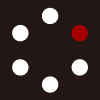
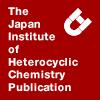
HETEROCYCLES
An International Journal for Reviews and Communications in Heterocyclic ChemistryWeb Edition ISSN: 1881-0942
Published online by The Japan Institute of Heterocyclic Chemistry
e-Journal
Full Text HTML
Received, 25th March, 2014, Accepted, 18th April, 2014, Published online, 21st April, 2014.
■ Photocyclization Reactions of N-Substituted 3-(2-Hydroxynaphthalen-1-yl)propenamide Derivatives Accompanied by Liberation of Aliphatic and Aromatic Primary Amines
Koh Watanabe, Shun Takahashi, Takuya Yoshizawa, Tetsutaro Igarashi, and Tadamitsu Sakurai*
Department of Material and Life Chemistry, Faculty of Engineering, Kanagawa University, 3-27-1 Rokkakubashi, Kanagawa-ku, Yokohama 221-8686, Japan
Abstract
(E)-3-(2-Hydroxynaphthalen-1-yl)propenoyl- and (E)-3-(2-hydroxynaphthalen-1-yl)-2-methylpropenoyl-protected aliphatic and aromatic primary amines were synthesized and their photolytic behavior in methanol was explored. The results showed that irradiation of the hydroxynaphthyl-propenamide derivatives in a protic polar solvent at wavelengths greater than 280 nm or 340 nm causes rather efficient deprotection reactions to afford the desired amines quantitatively along with fluorescent benzocoumarins.Photochemistry has contributed to the development of methodologies for the chemoselective release of photofunctional chromophores from organic compounds that are protected with various functional groups.1 Since light can be easily manipulated, achieving a spatiotemporal release of the protective groups even at low temperatures is not difficult. Many photoremovable protecting groups have been developed and applied to functional group transformations of organic compounds.1 However, significant attention has been focused on photocleavable protecting groups for primary amines owing to the biochemical significance of this functional group.2 We previously reported that the naphthylmethyleneaminooxycarbonyl chromophore (attached to the amino nitrogen of a primary amine) is removed photochemically without any difficulty; therefore, it functions as a novel photoremovable protecting group for primary amines.3 Moreover, this photofunctional protective group could be applied to various primary amines including α-amino acids. For example, when protected α-amino acid derivatives were irradiated at wavelengths greater than 280 nm (λ ≥ 280 nm), they efficiently decomposed to regenerate the corresponding α-amino acids in high yields.3
Thus, if protection of a functional group in a bioactive compound by a photocleavable chromophore could temporarily deactivate this compound, then such a protected bioactive compound is expected to serve as a so-called “caged compound.”4 Numerous caged compounds, such as photoremovable arylmethyl- and aroylmethyl-type chromophore-substituted carboxylic acids,5 amino acids,6 peptides,7 thiols,8 and nucleotides,9 have been reported in the literature. Most photofunctional caged compounds have been synthesized by protecting the carboxyl groups in bioactive molecules with the aforementioned photoremovable chromophores.5,6 In contrast, only a few caged compounds have been reported, in which these chromophores are attached to the primary amino nitrogen.9c Thus, to broaden the scope of application of these compounds it is imperative to develop photofunctional caged compounds by derivatization of the amino group in bioactive molecules.
Recently, 2-hydroxycinnamoyl and 2-aminocinnamoyl chromophores were shown to function as photoremovable protecting groups for primary alcohols and amines and related compounds.10a–e Note that excitation of (E)-2-hydroxy- and (E)-2-amino-cinnamic acid esters readily leads to isomerization to the (Z)-isomers, which subsequently cyclize to the fluorescent lactone and lactam rings, respectively, with expulsion of the corresponding alcohols. Although efficient photodeprotection of the cinnamic acid amide derivatives occurs under acidic solution and solid conditions,10b,e these derivatives only undergo photoisomerization reactions under neutral solution conditions to release negligible amounts of primary amines.10b In contrast, when we performed a systematic study on the photoinduced electron transfer-initiated cyclization reaction of N-acyl-α-dehydroarylalaninamides, we determined that the introduction of naphthalene rather than benzene ring as the aryl chromophore in these arylalaninamide derivatives significantly enhances their photoreactivity. In addition, in the excited singlet state 2-naphthol has a much higher acidity than phenol; thus, the 3-(2-hydroxynaphthalen-1-yl)propenoyl chromophore is a more attractive photolabile protecting group for primary amines compared to the 2-hydroxycinnamoyl chromophore.11
Based on the abovementioned guidelines for the molecular design of the protecting group, we designed the 3-(2-hydroxynaphthalen-1-yl)propenoyl and 3-(2-hydroxynaphthalen-1-yl)-2-methylpropenoyl chromophores as photocleavable protecting groups to enable the efficient release of a primary amine and a fluorescent coumarin derivative. In the present study, 4-methoxybenzylamine [(E)-1a], 4-cyanobenzylamine [(E)-1b], (S)-phenylalanine methyl ester [(E)-1c], and 4-methoxyaniline [(E)-1d] protected by these chromophores were synthesized and their photolytic behavior in methanol was investigated (Scheme 1).
