists (Scheme 26).85,86 Similarly were synthesised 3-phenylsulfonylindoles containing pentafluorosulfanyl substituents.75
Alternatively N-substituted 3-phenylsulfonylindoles were synthesized via reductive N-alkylation of ortho-aminobenzyl sulfones with ketones followed by condensation with dimethylformaldehyde-dimethylacetal (DMF-DMA) and cyclization (Scheme 27).87
Addition of phosphonium ylide generated from allyl triphenylphosphonium chloride to 1-nitronaphthalene and 5-nitroquinoline in the presence of titanium isopropoxide forms an unstable N-hydroxyindole derivative that upon action of ethyl bromoacetate and triethylamine transforms into benzo- or pyridoindoles as shown in the Scheme 28.88
Indole ring system is present in many pharmaceuticals, natural products, and biologically active compounds, thus there is continuous interest in efficient methods of synthesis of indoles. In recent years numerous reviews are published presenting methods of indole synthesis based on transition metals catalyzed reactions.89-92 These interesting and valuable methods have two disadvantages: usually they require rather sophisticated starting materials and the products contain residues of transition metal catalyst. Since pharmaceuticals and compounds tested as potential drugs cannot contain residues of transition metals in quantities exceeding 10 ppm, the indoles obtained by such methods need laborious purification before can be used for such purpose.93 Methods of synthesis of indoles via nucleophilic substitution of hydrogen presented in this short review use readily available starting materials, simple procedures and assure high yields of the required products, that do not contain residues of transition metals, thus are superior to transition metal catalyzed synthesis of indoles.
3.2. QUINOLINES
There are numerous examples of construction of benzopyridines (and among them quinolines) via cascade reactions – conversion of σH adducts of benzylic or allylic carbanions to nitrosoarenes followed by an intramolecular addition to form the ring. Also ortho-nitrobenzyl derivatives have found numerous applications in synthesis of quinolines. Here we briefly present synthesis of quinolines from products of nucleophilic substitution of hydrogen in nitroarenes.
Carbanion generated upon action of DBU on 3-phenylallyl phenyl sulfone reacts with 6-methoxy-3-nitropyridine in the presence of t-butyldimethylsilyl chloride to form substituted naphthyridine (Scheme 29).54 The reaction proceeds presumably via an addition of the sulfone carbanion followed by a conversion of the σH adducts into nitrosoarene and subsequent intramolecular condensation of the newly generated ambident carbanion with the nitroso group.
The usefulness of this reaction was shown in the synthesis of eupolauramine, an alkaloid from the bark of an african plant Euopomatia laurina. This approach consists in addition of carbanion of allyl phenyl sulfone to 1-methoxy-4-nitronaphthalene followed by conversion of the produced σ adduct into nitrosoarene. Further intramolecular condensation produces substituted 1-phenylsulfonyl-4-aza-phenanthrene (Scheme 30).54 The replacement of the phenylsulfonyl group with cyanide followed by a hydrolysis furnishes acid from that the final alkaloid can be obtained following the known procedure. 94
Carbanions of benzyl sulfones and their heterocyclic analogues react similarly with nitroarenes to form condensed heterocycles substituted with arenesulfonyl group as exemplified by reaction of 2-methoxy-5-nitropyridine and 2-thienylmethyl tolyl sulfone in the presence of DBU and N,O-bis-trimethylsilylacetamide (Scheme 31).95
Dianion of ethyl N-pivaloyl-3-aminocrotonate adds to 4-bromo-2-chloro-1-nitrobenzene to form σH-adduct that upon acylation with pivaloyl chloride is transformed into 3-aminoquinoline-2-carboxylic acid derivative, apparently via nitrosoarene (Scheme 32).96
