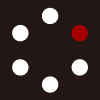
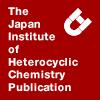
HETEROCYCLES
An International Journal for Reviews and Communications in Heterocyclic ChemistryWeb Edition ISSN: 1881-0942
Published online by The Japan Institute of Heterocyclic Chemistry
e-Journal
Full Text HTML
Received, 21st March, 2013, Accepted, 2nd May, 2013, Published online, 10th May, 2013.
DOI: 10.3987/COM-13-12714
■ Development of a Novel Method for Warfarin Synthesis via Lipase-Catalyzed Steroselective Michael Reaction
Kaoru Sano, Shun-ichi Saito, Yoshihiko Hirose, Yoshihito Kohari, Hiroto Nakano, Chigusa Seki, Michio Tokiwa, Mitsuhiro Takeshita, and Koji Uwai*
Department of Applied Sciences, Graduate School of Engineering, Muroran Institute of Technology, 27-1 Mizumoto, Muroran, Hokkaido 050-8585, Japan
Abstract
Steroselective synthesis of warfarin by promiscuous lipase-catalyzed Michael addition of 4-hydroxycoumarin and benzylideneacetone has been developed. The best result was obtained using lipase AS as a catalyst in anhydrous dimethylsulfoxide (DMSO) with 1:3 molar ratio of 4-hydroxycoumarin to benzylideneacetone at 20ºC for 7 d. The yield and enantiomeric excess were 85% and 45%ee (R-form), respectively.INTRODUCTION
Enzymatic catalytic promiscuity is an enzyme’s ability to catalyze an unexpected reaction. A catalytically promiscuous enzyme will catalyze more than one type of chemical reaction—the natural reaction as well as one or more unexpected reactions. Promiscuous reactions may be non-natural reactions that are useful for synthesis. Many type of enzymatic promiscuous reactions have been reported, especially in the last decade. For example, hydrolases, such as lipases and acylases catalyze the construction of carbon–carbon, carbon–nitrogen, carbon–oxygen, and carbon–sulfur bonds via Michael reaction and Markovnikov additions, 1 and racemases2 and arylmalonate decarboxylase3 catalyze the aldol reaction, etc. Among these reactions, conjugate addition of nucleophiles to α,β-unsaturated carbonyl compounds, i.e., the Michael reaction, is one of the most attractive. Since the first report by Kitazume et al., 1a several groups has reported enzymatic promiscuous Michael reactions. Serine hydrolases are the most commonly used enzymes for biocatalysis. However, only a few of these enzymes afford enantioselectivity. 1i, j
A wide range of 4-hydroxycoumarins are used as pharmaceuticals such as anticoagulants and substances that inhibit HIV or malaria. 4,5 Among the most prominent chiral 4-hydroxycoumarins is warfarin, which works as a vitamin K antagonist and is one of the most effective anticoagulants. Although both enantiomers of warfarin show anticoagulant activity, and warfarin has been in clinical use as a racemate for more than 50 years, the enantiomers have different metabolic pathways, and the activity of the S-form is three to five times stronger than that of the R-form. 6 Furthermore, the enantiomers are metabolized by different drug metabolizing enzymes,7 administering optically pure warfarin is the possibility of eliminating genetic problems and/or drug-drug interactions. Especially, patients expressing certain allelic variants of CYP2C9 which metabolize (S)- but not (R)-warfarin can avoid the risk of bleeding complications if they administer pure (R)-warfarin.8 Previously reported enantioselective syntheses of warfarin were mainly accomplished by transition–metal catalysis9 or organocatalysis, 10 or with the use of a chiral auxiliary;11 however, enantioselectivity of biocatalytic preparation of warfarin has been unsatisfied. 1j In this paper, we present a stereoselective synthesis of warfarin using a lipase–catalyzed Michael reaction.
RESULTS AND DISCUSSION
The synthesis of warfarin was performed by the Michael reaction of 4-hydroxycoumarin to benzylideneacetone (Scheme 1).
First, a series of commercially available lipases were screened as catalysts in MeOH; the results are summarized in Table 1.
As can be seen from Table 1, lipase AS is the best catalyst among the lipases tested for the Michael reaction (20% yield and 5% ee, Table 1, entry 4). Immobilized lipase had moderate activity (7% yield and 4% ee, Table 1, entry 7), whereas the other lipases tested gave low to medium conversions with no stereoselectivity. To confirm whether the reaction was lipase catalyzed, the reaction was conducted with no enzyme; the product was not observed.