As shown in Scheme 1, 2-hydroxy-1-naphthaldehyde was reacted with (carbethoxymethylene)-triphenylphosphorane (2a) and (carbethoxyethylidene)triphenylphosphorane (2b) in toluene to afford ethyl (E)-3-(2-hydroxynaphthalen-1-yl)propenoate [(E)-3a] and ethyl (E)-3-(2-hydroxynaphthalen-1-yl)-2-methylpropenoate [(E)-3b], respectively, in high yields. The introduction of the photoremovable protecting group in the primary amines was accomplished by alkaline hydrolysis of these propenoic acid ethyl esters (E)-3a and (E)-3b in an aqueous ethanolic solution of potassium hydroxide, followed by the condensation reaction of the resultant propenoic acids (E)-4a and (E)-4b with the primary amines in DMF containing N-hydroxysuccinimide and a water-soluble carbodiimide coupling reagent. The simultaneous formation of benzo[f]coumarin (5a) and 3-methylbenzo[f]coumarin (5b) as byproducts resulted in a decrease in the yields of the protected primary amines (E)-1a–e. Nuclear magnetic resonance (NMR) analysis of the coupling mode and constant for the two acryloyl proton signals confirmed the (E)-configuration of these ethyl propenoate, propenoic acid, and protected amine derivatives.
The ultraviolet (UV) absorption spectra obtained by irradiation of a nitrogen-saturated methanolic solution of (E)-1a (1.0 × 10–4 mol dm–3) for a given period of time at λ ≥ 280 nm with a 450 W high-pressure Hg lamp at room temperature (rt) are shown in Figure 1. UV absorption bands due to the (E)-3-(2-hydroxynaphthalen-1-yl)propenoyl chromophore rapidly decreased and the absorption bands of the photoproduct slowly appeared at ca. 310 and 340 nm. Similar changes in the UV absorption spectra were observed for the other propenamide derivatives (E)-1b–e. Benzo[f]coumarin (5a) was isolated as a byproduct in the synthesis of (E)-1a and it showed characteristic absorption bands at 316 and 348 nm in methanol. However, the slow spectroscopic changes observed suggest the occurrence of a cyclization reaction accompanying the liberation of 4-methoxybenzylamine (6a), as shown in Scheme 2. In addition, the requirement that photoisomerization of (E)-1a must occur prior to the conversion into 5a allows us to assume that the rapid changes in the UV absorption spectra are due to isomerization to the (Z)-isomer. Note that irradiation of (E)-1a at wavelengths greater than 340 nm (λ ≥ 340 nm) under the same conditions caused changes in the UV absorption spectra at almost the same rate as that observed on irradiation at λ ≥ 280 nm.
If side reactions, including the secondary decomposition of 5a and 6a, only contribute to a negligible extent to the photodeprotection reaction shown in Scheme 2, then it is possible to estimate the composition of (E)-1a, (Z)-1a, 5a, and 6a in the reaction mixture by considering the ratio of the area of the characteristic 1H NMR signals for these compounds. In addition, the magnitude of the coupling constant for the two acryloyl proton signals in 1a (J–CH=CHCO–) enables us to differentiate the (E)- and (Z)-isomers. Nitrogen-saturated methanolic solutions of (E)-1a–e (1.0 × 10–3 mol dm–3) were irradiated at λ ≥ 280 nm for 6 h at rt. Two mole equivalents of trifluoroacetic acid compared to (E)-1a–e were then added to the irradiated solutions, and the resulting mixtures were immediately evaporated to dryness in vacuo to analyze the composition of compounds in each mixture. Comparison of the 1H NMR spectra of the reaction mixtures obtained from (E)-1a–e with those of (E)-1a–e, 5a,b, and 6a–d (trifluoroacetate salts) revealed that irradiation of (E)-1a in methanol afforded a mixture comprising (Z)-1a (J–CH=CHCO– = 12.0 Hz), 5a, and 6a along with the starting material (E)-1a (J–CH=CHCO– = 16.5 Hz); however, despite the unambiguous appearance of 5b and 6b–d, the (Z)-isomers of 1b–e could not be detected in the mixtures obtained (Scheme 2). Note that the proton signals due to the side reaction products of 1 or the secondary decomposition products of 5 and 6 were negligible; consequently, the 1H NMR analysis described above enabled an estimation of the conversion of 1 and the composition of the corresponding coumarin derivatives 5 and primary amines 6 (as their trifluoroacetate salts), as indicated in Table 1.
