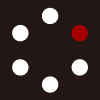
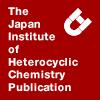
HETEROCYCLES
An International Journal for Reviews and Communications in Heterocyclic ChemistryWeb Edition ISSN: 1881-0942
Published online by The Japan Institute of Heterocyclic Chemistry
e-Journal
Full Text HTML
Received, 8th October, 2013, Accepted, 11th November, 2013, Published online, 22nd November, 2013.
DOI: 10.3987/REV-13-784
■ Chiral Oxazolidine Catalyst for Asymmetric Synthesis
Hiroto Nakano,* Yuko Okuyama, and Eunsang Kwon
Department of Applied Chemistry, Graduate School of Engineering, Muroran Institute of Technology, 27-1 Mizumoto, Muroran, Hokkaido 050-8585, Japan
Abstract
A design of a chiral ligand and its metal-coordinated catalyst is very important for achievement of a highly optical purity and a chemical yield in an asymmetric reaction. Recently, we developed the chiral oxazolidine ligands having N,O acetal structure, such as phosphinooxazolidine (POZ), phosphinooxazinane (POZI) and oxazolidine (OZ). POZ and POZI afforded products in an excellent enantioselectivities in Pd-catalyzed asymmetric allylic alkylation and tandem allylation. Furthermore, cationic Pd-POZ catalysts showed high levels of catalytic activity in the asymmetric Diels-Alder (DA) reactions of some dienes with oxazolidinone or pyrrazolidinone typed dienophiles. Cationic Pd-POZ catalyst showed an excellent catalytic activity in the DA reaction in ionic liquid (IL). The catalyst could be reused eight times without significant decrease of yield and enantioselectivity in the use of an ionic liquid as a solvent. OZ ligand also worked as organocatalyst in the DA reaction of 1,2-dihydropyridies with acroleins to afford the useful intermediate of Tamiflu. The review summarizes these our studies.CONTENTS
1. Introduction
2. Syntheses of POZ and POZI chiral ligands, and their application to Pd-catalyzed reactions
2-1. Syntheses of POZ and POZI
2-2. Asymmetric allylic alkylation
2-3. Tandem asymmetric allylation
3. DA reactions with cationic Pd–POZ
3-1. Synthesis of Pd–POZ
3-2. DA reactions with Pd–POZ
3-3. DA reactions using 1,2-dihydropyridines as dienes
4. Development of polymer-supported ligands and their Pd-complex catalysts: Application to
asymmetric allylic substitution reactions
4-1. Synthesis of polymer-supported POZ
4-2. Application to Pd-catalyzed asymmetric allylic substitution reactions
4-3. Recycling of polymer-supported chiral ligands
5. DA reactions with cationic Pd–POZ chiral catalysts in ionic liquids
5-1. DA reactions in ionic liquids
5-2. Reuse of ionic liquid and chiral catalyst
5-3. DA reactions in ionic liquids with various substrates
6. DA reactions with oxazolidine organocatalysts
6-1. Syntheses of oxazolidine organocatalysts
6-2. Examination of ctivities of chiral catalysts in DA reaction between 1,2-dihydropyridine
and acrolein
6-3. Asymmetric DA reactions with other 1,2-dihydropyridines
6-4. Discussion of reaction mechanism
6-5. Asymmetric DA reactions with multi-substituted acrolein derivatives
7. Conclusion
1. INTRODUCTIONS
Many biologically active compounds, including pharmaceuticals, are optically active. Often, only one of the enantiomers is required because the enantiomers display different activities in vivo. In pharmaceuticals in particular, differences in the absolute configuration can affect not only the pharmacological activity but also the toxicity. Penicillin V, for example, isolated from the penicillium mold, has antibiotic activity; however, its enantiomer has no antibiotic activity. The development of asymmetric syntheses for selectively obtaining one enantiomer is therefore important. Catalytic asymmetric synthesis, in which a minute amount of a chiral molecular catalyst theoretically enables infinite production of an optically active compound, is among the most important current challenges in organic synthetic chemistry; it is also important in terms of energy saving and environmental friendliness.1 We have performed research focused on developing highly active and versatile chiral molecular catalysts. Many types of excellent chiral ligands and their metal complex catalysts, in which the ligands are coordinated to a metal center, have been developed; for example, oxazoline ligands and their metal complexes2 are widely used as chiral catalysts (Figure 1) for reactions such as Diels–Alder (DA), ene, and Mukaiyama aldol reactions, cyclopropanation, and aziridination, because of their relatively easy availability.
Oxazolidine ligands, in which the imine moiety of the oxazoline ring is reduced, can form coordination complexes with various metal atoms, and the complexes are excellent chiral catalysts in some catalytic reactions,3 although there are few reports of their use. Such oxazolidine chiral ligands can be easily derived in a single step from β-amino alcohols and aldehydes. An oxazoline molecule basically has a planar structure, and has one or two chiral centers. In contrast, an oxazolidine molecule has a nonplanar, saturated heterocyclic structure, with up to three chiral centers and, unlike oxazoline ligands, its stereostructure is expected to provide an effective chiral space, affording high enantioselectivity.
We focused on oxazolidine chiral ligands, which can be synthesized in a single step from easily available starting materials. We planned to synthesize phosphinooxazolidine (POZ) 3, which contains a nitrogen atom and a phosphorus atom, enabling bidentate coordination, and also has an N,O-acetal structure, and phosphinooxazinane (POZI) 5, which has a six-membered ring structure.