The nature of the reaction medium is an important parameter in enzyme-catalyzed reactions, because of its effects on enzyme stability and substrate solubility. Some conventional organic solvents were therefore surveyed. The results indicated that different solvents had significant effects on the activity and enantioselectivity of the lipase AS–catalyzed Michael reaction (Table 2). The best results were
obtained in DMSO and DMF with product yields of 39% and 21%, and enantioselectivities of 29% and 19% ee, respectively (Table 2, entries 1 and 2), after 72 h. In contrast, no enantioselectivity was observed in some of the solvents tested, namely acetone, CHCl3, EtOAc, THF, 1,4-dioxane, Et2O, toluene, n-PrOH (Table 2, entries 3-9, and 16). Moreover, lipase AS did not show any obvious activity in cyclohexane, n-hexane, and H2O (Table 2, entries 10–12). The reason for this seemed to be poor solubilities of the substrates in these solvents.
Since the reaction seemed to give better chemical yields and enantioselectivities in aprotic polar solvents as shown in Table 2, we performed the reaction in various aprotic polar solvents. As expected, the products showed optical activities, but the enantioselectivities were low: 3-17% ee in acetylacetone, acrylonitrile, nitromethane, trichloroacetone, MeCN, DMAC, and NMP (Table 3). DMSO and DMF were therefore used in the following process.
Some enzymes require specific amount of H2O bound to them to maintain enzymatic activity; it is therefore important to confirm the optimal H2O content for the reaction system. The influence of H2O concentration on the lipase AS-catalyzed Michael reaction was therefore investigated. The mixed solvents tested were 90%, 50% and 10% DMSO/H2O. Anhydrous DMSO and DMF were also used to evaluate the effect of H2O (Table 4). The chemical yield and enantioselectivity decreased on addition of H2O (Table 4, entries 1–3). Moreover, when anhydrous solvents were employed, the model reaction gave better yields with product yields of 60% and 27%, and enantioselectivities of 28% and 18% ee, respectively (Table 4, entries 4 and 5) compared with those achieved using the normal grade of the solvent (Table 2, entries 1 and 2). These results indicated that the best solvent for the lipase AS-catalyzed Michael reaction was anhydrous DMSO. These results contradict the report by Xie et al.; 1j they obtained best result in 90% DMSO/H2O. Since lipases are very varied in their amino acid sequences and three-dimensional conformational structure (the molecular weight of the lipases tested in this research were 30–130 KDa), they might have different enzymatic natures, depending on their structures.
To further improve the lipase AS-catalyzed Michael reaction, the effects of the molar ratio of benzylideneacetone to 4-hydroxycoumarin on the reaction were investigated (Table 5). The molar ratio of the substrates had a significant effect on the product yield. An optimal yield of 73% was obtained with a 1:3 molar ratio of 4-hydroxycoumarin to benzylideneacetone at 30 ºC (Table 5, entry 4). Further increasing the number of equivalents of benzylideneacetone gave poorer results than expected (Table 5, entry 5 and 6).
Substrate solubility, enzyme stability, and enzymatic activity are strongly associated with the reaction temperature. The effects of temperature on the lipase AS-catalyzed Michael reaction of 4-hydroxycoumarin to benzylideneacetone were investigated at temperatures ranging from 10 ºC to 60 ºC (Figure 1). Although the yield from the enzymatic reaction was significantly increased by raising the temperature, the enantioselectivity of the product decreased with increasing temperature. Additionally, when we performed the reaction with no enzyme at each temperature, no product was detected. Thus, we estimated that the reaction did not proceed spontaneously but catalyzed by the enzyme. The best ee, 45%, with a low yield of 64% was obtained at 20 ºC, and the best yield, 92%, with only 3% ee, was obtained at 60 ºC, after 72 h. Taking both the activity and stereoselectivity of the enzyme into account, we chose 20 ºC for optimizing the reaction conditions.
We then investigated the time course of lipase AS-catalyzed Michael reaction of 4-hydroxycoumarin to benzylideneacetone (Figure 2). The best yield, 85%, was obtained after 7 days but further increasing the reaction time failed to improve the yield. The ee remained constant during the whole reaction period.