The data in Table 1 demonstrates that irradiation of (E)-1a at λ ≥ 340 nm causes its photodeprotection reaction with almost the same efficiency as irradiation at λ ≥ 280 nm and the conversion of 1a–c, i.e., the photodeprotection efficiency of (E)-1a–c, increases in the following order: 1a < 1b < 1c. The former finding implies that irradiation at λ ≥ 340 nm and λ ≥ 280 nm eventually produces the excited-state (Z)-1a, from which 5a and 6a are formed, at almost the same steady-state concentrations and the latter finding suggests that this order is closely related to the magnitude of electron-withdrawing inductive effects of the methoxybenzyl, cyanobenzyl, and methoxycarbonylmethyl groups. Thus, the above trend in deprotection efficiency confirms the occurrence of heterolytic C(=O)–NH bond cleavage. Comparison of the deprotection efficiency of (E)-1a with that of (E)-1d reveals that the replacement of the 4-methoxybenzylamino chromophore by the 4-methoxyanilino one lowers the deprotection efficiency to some extent. Moreover, the presence of a methyl group attached to the acryloyl vinyl carbon of (E)-1a [(E)-1e] also leads to a small decrease in the deprotection efficiency of this propenamide derivative through its electronic effect. These results are consistent with a mechanism, in which the amide carbonyl carbon in the excited-state (Z)-1 undergoes a nucleophilic attack of the hydroxyl oxygen in competition with the deactivation of this isomer. Furthermore, the acidity of the hydroxyl group in 1 is significantly increased in the excited singlet state; thus, we proposed the process shown in Scheme 3, which provides a rationale for the relatively efficient photodeprotection of the (E)-3-(2-hydroxynaphthalen-1-yl)propenoyl- and (E)-3-(2-hydroxynaphthalen-1-yl)-2-methylpropenoyl-protected primary amines (E)-1.11
The intramolecular proton transfer in (Z)-1* (see Scheme 3) possibly occurs during its lifetime in competition with deactivation to (Z)-1 and isomerization to (E)-1, yielding the tetrahedral intermediate [I]. Subsequent decomposition of this intermediate along with the generation of the coumarin derivatives 5 and primary amines 6 would complete the photodeprotection of (E)-1. As stated above, with the exception of (Z)-1a we were unable to find evidence for any (Z)-isomers derived from (E)-1b–e in the deprotection process of these propenamide derivatives. Analysis of the reaction pathways from (E)-1 to 5 and 6 confirms that the formation of the (Z)-isomer depends on the magnitude of the rate of the isomerization and proton transfer reactions of (Z)-1 relative to the rate of the isomerization reaction of (E)-1 to (Z)-1. Therefore, it is reasonable to interpret the absence of the (Z)-isomer on the basis of the much higher relative rate of the former reaction processes.
In conclusion, the protected primary amines (E)-1 undergo rather efficient photolytic reactions to afford the corresponding benzocoumarins 5 (showing their fluorescence bands at ca. 410 nm) and primary amines 6 in a 1:1 molar ratio without the formation of byproducts. Moreover, irradiation of (E)-1 in methanol at λ ≥ 340 nm, which is not harmful to cells, enables the photofragmentation of these propenoic acid amides with almost the same efficiency as irradiation at λ ≥ 280 nm. Therefore, the introduction into (E)-1 of a substituent that has a high solubility in water, the stability of which must be much higher than that of the corresponding propenoic acid esters in water, would make the resulting water-soluble (E)-1 derivatives potential candidates for a novel caged compound.
EXPERIMENTAL
1H and 13C NMR spectra were obtained from a JEOL JNM-ECA500 spectrometer. Chemical shifts were determined using tetramethylsilane as an internal standard. UV absorption and fluorescence spectra were obtained from a Shimadzu UV-3150 spectrophotometer and a Hitachi F-4500 spectrofluorimeter, respectively. A cell with a 10-mm path length was used. Matrix assisted laser desorption/ionization time-of-flight (MALDI-TOF) mass spectra were recorded on a Shimadzu/Kratos TOF mass spectrometer. Methanol was purified according to the standard procedure and freshly distilled prior to use.12 All other chemicals were obtained from commercial sources at the highest grade available.