It was shown earlier (Scheme 13) that σH adduct of carbanion of phenylacetonitrile to 4-chloronitrobenzene is converted in protic media into nitrosoarene and finally 2,1-benzisoxazole.46 On the other hand this σH adduct produced in aprotic solvent upon treatment with trialkylchlorosilanes or pivaloyl chloride cyclize to form acridine (Scheme 33).97
Similar transformation was reported to proceed between arylacetonitriles and 3-nitroimidazo[1,2-a]pyridine leading to pyridoimidazoquinoline derivatives that are of interest as highly fluorescent dyes.98 Analogous reaction of this nitroimidazopyridine with 3-indolylacetonitrile leads directly into pentacyclic system (Scheme 34).99
Alkylation of the VNS products – ortho-nitroarylacetonitriles with α-bromoketones provides ketonitriles that upon reduction under mild conditions with tin(II) in ethyl acetate – ethanol mixture form quinoline-4-carbonitriles.100 This reaction sequence applied to nitroindole-5-acetonitriles obtained via the VNS in 5-nitroindole derivative leads to tricyclic cyano substituted pyrrolo[3,2-f]quinoline derivative as exemplified in the Scheme 35.100
These alkylation products when treated with trimethylchlorosilane in presence of base transformed easily into 1-cyano-3-hydroxy-pyrrolo[3,2-e]indoles (Scheme 36).101
Reaction of ortho-nitroarylacetonitriles with the Vilsmeier-Haack reagent prepared from N-methylpyrrolidone followed by a cyclization induced by diazabicycloundecene (DBU) in the presence of bis-trimethylsilylacetamide (BSA) leads directly to pyrrolo[3,2-b]quinoline derivatives (Scheme 37).102
3.3. HETEROCYCLES FROM 2-NITROSODIPHENYLAMINES
As it was mentioned in the Section 2.3 N-anions of anilines add to nitroarenes, also those containing halogens, to form σH adducts that are readily converted into nitroso diarylamines, according to the intramolecular redox stoichiometry.55-57,59 The reaction proceeds particularly well in position ortho to the nitro group thus o-nitrosodiarylamines are readily available. These 2-nitrosodiarylamines are versatile starting materials for synthesis of a variety of heterocycles containing two nitrogen atoms in the ring. Thus simple heating of such 2-nitrosodiarylamine with acetic acid,55,57 a weak base such as K2CO357 or N,O-bis-(trimethylsilyl)acetamide57 results in formation of phenazines. In fact this is the simplest way of synthesis of this important class of heterocycles103-105 that can contain a variety of substituents in well defined positions of the both aromatic rings. This two-step synthesis of phenazines from anilines and nitroarenes resembles the old Wolff-Aue reaction, however the presented procedure assures much higher yields, wide scope of substituents and control of the orientation (Scheme 38).
The versatility of this methodology is presented in the Scheme 39. Two properly chosen pairs of nitroarene-arylamine can react to form analogous phenazines as exemplified by the synthesis of pyrrolo[3,2-a]phenazines from 5-nitroindole derivative and p-anisidine or 5-aminoindole and p-nitroanisole, respectively.106
Condensation of these 2-nitrosodiarylamines with benzyl aryl sulfones opens efficient route to 1,2-diaryl- benzimidazoles (Scheme 40).59 The first step of this reaction is condensation of the benzylic carbanion with the nitroso group (Ehrlich-Sachs reaction) followed by a substitution of the phenylsulfonyl group by the aniline nucleophile. 2-Nitrosodiphenylamines are versatile educts in synthesis of N-arylquinoxalines via base-induced condensation with a variety of CH acids of sufficient acidity stabilized by an alkoxycarbonyl group, such as phenylacetates and their heteroanalogues, cyanoacetates and phosphonylacetates. These reactions provide substituted quinoxalines and quinoxalinones presented in the Scheme 40.60,107
4. CONCLUSIONS
From the results presented in this short review it is evident that nucleophilic substitution of hydrogen is a primary fast reaction, whereas conventional SNAr of halogens just secondary ipso reaction. We have shown that nucleophilic substitution of hydrogen in its three variants: oxidative, vicarious, and conversion of the σH adducts into nitrosoarenes is an efficient and versatile tool for synthesis of heterocyclic systems.