Generically, hydrolases present histidine, aspartic acid and serine in their active site for hydrolysis. Although the amino acid sequence of lipase AS has still not been clarified, we suppose that histidine, aspartic acid and oxyanion hole contribute to the mechanism of this lipase-catalyzed Michael reaction. As shown in Scheme 2, 4-hydroxycoumarin possesses carbonyl-enol tautomers. Negatively charged carboxylate anion of aspartic acid abstract the imidazolic proton of histidine. Then, the imidazolic anion of histidine play as a base to abstract the carbonyl α-proton of 4-hydroxycoumarin. Meanwhile, carbonyl oxygen of benzylideneacetone may be trapped by oxyanion hole and α,β-unsaturated ketone is activated. Then, 4-hydroxycoumarin attacked as a Michael donor to benzylideneacetone, and finally, carbanion abstract a proton of histidine to give warfarin. Stereoselectivity might be introduced by the conformation of lipase which block the Si-face of benzylideneacetone slightly more than Re-face, however, the detailed conformation of lipase AS is still not clear.
CONCLUSION
In summary, we describe the lipase-catalyzed Michael reaction of 4-hydroxycoumarin to benzylideneacetone in anhydrous DMSO. The reaction conditions, including type of lipase, organic solvent, H2O content, molar ratio, temperature, and reaction period, were investigated. Warfarin, one of the most effective anticoagulants, was prepared in one step in good yield (85%) with 45%ee (R-form), using lipase AS as the catalyst, in anhydrous DMSO with a 1:3 molar ratio of 4-hydroxycoumarin to benzylideneacetone, at 20 ºC for 7 days. Although the enantioselectivity was still low, it is still the best result obtained in the enantioselective synthesis of warfarin using a biocatalyst (maximal reported ee: 22%).1j Among the many reported enzyme-catalyzed Michael reactions, only a few have shown enantioselectivity. The asymmetric Michael reaction activity of lipase AS is therefore an important example of enantioselective lipase catalytic promiscuity. Further studies focusing on improvement of the enentioselectivity of the lipase AS-catalyzed transformation are currently under investigation.
EXPERIMENTAL
Materials
Lipase AS (from Aspergillus niger), lipase AYS (from Candida rugosa), lipase PS (from Burkholderia cepacia), and lipase AK (from Pseudomonas fluorescens) were kindly provided by Amano Enzyme Inc (Nagoya, Japan). Lipase PL (from Alcaligenes sp.), lipase QLM (from Alcaligenes sp.), lipase OF (from Candida cylindracea), lipase SL (from Burkholderia cepacia), lipase TL (from Pseudomonas stutzeri), and lipase MY-30 (from Candida cylindracea) were kindly provided by Meito Sangyo Co (Nagoya, Japan). Lipase F-AP15 (from Rhizopus oryzae) was purchased from Wako Pure Chemical Industries Ltd (Osaka, Japan). Immobilized lipase (from Pseudomonas sp.) was purchased from TOYOBO Co (Osaka, Japan). PPL (from porcine pancreas) was purchased from Nacalai Tesque Inc (Kyoto, Japan). 4-Hydroxycoumarin was purchased from Tokyo Chemical Ind. Co. (Tokyo, Japan) and benzylideneacetone was purchased from Kanto Kagaku Reagent Division (Tokyo, Japan). All reactions were monitored by thin-layer chromatography (TLC) using 60-F254 silica gel plates (Merck, Darmstadt, Germany). Column chromatography was carried out using silica gel PSQ 60 (Fuji Silysia Chemical Ltd., Kasugai, Japan). 1H NMR spectra and 13C NMR spectra were recorded at 500 MHz and 125 MHz on a JEOL JNM-ECA (JEOL, Tokyo, Japan) in CDCl3 using tetramethylsilane as an internal standard. ESI-MS analysis of the samples was performed using an LCQ Advantage mass spectrometer (AB SCIEX (MA, USA) API 2000) equipped with an ESI ion source, in positive ionization mode, with data acquisition using Analyst version 1.4.2 software. HPLC analyses were performed using PU-2080 Plus Intelligent HPLC Pump (JASCO, Tokyo, Japan) equipped with a CHIRALPAK AD-H (Daicel Chemical Industries Ltd., Tokyo, Japan) column, and the eluent was monitored using a PU-2075 Plus Intelligent UV/Vis Detector (JASCO, Tokyo, Japan). Chromatographs were recorded using Chromato-Pro PC integrator (Run Time Co, Sagamihara, Japan).