Procedure for the synthesis of ethyl (E)-3-(2-hydroxynaphthalen-1-yl)propenoate [(E)-3a] and ethyl (E)-3-(2-hydroxynaphthalen-1-yl)-2-methylpropenoate [(E)-3b]
2a (35 mmol) or 2b (35 mmol) was added to an argon-saturated solution of 2-hydroxy-1-naphthaldehyde (30 mmol) in toluene (45 mL), and the resulting mixture was stirred for 30 min under an argon atmosphere at rt. The solution was evaporated to dryness under reduced pressure to afford an oily residue, which was subjected to column chromatography on silica gel (70–230 mesh) using hexane/EtOAc (1:2 v/v) or CHCl3/EtOAc (1:2 v/v) as eluent. (E)-3a and (E)-3b were then isolated in 90% and 75% yields, respectively.
(E)-3a: pale yellow solid; 1H NMR (500 MHz, DMSO-d6) δ = 1.30 (3H, t, J = 6.9 Hz), 4.23 (2H, q, J = 6.9 Hz), 6.88 (1H, d, J = 16.0 Hz), 7.27 (1H, d, J = 9.2 Hz), 7.37 (1H, dd, J = 6.9, 6.9 Hz), 7.56 (1H, dd, J = 6.9, 6.9 Hz), 7.85 (1H, d, J = 6.9 Hz), 7.86 (1H, d, J = 9.2 Hz), 8.09 (1H, d, J = 6.9 Hz), 8.29 (1H, d, J = 16.0 Hz), 10.81 (1H, s); 13C NMR (125 MHz, DMSO-d6) δ = 14.3, 59.9, 112.1, 118.3, 121.2, 121.9, 123.2, 127.6, 128.0, 128.8, 131.9, 132.6, 137.1, 156.3, 167.2.
(E)-3b: pale yellow solid; 1H NMR (500 MHz, DMSO-d6) δ = 1.34 (3H, t, J = 6.3 Hz), 1.65 (3H, s), 4.33 (2H, q, J = 6.3 Hz), 7.23 (1H, d, J = 7.5 Hz), 7.32 (1H, dd, J = 7.5, 7.5 Hz), 7.45 (1H, dd, J = 7.5, 7.5 Hz), 7.70 (1H, d, J = 7.5 Hz), 7.79 (1H, s), 7.81 (1H, d, J = 7.5 Hz), 8.83 (1H, d, J = 7.5 Hz), 9.94 (1H, s); 13C NMR (125 MHz, DMSO-d6) δ = 14.3, 15.0, 60.4, 114.3, 118.2, 122.8, 123.5, 126.7, 127.7, 128.3, 129.8, 130.9, 131.9, 134.1, 152.4, 167.2.
Procedure for the synthesis of (E)-3-(2-hydroxynaphthalen-1-yl)propenoic acid [(E)-4a] and (E)-3-(2-hydroxynaphthalen-1-yl)-2-methylpropenoic acid [(E)-4b]
Sodium hydroxide (40 mmol) was added to a solution of the ethyl (E)-propenoate derivative (8.0 mmol) prepared as described above in 50 vol% aqueous EtOH (100 mL). The reaction mixture was heated under reflux for 2 h. The mixture was cooled to rt, and the pH was adjusted to 2 by adding hydrochloric acid (3.0 mol dm–3). The resulting precipitates were filtered off, air-dried at rt, and rinsed several times with CHCl3. (E)-4a and (E)-4b were then isolated in 75% and 80% yields, respectively.
(E)-4a: pale yellow solid; 1H NMR (500 MHz, DMSO-d6) δ = 6.80 (1H, d, J = 16.0 Hz), 7.27 (1H, d, J = 8.6 Hz), 7.36 (1H, dd, J = 6.9, 6.9 Hz), 7.54 (1H, dd, J = 6.9, 6.9 Hz), 7.84 (1H, d, J = 6.9 Hz), 7.84 (1H, d, J = 8.6 Hz), 8.09 (1H, d, J = 6.9 Hz), 8.23 (1H, d, J = 16.0 Hz), 10.75 (1H, s), 12.31 (1H, s); 13C NMR (125 MHz, DMSO-d6) δ = 112.4, 118.4, 122.1, 122.5, 123.2, 127.4, 128.1, 128.8, 131.6, 132.7, 136.9, 156.0, 168.6.
(E)-4b: pale yellow solid; 1H NMR (500 MHz, DMSO-d6) δ = 1.62 (3H, s), 7.22 (1H, d, J = 8.0 Hz), 7.31 (1H, dd, J = 8.0, 8.6 Hz), 7.45 (1H, dd, J = 8.0, 8.6 Hz), 7.54 (1H, d, J = 8.0 Hz), 7.74 (1H, s), 7.74 (1H, d, J = 8.0 Hz), 8.22 (1H, d, J = 8.0 Hz), 9.89 (1H, s), 12.50 (1H, s); 13C NMR (125 MHz, DMSO-d6) δ = 15.0, 114.7, 118.3, 122.8, 123.6, 126.6, 127.7, 128.3, 129.6, 131.6, 132.0, 133.6, 152.3, 168.6.