References
1. C. G. Hartung and V. Snieckus, in 'The Directed ortho Metalation Reaction – A Point of Departure for New Synthetic Aromatic Chemistry', ed. by D. Astruc, Weinheim, FRG, 2004.
2. M. Mąkosza, Synthesis, 2011, 2341. CrossRef
3. O. N. Chupakhin, V. N. Charushin, and H. C. van der Plas, 'Nucleophilic Aromatic Substitution of Hydrogen', Academic Press, 1994.
4. M. Mąkosza and K. Wojciechowski, Chem. Rev., 2004, 104, 2631. CrossRef
5. M. Mąkosza, Russ. Chem. Bull., 1996, 45, 491. CrossRef
6. M. Mąkosza, Chem. Soc. Rev., 2010, 39, 2855. CrossRef
7. E. V. Malykhin, A. A. Shtark, and V. D. Shteingarts, Zh. Org. Khim., 1982, 18, 1898.
8. H. C. van der Plas and M. Woźniak, Croat. Chem. Acta, 1986, 59, 33.
9. B. Szpakiewicz and M. Grzegozek, Russ. J. Org. Chem., 2004, 40, 829. CrossRef
10. G. Bartoli, Acc. Chem. Res., 1984, 17, 109. CrossRef
11. M. Mąkosza and M. Surowiec, J. Organomet. Chem., 2001, 624, 167. CrossRef
12. M. Mąkosza and K. Staliński, Chem. Eur. J., 1997, 3, 2025. CrossRef
13. M. Mąkosza and D. Sulikowski, J. Org. Chem., 2009, 74, 3827. CrossRef
14. W. Adam, M. Mąkosza, C.-G. Zhao, and M. Surowiec, J. Org. Chem., 2000, 65, 1099. CrossRef
15. J. Goliński and M. Mąkosza, Tetrahedron Lett., 1978, 19, 3495. CrossRef
16. M. Mąkosza and J. Winiarski, Acc. Chem. Res., 1987, 20, 282. CrossRef
17. T. Glinka and M. Mąkosza, J. Org. Chem., 1983, 48, 3860. CrossRef
18. M. Mąkosza and J. Stalewski, Liebigs Ann. Chem., 1991, 605. CrossRef
19. M. Mąkosza and S. Ludwiczak, J. Org. Chem., 1984, 49, 4562. CrossRef
20. B. Mudryk and M. Mąkosza, Synthesis, 1988, 1007. CrossRef
21. J. M. Bakke, J. Heterocycl. Chem., 2005, 42, 463. CrossRef
22. M. Mąkosza, K. Sienkiewicz, and K. Wojciechowski, Synthesis, 1990, 850. CrossRef
23. M. Mąkosza and M. Białecki, Synlett, 1991, 181. CrossRef
24. M. Mąkosza and J. Winiarski, J. Org. Chem., 1984, 49, 1494. CrossRef
25. M. Mąkosza, W. Danikiewicz, and K. Wojciechowski, Liebigs Ann. Chem., 1988, 203. CrossRef
26. L. Lerman, M. Weinstock-Rosin, and A. Nudelman, Synthesis, 2004, 3043. CrossRef
27. B. A. Czeskis, D. K. Clodfelter, and W. J. Wheeler, J. Labelled Compd. Radiopharm., 2002, 45, 1143. CrossRef
28. J. E. Macor, J. T. Forman, R. J. Post, and K. Ryan, Tetrahedron Lett., 1997, 38, 1673. CrossRef
29. M. Mąkosza and Z. Owczarczyk, J. Org. Chem., 1989, 54, 5094. CrossRef
30. M. D. Crozet, C. Suspene, M. Kaafarani, M. P. Crozet, and P. Vanelle, Heterocycles, 2004, 63, 1629. CrossRef
31. S. Ostrowski, Heterocycles, 1996, 43, 389. CrossRef
32. G. D. Couch, P. J. Burke, R. J. Knox, and C. J. Moody, Tetrahedron, 2008, 64, 2816. CrossRef
33. P. Beier, T. Pastyrikova, and G. Iakobson, J. Org. Chem., 2011, 76, 4781. CrossRef
34. M. Mąkosza, M. Surowiec, and S. Voskresensky, Synthesis, 2000, 1237. CrossRef
35. M. Mąkosza and E. Kwast, Tetrahedron, 1995, 51, 8339. CrossRef
36. M. Achmatowicz, O. R. Thiel, G. Gorins, C. Goldstein, C. Affouard, R. Jensen, and R. D. Larsen, J. Org. Chem., 2008, 73, 6793. CrossRef