Typical reaction procedure
A mixture of benzylideneacetone (61.4 mg, 0.42 mmol), 4-hydroxycoumarin (22.7 mg, 0.14 mmol), and lipase AS (28.4 mg) in anhydrous DMSO (0.7 mL) was stirred under N2 at 20 ºC for 72 h. The progress of the reaction was monitored by TLC. The enzyme was filtrated off on the Celite pad and H2O was added to the filtrate. The residual solution was extracted with Et2O and the organic layer was dried over anhydrous MgSO4, and concentrated in vacuo. Purification of the residual mixture by column chromatography on silica gel using hexane : EtOAc (85:15, then 70:20) gave (R)-warfarin (22.7 mg, 64% yield, 45% ee) as colorless needles. mp 160-161 ºC. [α]24D +5.00 (c 0.1, MeCN) (lit.,10e [α]25D -10.7 (c 1.0, MeCN) for S-form, 99% ee). 1H NMR (500 MHz, CDCl3) δ: 1.67 (s, 3.00H, CH3, ketal), 1.71 (s, 2.64H, CH3, keto), 2.00 (t, J = 12.3 Hz, 0.50H, CH2, ketal), 2.29 (s, 0.50H, CH2, ketal), 2.39-2.55 (m, 1.23H, CH2, keto), 3.22-3.47 (m, 1.00 H, CH2, ketal), 3.85 (dd, J = 10.0 Hz, 19.4 Hz, 0.53H, CH2, keto), 4.16 (dd, J=6.9 Hz, 11.5 Hz, 0.50H, CH, ketal), 4.28 (dd, J = 3.2 Hz, 6.85 Hz, 0.50H, CH, ketal), 4.70 (d, J = 8.3 Hz, 0.88H, CH, keto), 7.19-7.23 (m, 7.00H, ArH, ketal), 7.27-7.36 (m, 6.16H, ArH, keto), 7.49 (dt, J = 1.7 Hz, 7.8 Hz, 1.0H, ArH, ketal), 7.56 (dt, J = 1.7 Hz, 7.8 Hz, 0.88H, ArH, keto), 7.81 (dd, J = 7.8 Hz, 1.0H, ArH, ketal), 7.89 (dd, J = 1.5 Hz, 8.0 Hz, 1.0H, ArH, ketal), 7.94 (dd, J = 1.4 Hz, 7.6 Hz, 0.9H, ArH, keto). 13C NMR (125 MHz, CDCl3) δ: 27.41, 27.98, 34.79, 35.43, 40.42, 42.83, 50.76, 99.30, 100.78, 101.42, 104.09, 116.08, 116.47, 116.67, 122.89, 123.17, 123.76, 124.06, 126.52, 127.16, 128.41, 128.64, 129.10, 131.63, 132.09, 143.47, 152.87, 159.30, 161.83, 162.45. ESI-MS: C19H16O4H+: [M+H] found 309.4. The enantiomeric excess was determined by HPLC, using CHIRALPAK AD-H column, and eluted with hexane:2-propanol (4:1 v/v) at 1.0 mL/min [tR=5.0 min for (R)-warfarin and tR=11.0 min for (S)-warfarin] monitored at 254 nm.
ACKNOWLEDGEMENTS
Financial support from a MuIT Grant for Selected Research is gratefully acknowledged. We thank Meito Sangyo Co and Amano Enzyme Inc for their generous donation of lipases.