Procedure for the synthesis of (E)-3-(2-hydroxynaphthalen-1-yl)propenoyl- and (E)-3-(2-hydroxynaphthalen-1-yl)-2-methylpropenoyl-protected primary amines [(E)-1a–e]
The (E)-propenoic acid derivative (4.0 mmol) prepared by the above procedure was dissolved in dry DMF (30 mL) containing N-hydroxysuccinimide (4.5 mmol) at rt. 1-Ethyl-3-(3-dimethylamino-propyl)carbodiimide hydrochloride (4.5 mmol) was added to the DMF solution and the reaction mixture was stirred for 3 h at rt. The appropriate primary amine hydrochloride (4.0 mmol) and triethylamine (5.0 mmol) were added to this mixture and dissolved by stirring. The solution was stirred for a further 12 h at rt and poured into a separatory funnel together with water (100 mL). The products were extracted with EtOAc (80 mL × 3). The combined extracts were rinsed with water (50 mL × 2), dried over anhydrous sodium sulfate, and evaporated to dryness in vacuo. The residual solid was purified by column chromatography on silica gel (70–230 mesh) using CHCl3/EtOAc (1:1 v/v) or hexane/acetone (1:2 v/v) as eluent. The corresponding protected primary amine (E)-1 was isolated in 18%–30% yield along with a small amount of benzo[f]coumarin (5a) or 3-methylbenzo[f]coumarin (5b) as a byproduct.
N-4-Methoxybenzyl (E)-3-(2-hydroxynaphthalen-1-yl)propenamide [(E)-1a]: 26% yield; pale yellow solid; 1H NMR (500 MHz, DMSO-d6) δ = 3.73 (3H, s), 4.35 (2H, d, J = 6.0 Hz), 6.91 (2H, d, J = 7.0 Hz), 6.97 (1H, d, J = 16.5 Hz), 7.23 (1H, d, J = 7.5 Hz), 7.24 (2H, d, J = 7.0 Hz), 7.35 (1H, dd, J = 7.5, 7.5 Hz), 7.50 (1H, dd, J = 7.5, 7.5 Hz), 7.78 (1H, d, J = 7.5 Hz), 7.81 (1H, d, J = 7.5 Hz), 8.05 (1H, d, J = 16.5 Hz), 8.08 (1H, d, J = 7.5 Hz), 8.61 (1H, t, J = 6.0 Hz), 10.43 (1H, s); 13C NMR (125 MHz, DMSO-d6) δ = 41.8, 55.1, 113.4, 113.7 (2C), 118.3, 122.4 (2C), 125.9, 127.1, 128.1, 128.6, 128.8 (2C), 130.5, 131.6, 131.9, 132.7, 155.0, 158.2, 166.0. MALDI-TOF-MS m/z calcd for C21H19NNaO3: 356.14 [M + Na]+. Found: 356.41.
N-4-Cyanobenzyl (E)-3-(2-hydroxynaphthalen-1-yl)propenamide [(E)-1b]: 18% yield; pale yellow solid; 1H NMR (500 MHz, DMSO-d6) δ = 4.53 (2H, d, J = 5.7 Hz), 7.05 (1H, d, J = 16.0 Hz), 7.27 (1H, d, J = 8.6 Hz), 7.35 (1H, dd, J = 6.9, 6.9 Hz), 7.52 (1H, dd, J = 6.9, 6.9 Hz), 7.52 (2H, d, J = 8.6 Hz), 7.81 (1H, d, J = 8.6 Hz), 7.83 (2H, d, J = 8.6 Hz), 7.84 (1H, d, J = 6.9 Hz), 8.11 (1H, d, J = 6.9 Hz), 8.13 (1H, d, J = 16.0 Hz), 8.85 (1H, t, J = 5.7 Hz), 10.53 (1H, s); 13C NMR (125 MHz, DMSO-d6) δ = 42.1, 109.5, 113.1, 118.3, 118.9, 122.3, 123.0, 125.3, 127.1, 128.1, 128.2 (2C), 128.7, 130.7, 132.3 (3C), 132.7, 145.7, 155.2, 166.4. MALDI-TOF-MS m/z calcd for C21H16N2NaO2: 351.42 [M + Na]+. Found: 351.36.