37. K. Wojciechowski and M. Mąkosza, Synthesis, 1989, 106. CrossRef
38. J. Miller, 'Aromatic Nucleophilic Substitution', Elsevier, 1968.
39. F. Seeliger, S. Błażej, S. Bernhardt, M. Mąkosza, and H. Mayr, Chem. Eur. J., 2008, 14, 6108. CrossRef
40. S. Błażej and M. Mąkosza, Chem. Eur. J., 2008, 14, 11113. CrossRef
41. M. Mąkosza and K. Sienkiewicz, J. Org. Chem., 1998, 63, 4199. CrossRef
42. A. R. Katritzky and K. S. Laurenzo, J. Org. Chem., 1988, 53, 3978. CrossRef
43. P. F. Pagoria, A. R. Mitchell, and R. D. Schmidt, J. Org. Chem., 1996, 61, 2934. CrossRef
44. M. Mąkosza and M. Białecki, J. Org. Chem., 1998, 63, 4878. CrossRef
45. S. Seko and K. Miyake, J. Chem. Soc., Chem. Commun., 1998, 1519.
46. R. B. Davis and L. C. Pizzini, J. Org. Chem., 1960, 25, 1884. CrossRef
47. R. B. Davis, L. C. Pizzini, and J. D. Benigni, J. Am. Chem. Soc., 1960, 82, 2913. CrossRef
48. J. L. Kim, J. K. Kim, and S. I. Hong, Polymer Bull., 1999, 42, 511. CrossRef
49. E. R. Simas, T. D. Martins, T. D. Z. Atvars, and L. Akcelrud, J. Luminesc., 2009, 129, 119.
50. P. Beier and T. Pastýříková, Beilstein J. Org. Chem., 2013, 9, 411. CrossRef
51. V. Y. Orlov, V. V. Ganzha, A. D. Kotov, and V. G. Sokolov, Russ. J. Org. Chem., 2007, 43, 1502. CrossRef
52. M. Rahimizadeh, M. Pordel, M. Bakavoli, Z. Bakhtiarpoor, and A. Orafaie, Monatsh. Chem., 2009, 140, 633. CrossRef
53. M. Pordel, A. Abdollahi, and B. Razavi, Russ. J. Bioorg. Chem., 2013, 39, 211. CrossRef
54. Z. Wróbel, Eur. J. Org. Chem., 2000, 521. CrossRef
55. Z. Wróbel and A. Kwast, Synlett, 2007, 1525. CrossRef
56. Z. Wróbel and A. Kwast, Synthesis, 2010, 3865. CrossRef
57. A. Kwast, K. Stachowska, A. Trawczyński, and Z. Wróbel, Tetrahedron Lett., 2011, 52, 6484. CrossRef
58. H. Kotsuki, S. Kobayashi, K. Matsumoto, H. Suenaga, and H. Nishizawa, Synthesis, 1990, 1147. CrossRef
59. Z. Wróbel, K. Stachowska, K. Grudzień, and A. Kwast, Synlett, 2011, 1439. CrossRef
60. Z. Wróbel, K. Stachowska, A. Kwast, A. Gościk, M. Królikiewicz, R. Pawłowski, and I. Turska, Helv. Chim. Acta, 2013, 96, 956. CrossRef
61. W. Danikiewicz and M. Mąkosza, J. Org. Chem., 1991, 56, 1283. CrossRef
62. M. Mąkosza and K. Wojciechowski, in 'Nucleophilic Substitution of Hydrogen - an Efficient Tool in Synthesis of Heterocyclic Compounds', ed. by O. Attanasi, Rome, 2011.