We also planned to use oxazolidine 58 itself as a chiral organocatalyst. This article describes the development of a series of chiral ligands (Figure 1) and the application of these ligands to asymmetric catalytic reactions.
2. SYNTHESES OF POZ AND POZI CHIRAL LIGANDS, AND THEIR APPLICATION TO
Pd-CATALYZED REACTIONS
2-1. Syntheses of POZ and POZI
We used an easily available cyclic amino alcohol, pyrrolidine methanol 1. Because this compound and its metal complex are effective and widely used chiral catalysts, ligands with a pyrrolidine methanol skeleton were expected to form efficient chiral environments.
We therefore decided to synthesize POZ 3, which has a pyrrolidine skeleton.4 In addition, the synthesis of POZI 5 via the reaction between a xylofuranosamine derivative 4, a saccharide that can be derived from easily available d-xylofuranose, and aldehyde 2 was also attempted.5 The desired products, POZ 3 and POZI 5, were easily obtained by a dehydration condensation reaction between 1 or 4, respectively, and aldehyde 2 (Scheme 1).
2-2. Asymmetric allylic alkylation
The catalytic activities of chiral ligands 3 and 5, synthesized as described in the previous section, were examined in Pd-catalyzed asymmetric allylic alkylation.4,5 The reaction was performed using 1,3-diphenylpropenyl acetate 6 and dimethyl malonate 7 as substrates, and the π-allylpalladium chloride dimer [PdCl(η3-C3H5)]2 as a Pd catalyst under various conditions (Table 1). Both ligands showed good asymmetric catalytic activity and, in particular, 3 gave almost complete reaction and the desired allylated product 8 with high enantioselectivity [98%, 96% enantiomeric excess (ee); entry 1].
2-3. Tandem asymmetric allylation
Many biologically active compounds contain a morpholine or piperazine skeleton, such as the gastroprokinetic agent mosapride (a selective serotonin 5-HT4 receptor agonist), and the anti-HIV (human immunodeficiency virus) agent indinavir (a protease inhibitor) (Scheme 2).6 Despite the importance of developing methods for synthesizing these skeletons with chiral centers, only two examples of particularly effective catalytic asymmetric syntheses have been reported, by Hayashi et al.7 and Achiwa et al.,8 who performed tandem asymmetric allylations. However, these two syntheses did not achieve enantiomeric excesses higher than 90% ee.
We used the ligands that we developed, i.e., 3 and 5, in catalytic asymmetric reactions.5a First, we examined a tandem asymmetric allylation, in which cis-2-butene-1,4-diol diacetate 9 was used as the substrate, N-benzylethanolamine 10 as the nucleophile, [PdCl(η3-C3H5)]2 as the Pd catalyst, and Et3N as the base; the target product was 4-benzyl-2-vinylmorpholine 11 (Table 2). The ligand POZ 3 was not effective in this reaction, but POZI 5d gave the desired product 11 in relatively good chemical yield and with high enantiomeric excess (entries 6 and 7).
Next, tandem asymmetric allylation to give 1,4-dibenzyl-2-vinylpiperazine 13 was examined using N,N'-dibenzylethylenediamine 12 as the nucleophile (Table 3). The reaction conditions were the same as those when amine 10 was used. The reaction with POZI 5d resulted in a moderate chemical yield and relatively good enantiomeric excess (entry 6).
We therefore succeeded in achieving a higher enantiomeric excess than those achieved in past studies of catalytic tandem asymmetric allylation, and obtained 4-benzyl-2-vinylmorpholine 11 and 1,4-dibenzyl-2-vinylpiperazine 13. In particular, our synthesis of 11 was the first to achieve enantioselectivity greater than 90% ee.
3. DA REACTIONS WITH CATIONIC Pd–POZ
3-1. Synthesis of Pd–POZ
Chiral catalysts9 containing metals such as boron, aluminum, titanium, and copper have been developed as chiral Lewis acids for use in catalytic asymmetric DA reactions. As far as studies using a transition metal, i.e., palladium, are concerned, only two examples have been reported, namely, Pd–BINAP [BINAP:2,2′-bis(diphenylphosphino)-1,1′-binaphthyl]10 and Pd–oxazoline catalysts.11 These catalysts were only effective for specific substrates and could not be applied to a wide range of substrates.
We planned to synthesize a Pd complex with the POZ ligand, which was found to be effective for the allylation mentioned above, and to use the complex in an asymmetric DA reaction.12 The reaction between POZ 3 and PdCl2 in dichloromethane at room temperature afforded PdCl2–POZ complex 14 in high yield. However, when the reaction was performed in refluxing 1,2-dichloroethane, the product 16, in which the stereochemistry at the 2-position was inverted, was obtained in high yield. Furthermore, 14 was converted to 16 in refluxing 1,2-dichloroethane. The reactions between complexes 14 and 16 and AgX (X = anion) easily gave the desired active cationic chiral catalysts, Pd–POZ 15 and 17, respectively (Scheme 3).