N-(S)-(1-Methoxycarbonyl-2-phenyl)ethyl (E)-3-(2-hydroxynaphthalen-1-yl)propenamide [(E)-1c]: 30% yield; pale yellow solid; 1H NMR (500 MHz, DMSO-d6) δ = 3.00 (1H, dd, J = 9.7, 13.7 Hz), 3.11 (1H, dd, J = 5.7, 13.7 Hz), 3.64 (3H, s), 4.64 (1H, ddd, J = 5.7, 8.0, 9.7 Hz), 7.00 (1H, d, J = 16.0 Hz), 7.20–7.31 (6H, m), 7.34 (1H, dd, J = 6.9, 6.9 Hz), 7.51 (1H, dd, J = 6.9, 6.9 Hz), 7.79 (1H, d, J = 8.6 Hz), 7.83 (1H, d, J = 6.9 Hz), 8.03 (1H, d, J = 16.0 Hz), 8.05 (1H, d, J = 6.9 Hz), 8.69 (1H, d, J = 8.0 Hz), 10.51 (1H, s); 13C NMR (125 MHz, DMSO-d6) δ = 36.7, 51.9, 54.0, 113.0, 118.3, 122.2, 123.0, 124.8, 126.6, 127.2, 128.1, 128.3 (2C), 128.7, 129.1 (2C), 130.7, 132.5, 132.7, 137.4, 155.3, 166.2, 172.4. MALDI-TOF-MS m/z calcd for C23H20NNaO4: 398.54 [M + Na]+. Found: 398.42.
N-4-Methoxyphenyl (E)-3-(2-hydroxynaphthalen-1-yl)propenamide [(E)-1d]: 22% yield; pale yellow solid; 1H NMR (500 MHz, DMSO-d6) δ = 3.73 (3H, s), 6.90 (2H, d, J = 7.0 Hz), 7.10 (1H, d, J = 16.0 Hz), 7.25 (1H, d, J = 9.5 Hz), 7.35 (1H, dd, J = 7.5, 9.5 Hz), 7.53 (1H, dd, J = 7.5, 9.5 Hz), 7.66 (2H, d, J = 7.0 Hz), 7.81 (1H, d, J = 7.5 Hz), 7.83 (1H, d, J = 7.5 Hz), 8.12 (1H, d, J = 9.5 Hz), 8.17 (1H, d, J = 16.0 Hz), 10.09 (1H, s), 10.50 (1H, s); 13C NMR (125 MHz, DMSO-d6) δ = 55.1, 114.0, 114.5 (2C), 118.3, 122.4 (2C), 123.9, 124.1, 127.3, 128.6, 128.8, 129.0, 130.8, 132.6, 132.7, 132.8, 155.2, 155.8, 164.8. MALDI-TOF-MS m/z calcd for C20H17NNaO3: 342.12 [M + Na]+. Found: 342.48.
N-4-Methoxybenzyl (E)-3-(2-hydroxynaphthalen-1-yl)-2-methylpropenamide [(E)-1e]: 20% yield; pale yellow solid; 1H NMR (500 MHz, DMSO-d6) δ = 1.34 (3H, s), 3.73 (3H, s), 4.35 (2H, d, J = 6.0 Hz), 6.91 (2H, d, J = 7.0 Hz), 7.23 (1H, d, J = 7.5 Hz), 7.24 (2H, d, J = 7.0 Hz), 7.35 (1H, dd, J = 7.5, 7.5 Hz), 7.50 (1H, dd, J = 7.5, 7.5 Hz), 7.74 (1H, s), 7.78 (1H, d, J = 7.5 Hz), 7.81 (1H, d, J = 7.5 Hz), 8.08 (1H, d, J = 7.5 Hz), 8.61 (1H, t, J = 6.0 Hz), 10.40 (1H, s); 13C NMR (125 MHz, DMSO-d6) δ = 15.2, 33.6, 55.1, 113.7 (2C), 115.1, 118.3, 122.7, 123.8, 126.4, 127.6, 127.7, 128.2, 128.7 (2C), 129.1, 131.9, 132.3, 135.5, 152.1, 158.2, 168.0. MALDI-TOF-MS m/z calcd for C22H21NNaO3: 370.15 [M + Na]+. Found: 370.41.
5a: White solid; 1H NMR (500 MHz, DMSO-d6) δ = 6.65 (1H, d, J = 9.7 Hz), 7.59 (1H, d, J = 9.2 Hz), 7.64 (1H, dd, J = 7.4, 7.4 Hz), 7.76 (1H, dd, J = 7.4, 7.4 Hz), 8.07 (1H, d, J = 7.4 Hz), 8.21 (1H, d, J = 9.2 Hz), 8.56 (1H, d, J = 7.4 Hz), 8.95 (1H, d, J = 9.7 Hz); 13C NMR (125 MHz, DMSO-d6) δ = 112.9, 115.4, 116.8, 122.3, 126.1, 128.3, 128.8, 129.9, 133.2 (2C), 140.4, 153.3, 160.0. MALDI-TOF-MS m/z calcd for C13H8NaO2: 219.22 [M + Na]+. Found: 219.20.