63. N. Moskalev, M. Barbasiewicz, and M. Mąkosza, Tetrahedron, 2004, 60, 347.
64. M. Sulur, P. Sharma, R. Ramakrishnan, R. Naidu, E. Merifield, D. M. Gill, A. M. Clarke, C. Thomson, M. Butters, S. Bachu, C. H. Benison, N. Dokka, E. R. Fong, D. R. J. Hose, G. P. Howell, S. E. Mobberley, S. C. Morton, A. K. Mullen, J. Rapai, and B. Tejas, Org. Proc. Res. Dev., 2012, 16, 1746. CrossRef
65. N. Moskalev and M. Mąkosza, Heterocycles, 2000, 52, 533. CrossRef
66. K. Wojciechowski and M. Mąkosza, Tetrahedron Lett., 1984, 25, 4793. CrossRef
67. G. N. Walker, J. Am. Chem. Soc., 1955, 77, 3844. CrossRef
68. O. Khdour, A. Ouyang, and E. B. Skibo, J. Org. Chem., 2006, 71, 5855. CrossRef
69. M. S. C. Pedras, Q.-A. Zheng, and R. S. Gadagi, Chem. Commun., 2007, 368. CrossRef
70. H. Yamagishi, K. Matsumoto, K. Iwasaki, T. Miyazaki, S. Yokoshima, H. Tokuyama, and T. Fukuyama, Org. Lett., 2008, 10, 2369. CrossRef
71. M. Jeanty, F. Suzenet, and G. Guillaumet, J. Org. Chem., 2008, 73, 7390. CrossRef
72. G. Groszek, M. Bednarski, M. Dybala, and B. Filipek, Eur. J. Med. Chem., 2009, 44, 809. CrossRef
73. K. Yoshikawa, A. Yokomizo, H. Naito, N. Haginoya, S. Kobayashi, T. Yoshino, T. Nagata, A. Mochizuki, K. Osanai, K. Watanabe, H. Kanno, and T. Ohta, Bioorg. Med. Chem., 2009, 17, 8206. CrossRef
74. M. Jeanty, F. Suzenet, P. Delagrange, O. Nosjean, J. A. Boutin, D. H. Caignard, and G. Guillaumet, Bioorg. Med. Chem. Lett., 2011, 21, 2316. CrossRef
75. G. Iakobson, M. Posta, and P. Beier, Synlett, 2013, 24, 855. CrossRef
76. Z. Wróbel and M. Mąkosza, Synlett, 1993, 597. CrossRef
77. Z. Wróbel, Tetrahedron, 1998, 54, 2607. CrossRef
78. S. R. Banini, M. R. Turner, M. M. Cummings, and B. C. G. Söderberg, Tetrahedron, 2011, 67, 3603. CrossRef
79. B. C. G. Söderberg, S. R. Banini, M. R. Turner, A. R. Minter, and A. K. Arrington, Synthesis, 2008, 903. CrossRef
80. Z. Wróbel and M. Mąkosza, Tetrahedron, 1993, 49, 5315. CrossRef
81. R. Bujok, Z. Wróbel, and K. Wojciechowski, Synlett, 2012, 23, 1315. CrossRef
82. K. Wojciechowski and M. Mąkosza, Synthesis, 1986, 651. CrossRef
83. K. Wojciechowski and M. Mąkosza, Bull. Soc. Chim. Belg., 1986, 95, 671. CrossRef
84. M. Mąkosza, T. Glinka, and J. Kinowski, Tetrahedron, 1984, 40, 1863. CrossRef