The structures of complexes 14 and 16 were analyzed using X-ray crystallography. Based on the X-ray crystallographic results as the initial state, molecular orbital (MO) calculations for complexes 14 and 16 were performed. The PM5 method (MOPAC2002) best reproduced the structures. The calculated results for the heat of formation indicate that complex 14, which is effective for enantioselectivity, is less thermally stable than complex 16; this agrees with the detailed structure obtained by X-ray crystallography (Scheme 3). The calculation results for analogous compounds also suggest that catalysts derived from sterically hindered complexes give better enantioselectivities.
3-2. DA reactions with Pd–POZ
The prepared Pd–POZ complexes 15 and 17 were used in asymmetric DA reactions with cyclopentadiene 18 and oxazolidinone dienophile 19 (Table 4).12 Pd–POZ 15a, with SbF6- as the counter ion, afforded the DA adduct 20 in high yield and with high enantioselectivity (entry 1). Furthermore, use of 0.5 mol% of this catalyst gave a high enantioselectivity, 90% ee, although the chemical yield decreased to moderate (entry 7). In contrast, 17, a diastereomer of 15a, did not show any effective catalytic activity (entry 8). We then used Pd–POZ 15a to examine reactions with other dienes and dienophiles. Almost complete enantioselectivity (98% ee) was achieved in the reactions between 18 and 21, and between 18 and 23, with good chemical yields. In addition, the reaction with cyclohexadiene 25 also gave good results.
3-3. DA reactions using 1,2-dihydropyridines as dienes
DA reactions in which 1,2-dihydropyridines are used as the dienes have been used as key reactions for the syntheses of various biologically active compounds. Isoquinuclidine derivatives,13 which have a 2-azabicyclo[2.2.2]octane (isoquinuclidine) skeleton built by the DA reaction involving 1,2-dihydropyridines, are useful synthetic intermediates for the syntheses of, for example, catharanthine,14 which is a precursor of the anticancer agents vinblastine and vincristine, and ibogaine,15 which has attracted attention because of its potential effectiveness against alcohol and drug addiction (Scheme 4).
Furthermore, it has been reported recently that an isoquinuclidine derivative is a useful synthetic intermediate in production of the anti-influenza agent (-)-oseltamivir (Scheme 4).16 The development of catalytic asymmetric DA reactions using 1,2-dihydropyridines is therefore important. We tried using our Pd–POZ chiral catalysts in DA reactions, using 1,2-dihydropyridines as the dienes.17
First, we attempted the reaction between 1-phenoxycarbonyl-1,2-dihydropyridine 27 and dienophile 19 with Pd–POZ 15a; however, a satisfactory enantiomeric excess was not obtained. We therefore focused on pyrazolidinone dienophile 29 to achieve high enantiomeric excess.18 Compared with oxazolidinone dienophile 19, dienophile 29 is expected to form an effective chiral space, giving
high enantiofacial selectivity, as a result of interactions between the chiral catalyst used in the reaction and the achiral substituent, making one enantioface on the N atom of dienophile 29 more favored. We used the successful chiral catalyst 15a to examine DA reactions between diene 27 and pyrazolidinone dienophiles 29 with different substituents on the N atom (Table 5). The reaction using dienophile 29a, which has a benzyl group on the N atom, yielded the desired DA adduct 30a in good chemical yield and with high enantioselectivity (80%, 97% ee), as expected (entry 1).
To clarify the reaction mechanism, semi-empirical MO calculations were carried out, as described in Section 2.1. Two intermediates, A and B, in which two oxygen atoms of two carbonyl groups of the dienophile are coordinated to the Pd atom, were considered as the reaction intermediate. The respective intermediates have six possible isomeric structures because there are two possible orientations of the N atom and three possible orientations of the phenyl group in the dienophile. The most stable isomeric structure is shown in Scheme 5. There is a 6 kcal/mol difference between the heats of formation of the reaction intermediate complexes A and B; A is the preferred structure. In the case of attack from the re face, the olefin moiety of the coordinated dienophile would be blocked by the benzyl group. Diene 27 therefore seems to attack the intermediate from the less crowded si face, to yield the DA adduct 30a with high enantiomeric excess.
We used the chiral catalyst Pd–POZ 15a in the DA reaction between 1,2-dihydropyridine and a pyrazolidinone dienophile, and succeeded for the first time in synthesizing the desired optically active isoquinuclidine compounds with previously unreported, practical, high enantiomeric excesses.
4. DEVELOPMENT OF POLYMER-SUPPORTED LIGANDS AND THEIR Pd-COMPLEX CATALYSTS: APPLICATION TO ASYMMETRIC ALLYLIC SUBSTITUTION REACTIONS
4-1. Synthesis of polymer-supported POZ
With the aim of developing recoverable and reusable POZ, we tried to make polymer-supported POZ. Ligand 32, which has a hydroxy group for supporting it on a polymer, was synthesized by a dehydration condensation reaction between aldehyde 31 and amino
alcohol 1 (Scheme 6). The reactions between 32 and polymers 33 (PS-DES-Cl),19 34 (PS-Et-COOH),20 and 35 (TentaGel MB-COOH)21 were carried out in dichloromethane in the presence of imidazole or diisopropylcarbodiimide at room temperature, to yield the corresponding desired products 36a–c (Scheme 7).
4-2. Application to Pd-catalyzed asymmetric allylic substitution reactions
The synthesized ligands 36a–c were used in Pd-catalyzed asymmetric allylic substitution reactions, and their catalytic activities were examined (Table 6).22 First, the polystyrene-supported POZ ligand 36b was used in the allylic alkylation of 1,3-diphenylpropenyl acetate 6 with dimethyl malonate 7. The desired product 8 was obtained with almost 100% chemical yield and enantioselectivity (99%, 99% ee; entry 3).