5b: White solid; 1H NMR (500 MHz, DMSO-d6) δ = 2.23 (3H, s), 7.57 (1H, d, J = 7.5 Hz), 7.62 (1H, dd, J = 6.5, 6.5 Hz), 7.75 (1H, dd, J = 6.5, 6.5 Hz), 8.05 (1H, d, J = 6.5 Hz), 8.12 (1H, d, J = 7.5 Hz), 8.55 (1H, d, J = 6.5 Hz), 8.81 (1H, s).
The NMR spectral behavior of 5a13a,b and 5b13a agreed with that described in the literature.
Procedure for the irradiation of (E)-1a–e
The photochemical behavior of (E)-1, including isomerization to the corresponding (Z)-isomer, was analyzed. A nitrogen-saturated MeOH solution of each propenamide derivative (1.0 × 10–4 mol dm–3, 40 mL) was placed in a Pyrex glass container and irradiated for a given period of time with light passed through a Toshiba IRA-25S glass filter (λ ≥ 280 nm) or Toshiba IRA-25S and Corning 0-52 glass filters (λ ≥ 340 nm) from a 450 W high-pressure Hg lamp mounted in a purpose-built lamp house (external irradiation). At suitable time intervals, an aliquot of the irradiated solution was transferred into a quartz glass UV cell and the UV absorption spectrum was recorded at rt.
The structure and composition of the (E)-1-derived photoproducts were then determined as follows: 1) A nitrogen-saturated MeOH solution of each propenamide derivative (1.0 × 10–3 mol dm–3, 40 mL) was placed in a Pyrex glass container and irradiated for 6 h at λ ≥ 280 nm from the same light source as above. 2) A MeOH solution of trifluoroacetic acid (2.0 × 10–2 mol dm–3, 4 mL) was added to the respective irradiated solution. 3) The resulting solution was immediately evaporated to dryness under reduced pressure to afford a residual solid, which was dissolved in DMSO-d6 and analyzed by 1H NMR spectroscopy. The structure of the coumarin derivatives 5a,b and trifluoroacetate salts of the primary amines 6a–d was identified by comparing the 1H NMR spectrum of this residual solid with that of an equimolar mixture of the corresponding authentic coumarin and amine derivatives. In addition, to determine the 1H NMR yields of 5a and 6a, the (E)-1a-derived residual solid was dissolved in DMSO-d6 (1.0 mL) containing 1,3,5-trimethoxybenzene (1.0 × 10–2 mol dm–3) as an internal standard and subjected to 1H NMR spectral analysis.
References
1. a) R. W. Binkley and T. W. Flechtner, 'Synthetic Organic Photochemistry', ed. by W. M. Horspool, Plenum, New York, 1984, pp. 375–423; CrossRef b) F. Guillier, D. Orain, and M. Bradley, Chem. Rev., 2000, 100, 2091; CrossRef c) C. G. Bochet, J. Chem. Soc., Perkin Trans. 1, 2002, 125; d) P. Klan, T. Solomek, C. G. Bochet, A. Blanc, R. Givens, M. Rubina, V. Popik, A. Kostikov, and J. Wirz, Chem. Rev., 2013, 113, 119. CrossRef
2. a) A. Abad, D. Mellier, J. P. Pete, and C. Portella, Tetrahedron Lett., 1971, 4555; CrossRef b) B. Amit, U. Zehavi, and A. Patchornik, J. Org. Chem., 1974, 39, 192; CrossRef c) S. Hanessian and R. Masse, Carbohydr. Res., 1977, 54, 142; CrossRef d) J. A. Pincock and A. Jurgens, Tetrahedron Lett., 1979, 1029; CrossRef e) W. Yuan, K. Fearon, and M. H. Gelb, J. Org. Chem., 1989, 54, 906; CrossRef f) S. A. Sundberg, R. W. Barrett, M. Pirrung, A. L. Lu, B. Kiangsoontra, and C. P. Holmes, J. Am. Chem. Soc., 1995, 117, 12050; CrossRef g) J. F. Cameron, C. G. Willson, and J. M. J. Frechet, J. Chem. Soc., Chem. Commun., 1995, 923; CrossRef h) M. C. Pirrung and C.