85. R. C. Bernotas, S. A. Antane, S. E. Lenicek, S. N. Haydar, A. J. Robichaud, B. L. Harrison, G. M. Zhang, D. Smith, J. Coupet, and L. E. Schechter, Bioorg. Med. Chem. Lett., 2009, 19, 6935. CrossRef
86. G. D. Heffernan, R. D. Coghlan, E. S. Manas, R. E. McDevitt, Y. Li, P. E. Mahaney, A. J. Robichaud, C. Huselton, P. Alfinito, J. A. Bray, S. A. Cosmi, G. H. Johnston, T. Kenney, E. Koury, R. C. Winneker, D. C. Deecher, and E. J. Trybulski, Bioorg. Med. Chem., 2009, 17, 7802. CrossRef
87. R. C. Bernotas, S. Antane, R. Shenoy, V.-D. Le, P. Chen, B. L. Harrison, A. J. Robichaud, G. M. Zhang, D. Smith, and L. E. Schechter, Bioorg. Med. Chem. Lett., 2010, 20, 1657. CrossRef
88. A. Korda and Z. Wróbel, Synlett, 2003, 1465. CrossRef
89. M. Platon, R. Amardeil, L. Djakovitch, and J.-C. Hierso, Chem. Soc. Rev., 2012, 41, 3929. CrossRef
90. G. R. Humphrey and J. T. Kuethe, Chem. Rev., 2006, 106, 2875. CrossRef
91. R. Vicente, Org. Biomol. Chem., 2011, 9, 6469. CrossRef
92. D. F. Taber and P. K. Tirunahari, Tetrahedron, 2011, 67, 7195. CrossRef
93. C. E. Garrett and K. Prasad, Adv. Synth. Catal., 2004, 346, 889. CrossRef
94. M. Kawase, Y. Miyake, T. Sakamoto, M. Shimada, and Y. Kikugawa, Tetrahedron, 1989, 45, 1653. CrossRef
95. Z. Wróbel, Synlett, 2004, 1929. CrossRef
96. R. Bujok, A. Kwast, P. Cmoch, and Z. Wróbel, Tetrahedron, 2010, 66, 698. CrossRef
97. M. Bobin, A. Kwast, and Z. Wróbel, Tetrahedron, 2007, 63, 11048. CrossRef
98. M. Rahimizadeh, M. Pordel, M. Bakavoli, H. Eshghi, and A. Shiri, Mendeleev Comm., 2009, 19, 161. CrossRef
99. M. Rahimizadeh, M. Pordel, M. Ranaei, and M. Bakavoli, J. Heterocycl. Chem., 2012, 49, 208. CrossRef
100. R. Bujok, A. Trawczyński, Z. Wróbel, and K. Wojciechowski, Synlett, 2012, 23, 2682. CrossRef
101. A. Trawczyński, R. Bujok, Z. Wróbel, and K. Wojciechowski, Beilstein J. Org. Chem., 2013, 9, 934. CrossRef
102. Z. Wróbel, K. Wojciechowski, A. Kwast, and N. Gajda, Synlett, 2010, 2435. CrossRef
103. A. Cimmino, A. Evidente, V. Mathieu, A. Andolfi, F. Lefranc, A. Kornienko, and R. Kiss, Nat. Prod. Rep., 2012, 29, 487. CrossRef
104. U. Beifuss and M. Tietze, Top. Curr. Chem., 2005, 244, 77.
105. J. B. Laursen and J. Nielsen, Chem. Rev., 2004, 104, 1663. CrossRef
106. R. Bujok, M. Więcław, Z. Wróbel, and K. Wojciechowski, Monatsh. Chem., submitted.
107. M. Królikiewicz, P. Cmoch, and Z. Wróbel, Synlett, 2013, 24, 973. CrossRef