4-3. Recycling of polymer-supported chiral ligands
Reuse of the most successful ligand, 36b, was attempted in the same reaction (Table 7).22 To recycle the ligand, the reaction substrates and products were separated from the polymer ligand by removing the dichloromethane phase in which the reaction substrates and products were dissolved, using a syringe. A new palladium source was not added to the reaction mixture. The polymer ligand was reusable but its catalytic activity gradually declined; the enantioselectivity declined greatly in the third cycle.
5. DA REACTIONS WITH CATIONIC PD–POZ CHIRAL CATALYSTS IN IONIC LIQUIDS
5-1. DA reactions in ionic liquids
The synthesis of polymer-supported chiral ligands was problematic and it was difficult to maintain high catalytic activity of the chiral ligands in recycling experiments. We therefore changed the focus of our research from modification of ligands and catalysts to examination of reaction media for asymmetric catalytic reactions. We performed DA reactions with chiral catalysts in ionic liquids,23
which have attracted attention as new reaction media, and tried to recover and reuse the catalysts in ionic liquids (Table 8).24 Complex 15a, which showed the best catalytic activity in the reaction in dichloromethane, was used as the catalyst, and imidazolium salts 37a–g, which are easily available and widely used in such reactions, were used as ionic liquids. The use of ionic liquid 37d, which has BF4- as the counter ion, as the solvent, gave the best chemical yield and enantioselectivity of DA adduct 20 (89%, 96% ee; entry 4). The enantioselectivity of the reaction in an ionic liquid was better than that in dichloromethane at room temperature, and was comparable to that in dichloromethane at -50 °C (Table 4). The reaction in an ionic liquid can afford a high chemical yield and enantioselectivity without control of the reaction temperature.
5-2. Reuse of ionic liquid and chiral catalyst
Reuse of the most successful Pd–POZ catalyst, 15a, in ionic liquid 37d was examined (Table 9).24 Ionic liquid 37d and catalyst 15a (5 mol%) were used repeatedly in the reaction at room temperature for 48 h in an argon atmosphere. Although the chemical yields and enantioselectivities were good in the first and second cycles (89–90%, 85–93% ee), they decreased significantly in the third cycle (69%, 65% ee). It is assumed that the reason for the decrease is deactivation of the catalyst by oxygen entering the system during recycling.
To prevent deactivation of the catalyst by oxygen and to obtain the products with higher enantioselectivities, the asymmetric DA reaction between diene 18 and dienophile 19 in a mixed solvent containing ionic liquid 37d and dichloromethane was examined (Table 10). Ionic liquid 37d and catalyst 15a were efficiently recovered and reused; the chemical yield and enantioselectivity of the DA adduct 20 remained high until the seventh cycle (89–99%, 94–99% ee) and the chemical yield and enantioselectivity were 89% and
88% ee, respectively, in the eighth cycle. Although the chemical yield and enantioselectivity decreased in the ninth cycle because of deactivation of the catalyst (57%, 75% ee), this method delayed deactivation of the catalyst, and Pd–POZ catalyst 15a could be used eight times.
These results demonstrated that a mixture of ionic liquid 37d and dichloromethane was the best reaction medium. The obtained enantioselectivity (99% ee) was the highest reported for an asymmetric DA reaction in an ionic liquid, and the number of times the chiral catalyst could be recycled was also the highest (eight times).
5-3. DA reactions in ionic liquids with various substrates
Asymmetric DA reactions between other dienes and dienophiles under the same conditions (in the mixed solvent) were also examined (Table 11).24 The reaction between diene 18 and dienophile 21 yielded the DA adduct 22 in moderate chemical yield with an enantioselectivity of 98% ee (entry 1). The reactions between 18 and 38, and between 25 and 19, also gave relatively high enantioselectivities (entries 2 and 3). Reactions using furan 40 or 1,2-dihydropyridine 27 as the diene were also examined. The expected DA adducts 41 and 28 are useful synthetic intermediates of biologically active compounds, so development of methods
affording high chemical yields and enantioselectivities is important. In the reaction with furan 40, a satisfactory regioselectivity and chemical yield of the desired product 41 were not obtained; however, the endo adduct 41a was obtained with 85% ee, and the exo adduct 41b was obtained with almost complete enantioselectivity (98% ee; entry 4). In contrast, the reaction between 1,2-dihydropyridine 27 and 19 afforded a chemical yield of 80% and a high enantioselectivity of 96% ee (entry 5). In Section 2.3, it was shown that to obtain the highest enantioselectivity in the asymmetric DA reaction with 1,2-dihydropyridine 27, the reaction has to involve the pyrazolidinone dienophile 29a, which was prepared separately from the Pd–POZ catalyst (Table 5).17 However, it was demonstrated that by using a mixed solvent containing an ionic liquid as the reaction medium, high enantioselectivity can be achieved in the reaction using the easily available oxazolidinone dienophile.