-Y. Huang, Tetrahedron Lett., 1995, 36, 5883; CrossRef i) J. F. Cameron, C. G. Willson, and J. M. J. Frechet, J. Chem. Soc., Perkin Trans. 1, 1997, 2429; CrossRef j) G. Papageorgiou and J. E. T. Corrie, Tetrahedron, 1997, 53, 3917; CrossRef k) J. Cossy and H. Rakotoarisoa, Tetrahedron Lett., 2000, 41, 2097; CrossRef l) C. G. Bochet, Tetrahedron Lett., 2000, 41, 6341; CrossRef m) A. Stutz, C. Hobartner, and S. Pitsch, Helv. Chim. Acta, 2000, 83, 2477; CrossRef n) H. Du and M. K. Boyd, Tetrahedron Lett., 2001, 42, 6645; CrossRef o) R. O. Schoenleber and B. Giese, Synlett, 2003, 501; CrossRef p) K. R. Bhushan, C. DeLisi, and R. A. Laursen, Tetrahedron Lett., 2003, 44, 8585. CrossRef
3. T. Igarashi, M. Shimokawa, M. Iwasaki, K. Nagata, M. Fujii, and T. Sakurai, Synlett, 2007, 1436. CrossRef
4. G. Mayer and A. Heckel, Angew. Chem. Int. Ed., 2006, 45, 4900. CrossRef
5. a) A. K. Singh and P. K. Khade, Tetrahedron Lett., 2005, 46, 5563; CrossRef b) P. K. Khade and A. K. Singh, Tetrahedron Lett., 2007, 48, 6920. CrossRef
6. a) V. R. Shembekar, Y. Chen, B. K. Carpenter, and G. P. Hess, Biochemistry, 2005, 44, 7107; CrossRef b) A. Specht, J.-S. Thomann, K. Alarcon, W. Wittayanan, D. Ogden, T. Furuta, Y. Kurakawa, and M. Goeldner, ChemBioChem, 2006, 7, 1690; CrossRef c) A. Soldevilla and A. G. Griesbeck, J. Am. Chem. Soc., 2006, 128, 16472; CrossRef d) V. R. Shembekar, Y. Chen, B. K. Carpenter, and G. P. Hess, Biochemistry, 2007, 46, 5479; CrossRef e) N. Senda, A. Momotake, and T. Arai, Bull. Chem. Soc. Jpn., 2007, 80, 2384; CrossRef f) M. J. G. Fernandes, M. S. T. Gonçalves, and S. P. G. Costa, Tetrahedron, 2007, 63, 10133; CrossRef g) K. Stensrud, J. Noh, K. Kandler, J. Wirz, D. Heger, and R. S. Givens, J. Org. Chem., 2009, 74, 5219. CrossRef
7. S. Petersen, J. M. Alonso, A. Specht, P. Duodu, M. Goeldner, and A. del Campo, Angew. Chem. Int. Ed., 2008, 47, 3192. CrossRef
8. P. Rathert, T. Rasko, M. Roth, K. Slaska-Kiss, A. Pingoud, A. Kiss, and A. Jeltsch, ChemBioChem, 2007, 8, 202. CrossRef
9. a) D. Geißler, W. Kresse, B. Wiesner, J. Bendig, H. Kettenmann, and V. Hagen, ChemBioChem, 2003, 4, 162; CrossRef b) V. Hagen, S. Frings, B. Wiesner, S. Helm, U. B. Kaupp, and J. Bendig, ChemBioChem, 2003, 4, 434; CrossRef c) X. Tang and I. J. Dmochowski, Org. Lett., 2005, 7, 279. CrossRef
10. a) C. Shin, Y. Nakajima, T. Haga, and Y. Sato, Bull. Chem. Soc. Jpn., 1986, 59, 3917; CrossRef b) H. Li, J. Yang, and N. A. Porter, J. Photochem. Photobiol., A, 2005, 169, 289; c) N. Gagey, P. Neveu, C. Benbrahim, B. Goetz, I. Aujard, J.-B. Baudin, and L. Jullien, J. Am. Chem. Soc., 2007, 129, 9986; CrossRef ; CrossRef e) S. Fukuda, K. Sakayori, and K. Arihara, Jpn. Kokai Tokkyo Koho, JP 2011-222788A.
11. J. Gao, N. Li, and M. Freindorf, J. Am. Chem. Soc., 1996, 118, 4912. CrossRef
12. J. A. Riddick, W. B. Bunger, and T. K. Sakano, 'Organic Solvents', 4th ed., Wiley, Chichester, 1986.
13. a) R. G. Harvey, C. Cortez, T. P. Ananthanarayan, and S. Schmolka, J. Org. Chem., 1988, 53, 3936; CrossRef b) Y.-S. Hon, T.-W. Tseng, and C.-Y. Cheng, Chem. Commun., 2009, 5618. CrossRef