References
1. a) T. Kitazume, T. Ikeya, and K. Murata, J. Chem. Soc., Chem. Commun., 1986, 1331; CrossRef b) O. Torre, I. Alfonso, and V. Gotor, Chem. Commun., 2004, 1724; CrossRef c) M. Svedendahl, K. Hult, and P. Berglund, J. Am. Chem. Soc., 2005, 127, 17988; CrossRef d) P. Carlqvist, M. Svedendahl, C. Branneby, K. Hult, T. Brinck, and P. Berglund, ChemBioChem, 2005, 6, 331; CrossRef e) W. B. Wu, N. Wang, J. M. Xu, Q. Wu, and X. F. Lin, Chem. Commun., 2005, 18, 2348; CrossRef f) W. B. Wu, J. M. Xu, Q. Wu, D. S. Lv, and X. F. Lin, Adv. Synth. Catal., 2006, 348, 487; CrossRef g) J. M. Xu, F. Zhang, B. K. Liu, Q. Wu, and X. F. Lin, Chem. Commun., 2007, 20, 2078; CrossRef h) F. W. Lou, B. K. Liu, Q. Wu, D. S. Lv, and X. F. Lin, Adv. Synth. Catal., 2008, 350, 1959; CrossRef i) J. F. Cai, Z. Guan, and Y. H. He, J. Mol. Cat. B. Enzym., 2011, 68, 240; CrossRef j) B. H. Xie, Z. Guan and Y. H. He, J. Chem. Tech. Biotech., 2012, 87, 1709. CrossRef
2. F. P. Seebeck and D. Hilvert, J. Am. Chem. Soc., 2003, 125, 10158. CrossRef
3. Y. Terao, K. Miyamoto, and H. Ohta, Chem. Lett., 2007, 36, 420. CrossRef
4. L. E. Visser, R. H. N. van Schaik, M. van Vliet, P. H. Trienekens, P. A. G. M. De Smet, A. G. Vulto, A. Hofmann, C. M. van Duijn, and B. H. C. Stricker, Clin. Pharmacol. Ther., 2005, 77, 479. CrossRef
5. M. Ufer, Clin. Pharmacokinet., 2005, 44, 1227. CrossRef
6. D. Marez, M. Legrand, N. Sabbagh, J. M. Lo Guidice, C. Spire, J. J. Lafitte, U. A. Meyer, and F. Broly, Pharmacogenetics, 1997, 7, 193. CrossRef
7. A. E. Rettie, K. R. Korzekwa, K. L. Kunze, R. F. Lawrence, A. C. Eddy, T. Aoyama, H. V. Gelboin, F. J. Gonzalez and W. F. Trager, Chem. Res. Toxicol., 1992, 5, 54. CrossRef
8. H. J. Bardsley and A. K. Daly, PCT Int. Appl. Patent 2000, WO 0043003.
9. a) A. Robinson, and H. Y. Li, Tetrahedron Lett., 1996, 37, 8321; CrossRef b) A. S. Demir, C. Tanyeli, V. Gulbeyaz and H. Akgun, Turk. J. Chem., 1996, 20, 139; c) Y. Tsuchiya, Y. Hamashima, and M. Sodeoka, Org. Lett., 2006, 8, 4851; CrossRef d) S. K. Ray, P. K. Singh, N. Molleti and V. K. Singh, J. Org. Chem., 2012, 77, 8802. CrossRef
10. a) N. Halland, T. Hansen, and K. A. Jørgensen, Angew. Chem. Int. Ed., 2003, 42, 4955; CrossRef b) H. Kim, C. Yen, P. Preston, and J. Chin, Org. Lett., 2006, 8, 5239; CrossRef c) J. W. Xie, L. Yue, W. Chen, W. Du, J. Zhu, J. G. Deng, and Y.C. Chen, Org. Lett., 2007, 9, 413; CrossRef d) Z. Dong, L. Wang, X. Chen, X. Liu, L. Lin, and X. Feng, Eur. J. Org. Chem., 2009, 5192; CrossRef e) M. Rogozinska, A. Adamkiewicz, and J. Mlynarski, Green Chem., 2011, 13, 1155; CrossRef f) J. Dong and D. M. Du, Org. Biomol. Chem., 2012, 10, 8125; CrossRef g) S. Ma, L. Wu, M. Liu, and Y. Wang, Org. Biomol. Chem., 2012, 10, 3721; CrossRef h) Y. Liu, X. Liu, M. Wang, P. He, L. Lin, and X. Feng, J. Org. Chem., 2012, 77, 4136; CrossRef i) M. Leven, J. M. Neudörfl, and B. Goldfuss, Beilstein J. Org. Chem., 2013, 9, 155. CrossRef
11. G. Cravotto, G. M. Nano, G. Palmisano, and S. Tagliapietra, Tetrahedron: Asymmetry, 2001, 12, 707. CrossRef