6. DA REACTIONS WITH OXAZOLIDINE ORGANOCATALYSTS
6-1. Syntheses of oxazolidine organocatalysts
Environmental effects now have to be considered in chemical syntheses, and environmentally benign organic syntheses have been receiving attention.25 The chiral catalysts used in catalytic asymmetric syntheses can be divided into two categories: organometallic catalysts and metal-free chiral organocatalysts. Organometallic catalysts are highly active but unstable to water and oxygen, and the metals have the disadvantages of being expensive, toxic, and difficult to dispose of. In contrast, organocatalysts are stable in air, easy to handle, and inexpensive, so they are being focused on as next-generation, environmentally friendly catalysts.
The mechanisms of the actions of chiral organocatalysts are broadly divided into two types: noncovalent and covalent. 26 Chiral organocatalysts with a noncovalent mechanism attach to the substrate by hydrogen bonding and activate it by acting as a Lewis acid. Representative examples of such organocatalysts are chiral phosphoric acids such as the chiral Brønsted acid catalysts reported by Terada et al.27 and Akiyama et al.28 Chiral organocatalysts with a covalent mechanism immobilize the substrate on the catalyst by forming iminium or enamine species with the substrate, and activate the substrate. The catalyst promotes the reaction by controlling enantioselective attack of a nucleophile on the immobilized substrate. Examples of such catalysts include the imidazolidinone catalysts29 reported by MacMillan et al. and the siloxyproline catalysts30 reported by both Hayashi et al. and Jorgensen et al., and they can be used in a wide range of reactions such as cycloadditions, intramolecular Michael reactions, and Friedel–Crafts reactions. The POZ chiral ligand 3, which we developed, is based on an oxazolidine ring that can provide a chiral space, affording the product with high enantioselectivity (Sections 1 to 4). Accordingly, we decided to develop oxazolidine chiral organocatalysts A, based on an oxazolidine ring, and use them in DA reactions with 1,2-dihydropyridine derivatives as the dienes.31 These oxazolidine organocatalysts are expected to afford high enantioselectivity because enantioselective attack of the diene on an activated iminium salt intermediate formed by reaction between the catalyst and the dienophile is effectively controlled by the stereochemistries of the substituents in the 2- and 4-positions of the catalysts (Scheme 8).
To date, there has been only one report of a catalytic asymmetric DA reaction of 1,2-dihydropyridine derivatives using chiral organocatalysts, namely the key reaction in the total synthesis of (-)-oseltamivir by Fukuyama et al.,16 mentioned earlier.
In their study, an asymmetric DA reaction between 1-benzyloxycarbonyl-1,2-dihydropyridine 43 and acrolein 44 with MacMillan catalyst 42 was performed; 45a, a synthetic intermediate of (-)-oseltamivir, was obtained with high enantioselectivity (>99% ee); however, the chemical yield was unsatisfactory and the reaction needs to be improved to make it suitable for practical applications (Scheme 9).
The β-amino alcohols used for the synthesis of the oxazolidine catalysts were derived from amino acids with easily available pure enantiomers. A geminal diphenyl group was introduced at the 1-position of the β-amino alcohol to inhibit dissociation of the formed oxazolidine ring and keep it stable. Benzaldehyde, which affords 2-monosubstituted oxazolidine compounds, and acetone, which affords 2-disubstituted bulky compounds, were selected as the carbonyl compounds. To enhance turnover of the catalysts, i.e., to promote formation and dissociation of iminium salts, the synthesized oxazolidine compounds were used as the corresponding salts, obtained by adding acids. Haloacetic acids, especially trifluoroacetic acid, which is an organic acid commonly used for organocatalysts, and hydrochloric acid, which is a representative inorganic acid, were selected.
First, Grignard reactions of the easily available amino acid methyl ester hydrochlorides 46–49 were carried out to obtain the corresponding diphenyl amino alcohols 50–53.32 Dehydration condensation of the diphenyl amino alcohols and benzaldehyde
yielded the products 54–57 quantitatively. The products were in equilibrium between oxazolidines and imines; the ratios of the two states were determined based on their 1H-NMR spectra (CDCl3). The equilibrium mixtures 54–57 were shifted to the respective single oxazolidine compounds 58–61 by adding the corresponding acids. However, 56 and 57 yielded mixtures of the corresponding oxazolidine salts 60 and 61 and their decomposition products, amino alcohol salts or iminium salts, on reaction with organic acids. The precursors of 64 and 65, which have a geminal dimethyl group at the 2-position of the oxazolidine ring, were obtained in good chemical yields by dehydration condensation between acetone and the amino alcohols 50 and 51, without formation of undesired imines.33 The addition of trifluoroacetic acid to the obtained precursors 62 and 63 yielded the corresponding desired products 64 and 65 quantitatively (Scheme 10). The absolute configurations at the 2-positions of 58–61 were determined based on NOE difference spectra of the 2- and 4-positions, obtained by 1H-NMR spectroscopy.
6-2. Examination of activities of chiral catalysts in DA reaction between 1,2-dihydropyridine and acrolein
We examined the catalytic activities of chiral oxazolidine organocatalysts 58, 59, 64, and 65, obtained as described in the previous section, in the DA reaction; 1-phenoxycarbonyl-1,2-dihydropyridine 27 was used as the diene and acrolein 44 was used as the dienophile.
Based on the results reported by Fukuyama et al.,16 we performed the reaction using 2 equiv of diene 27 and 10 mol% of the chiral catalysts 58, 59, 64, and 65, in a mixed MeCN–H2O (19:1) solvent at 0 °C for 24 h. To calculate the chemical yields and enantioselectivities, the obtained adducts 66 were converted to the corresponding alcohols 67 by reduction with NaBH4 (Table 12).
Trifluoroacetate 58a, which has a phenyl group at the 2-position and a bulky tert-butyl group at the 4-position of the oxazolidine ring of the catalyst, afforded the desired endo DA adduct (7S)-66a with almost complete enantioselectivity (71%, >99% ee; entry 1). Trifluoroacetate 59, which has an isopropyl group at the 4-position of the oxazolidine ring, gave the corresponding DA adduct in good chemical yield with high enantioselectivity (70%, 97% ee; entry 2).
Next, DA reactions with 64 and 65, which have a geminal dimethyl group at the 2-position of the oxazolidine ring, were performed under the same conditions as those for the reaction with catalyst 58a (entries 7 and 8). However, the chemical yield and enantioselectivity both declined in the reactions with either 64 or 65. The reason why the chemical yields and enantioselectivities vary greatly, depending on the catalysts used, i.e., 58a, which has a phenyl group at the 2-position of the oxazolidine ring, or 64 and 65, which have a geminal dimethyl group at the 2-position, is considered to be as follows. As shown in Scheme 11 (a), in the case of 58a, which has a phenyl group at the 2-position, the substituents at the 2- and 4-positions are situated in the cis position, and the diene attacks the less sterically hindered si face, giving high enantioselectivity. In the cases of 64 and 65, which have a geminal dimethyl group at the 2-position, a methyl group is present on the si face, which sterically hinders attack by the diene, so good chemical yields and enantioselectivities are not obtained, as shown in Scheme 11 (b).
6-3. Asymmetric DA reactions with other 1,2-dihydropyridines
We used our chiral oxazolidine organocatalysts in DA reactions using other 1,2-dihydropyridine derivatives as the dienes. The most successful catalyst in the reactions described in the previous section, i.e., 58a, was used in DA reactions with 1-benzyloxycarbonyl-1,2-dihydropyridine 43 or 1-tert-butoxycarbonyl-1,2-dihydropyridine 68 as the diene, performed under the same conditions as those used for the reaction with diene 27 (Table 13). The reaction with diene 43 successfully afforded the desired adduct 45a in high chemical yield (90%), with almost complete enantioselectivity (>99% ee; entry 1). The reaction with diene 68 afforded the desired adduct 69 in
moderate yield (51%) and with almost complete enantioselectivity (>99% ee; entry 5).
6-4. Discussion of reaction mechanism
The DA reaction between diene 27 and acrolein in the presence of catalyst 58a gave enantioselectivity of more than 99% ee, as described in Section 5.2. This demonstrated that substituents at the 2- and 4-positions of the oxazolidine skeleton of the catalyst greatly contribute to high enantioselectivity. To clarify the reaction mechanism, we performed MO calculations on the iminium salts generated as intermediates and the dienes used in the reaction. Calculation of the total energy of the molecules after conformational analysis revealed that the iminium salt A is more stable by approximately 0.1 kcal/mol than salt B (Scheme 12). Conformational analysis of diene 27 indicated that conformation b is more stable than conformation a because the methylene moiety and the carbonyl oxygen are further apart.
The results showed that when diene b, which has a stable conformation, attacks the intermediate iminium salt A, which has a stable conformation, the diene has two possible routes, (i) and (ii), to the reactive site of the iminium salt (Scheme 12). In case (ii), attack from both the si and re faces of the olefin is difficult because of steric repulsion between the diene and the tert-butyl group at the 4-position or the phenyl group at the 5-position of the oxazolidine ring. In contrast, in case (i), although attack from the re face is difficult because of steric repulsion with the phenyl group at the 2-position and the tert-butyl group at the 4-position of the oxazolidine ring, attack from the less sterically hindered si face seems to be easy. These results suggest that in the DA reaction with diene 27, 27 approaches the intermediate iminium salt A (i) from the si face to yield the (7S)-DA adduct 66a. It should be noted that in this possible reaction mechanism, the influence of the counter anions of the catalysts was not taken into account because the action mechanism is unclear.
6-5. Asymmetric DA reactions with multi-substituted acrolein derivatives
With the aim of extending the substrate applicability of the catalyst, we examined the activities of the chiral catalysts by performing asymmetric DA reactions with a dienophilic multi-substituted acrolein derivative, fumaraldehydic acid methyl ester 72. Rawal et al. reported the first example of an asymmetric DA reaction between a 1,2-dihydropyridine derivative and a multi-substituted acrolein, i.e., the DA reaction between 27 and methacrolein with chiral organometallic catalysts (salen complexes); the product was not obtained with good enantioselectivity.34 First, we performed the DA reaction with diene 27. The desired adduct 73 was obtained in good chemical yield (68%) and with almost complete enantioselectivity (>99%). The reaction with diene 43 also successfully afforded the desired adduct 74 in good chemical yield (83%) and with almost complete enantioselectivity (>99%; Scheme 13). The absolute
stereochemistry of DA adduct 73 was determined by the X-ray analysis of Br-lactone 77 converted from 73. On the other hand, the absolute stereochemistry of DA adduct 74 was determined by the chemical conversion. Thus, alcohol 76 was converted to 75 determined the absolute stereochemistry by X-ray anaylsis of 77.
These results revealed that the chiral oxazolidine organocatalyst 58a is an excellent chiral catalyst, not only in asymmetric DA reactions with acrolein, but also in reactions with multi-substituted acrolein derivatives. This suggests that the chiral catalyst 58a would also have excellent catalytic activity in reactions with various multi-substituted acrolein derivatives; it is expected that isoquinuclidine derivatives with substituents introduced at the desired ring positions could be synthesized by performing asymmetric DA reactions with this catalyst.
7. CONCLUSION
This article described the syntheses of our POZ and POZI chiral ligands, their Pd-complex catalysts, and oxazolidine chiral organocatalysts, and their usefulness in asymmetric catalytic reactions. We found that these chiral ligands and catalysts show excellent catalytic activities in the reactions we investigated. These catalysts can be synthesized easily, and we intend to use them in other asymmetric catalytic reactions to find further applications.
ACKNOWLEDGEMENTS
We would like to acknowledge the contributions of many co-workers and their efforts in helping to obtain the results we described here. Dr Chizuko Kabuto (Tohoku University) who was co-worker had passed away on 19 August, 2013 at the age of 69. We shall always remember her, her gentle smile and her excellent contribution to chemistry. We shall pray for the repose of her.
References
1. “Comprehensive Asymmetric Catalysis” ed. by E. J. Jacobsen, A. Pfaltz, and H. Yamamoto, Springer, New York, 1999.
2. (a) A. K. Ghosh, P. Mathivanan, and J. Cappiello, Tetrahedron Lett., 1996, 37, 3815; CrossRef (b) D. A. Evans, S. J. Miller, T. Lectka, and P. V. Matt, J. Am. Chem. Soc., 1999, 121, 7559; CrossRef (c) D. A. Evans, D. M. Barnes, J. S. Johnson, T. Lectka, P. V. Matt, S. J. Miller, J. A. Murry, R. D. Norcross, E. A. Shaughnessy, and K. R. Campos, J. Am. Chem. Soc., 1999, 121, 7582. CrossRef
3. (a) M. Falorni, C. Collu, S. Conti, and G. Giacomelli, Tetrahedron: Asymmetry, 1996, 7, 293; CrossRef (b) M.-J. Jin, J.-A. Jung, and S.-H. Kim, Tetrahedron Lett., 1999, 40, 5197; CrossRef (c) C. Augier, V. Malara, V. Lazzeri, and B. Waegell, Tetrahedron Lett., 1995, 36, 8775. CrossRef
4. Y. Okuyama, H. Nakano, and H. Hongo, Tetrahedron: Asymmetry, 2000, 11, 1193. CrossRef
5. (a) H. Nakano, J. Yokoyama, R. Fujita, and H. Hongo, Tetrahedron Lett., 2002, 43, 7761; CrossRef (b) H. Nakano, J. Yokoyama, Y. Okuyama, R. Fujita, and H. Hongo, Tetrahedron: Asymmetry, 2003, 14, 236. CrossRef
6. (a) T. Morie, S. Kato, H. Harada, N. Yoshida, and J. Matsumoto, Chem. Pharm. Bull., 1994, 42, 877; CrossRef (b) N. Sakurai, F. Hirayama, T. Kuroda, S. Uemori, A. Moriguchi, K. Yamamoto, Y. Ikeda, and T. Kawakita, Bioorg. Med. Chem. Lett., 1998, 8, 2185; CrossRef (c) S. Berg, L.-G. Larsson, L. Renyi, S. B. Ross, and S.-O. Thorberg, J. Med. Chem., 1998, 41, 1934; CrossRef (d) B. D. Dorsey, C. McDonough, S. L. McDaniel, R. B. L. Levin, C. L. Newton, J. M. Hoffman, P. L. Dark, J. A. Zugay-Murphy, E. A. Emilio, W. A. Schleif, D. B. Olsen, M. W. Stahlhut, C. A. Rutkowski, L. C. Kuo, J. H. Lin, I.-W. Chen, S. R. Michelson, M. K. Holloway, J. R. Huff, and J. P. Vacca, J. Med. Chem., 2000, 43, 3386. CrossRef
7. Y. Uozumi, A. Tanahashi, and T. Hayashi, J. Org. Chem., 1993, 58, 6826. CrossRef
8. A.Yamazaki and K. Achiwa, Tetrahedron: Asymmetry, 1995, 6, 1021. CrossRef
9. (a) E. J. Corey and T. P. Loh, J. Am. Chem. Soc., 1991, 113, 8966; CrossRef (b) J. Bao, W. D. Wulff, and A. L. Rheingold, J. Am. Chem. Soc., 1993, 115, 3814; CrossRef (c) K. Narasaka, N. Iwasawa, M. Inoue, T. Yamada, M. Nakasima, and J. Sugimori, J. Am. Chem. Soc., 1989, 111, 5340; CrossRef (d) D. A. Evans, S. J. Miller, T. Lectka, and P. Matt, J. Am. Chem. Soc., 1999, 121, 7559. CrossRef
10. (a) S. Oi, K. Kashiwagi, and Y. Inoue, Tetrahedron Lett., 1998, 39, 6253; CrossRef (b) A. K. Ghosh and H. Matsuda, Org. Lett., 1999, 1, 2157. CrossRef
11. K. Hiroi and K. Watanabe, Tetrahedron: Asymmetry, 2002, 13, 1841. CrossRef
12. (a) H. Nakano, Y. Okuyama, Y. Suzuki, R. Fujita, and C. Kabuto, Chem. Commun., 2002, 1146; CrossRef (b) H. Nakano, K. Takahashi, Y. Okuyama, C. Senoo, N. Tugawa, Y. Suzuki, R. Fujita, K. Sasaki, and C. Kabuto, J. Org. Chem., 2004, 69, 7092; CrossRef (c) H. Nakano, Y. Suzuki, C. Kabuto, R. Fujita, and H. Hongo, J. Org. Chem., 2002, 67, 5011. CrossRef
13. S. D. Glick, I. M. Maisonneuve, and K. K. Szumlinski, “The Alkaloids” Vol. 56, ed. by K. R. Alper, S. D. Glick, and G. A. Cordell, Academic Press, San Diego, 2001.
14. C. Marazano, M. T. Goff M, J. L. Fourrey J, and B. C. Das, J. Chem. Soc., Chem. Commun., 1981, 389. CrossRef
15. D. Y. He, N. N. McGough, A. Ravindranathan, J. Jeanblanc, M. L. Logrip, K. Phamluong, P. H. Janak, and D. J. Pon, Neurosci., 2005, 25, 619. CrossRef
16. N. Satoh, T. Akiba, S. Yokoshima, and T. Fukuyama, Angew. Chem. Int. Ed., 2007, 46, 5734. CrossRef
17. (a) H. Nakano, N. Tsugawa, and R. Fujita, Tetrahedron Lett., 2005, 46, 5677; CrossRef (b) H. Nakano, N. Tsugawa, K. Takahashi, Y. Okuyama, and R. Fujita, Tetrahedron, 2006, 62, 10879.. CrossRef
18. M. P. Sibi, L. Lakshmanan, M. Lel, and C. P. Jasperse, J. Am. Chem. Soc., 2001, 123, 8444. CrossRef
19. Y. Hu, J. A. Porco, Jr., J. W. Labadie, O. W. Gooding, and B. M. Trost, J. Org. Chem., 1998, 63, 4518. CrossRef
20. J. S. Panek and B. Zhu, Tetrahedron Lett., 1996, 37, 8151. CrossRef
21. K. Hallman, E. Macedo, K. Nordstrom, and C. Moberg, Tetrahedron: Asymmetry, 1999, 10, 4037. CrossRef
22. (a) H. Nakano, K. Takahashi, and R. Fujita, Heterocycles, 2004, 64, 407; CrossRef (b) H. Nakano, K. Takahashi, Y. Suzuki, and R. Fujita, Tetrahedron: Asymmetry, 2005, 16, 609; CrossRef (c) H. Nakano, K. Takahashi, and R. Fujita, Tetrahedron: Asymmetry, 2005, 16, 2133. CrossRef
23. (a) “Ionic Liquids-Industrial Applications for Green Chemistry” ed. by R. D. Roger and K. R. Seddon, ACS Symposium Series 818, American Chemical Society, 2002; (b) “Ionic Liquids in Synthesis” ed. by P. Wasserscheid and T. Welton, Wiley-VCH, Weinheim, 2003; (c) “Electrochemical Aspect of Ionic Liquids” ed. by H. Ohno, John Wiley and Sons, New York, 2005. CrossRef
24. K. Takahashi, H. Nakano, and R. Fujita, Chem. Commun., 2007, 263. CrossRef
25. B. M. Trost, Angew. Chem., Int. Ed. Engl., 1995, 34, 259. CrossRef
26. A. Berkessel and H. Groger, Asymmerric Organocatalysis, Wiley-VCH, Weinheim, 2005. CrossRef
27. D. Uraguchi and M. Terada, J. Am. Chem. Soc., 2004, 126, 5356. CrossRef
28. T. Akiyama, J. Itoh, K. Yokota, and K. Fuchibe, Angew. Chem., Int. Ed. Engl., 2004, 43, 1566. CrossRef
29. G. Lelais and D. W. C. MacMillan, Aldrichimica Acta, 2006, 39, 79.
30. (a) Y. Hayashi, T. Sumiya, J. Takahashi, H. Gotoh, T. Urushima, and M. Shoji, Angew. Chem. Int. Ed., 2008, 45, 958; CrossRef (b) M. Marigo, T. C. Wabnitz, D. Fielenbach, and K. A. Jorgensen, Angew. Chem. Int. Ed., 2005, 44, 794. CrossRef
31. H. Nakano, K. Osone, M. Takeshita, E. Kwon, C. Seki, H. Matsuyama, N. Takano, and Y. Kohari, Chem. Commun., 2010, 46, 4827. CrossRef
32. C. Da, Z. Han, M. Ni, F. Yang, D. Liu, Y. Zhou, and R. Wang, Tetrahedron: Asymmetry, 2003, 14, 659. CrossRef
33. (a) Z. Xu, J. Mao, and Y. Zhang, Org. Biomol. Chem., 2008, 6, 1288; CrossRef (b) Y. Kang, R. Wang, L. Liu, C. Da, W. Yan, and Z. Xu, Tetrahedron Lett., 2005, 46, 863. CrossRef
34. N. Takenaka, Y. Huang, and V. H. Rawal, Tetrahedron, 2002, 58, 8299. CrossRef