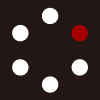
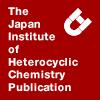
HETEROCYCLES
An International Journal for Reviews and Communications in Heterocyclic ChemistryWeb Edition ISSN: 1881-0942
Published online by The Japan Institute of Heterocyclic Chemistry
e-Journal
Full Text HTML
Received, 10th October, 2014, Accepted, 22nd December, 2014, Published online, 7th January, 2015.
DOI: 10.3987/COM-14-13104
■ Cyclohexane-Fused Pyridine Derivatives with Photophysical Properties: Synthesis by “Three-Component” Domino Reaction and Structural Optimization by DFT Calculations
Jianqiang Wang, Ziping Li, Xinzhi Wang, Yixin Zhou, and Cheng Guo*
College of Science, Nanjing Tech University, Nanjing 211816, China
Abstract
An environment-friendly and highly efficient method for the preparation of 2-amino-4-phenyl-3-cyano-5,6-dihydrobenzo[h]quinoline derivatives was successfully developed via arylaldehyde, 3,4-dihydro-1(2H)- naphthalenone, malononitrile, ammonium acetate in ethanol by “three-component” domino reaction under microwave irradiation. The reaction conditions were optimized by changing microwave power, temperature and time. Their structures were characterized by ESI-MS, 1H-NMR, 13C-NMR, and IR. Their photophysical properties were detected by UV-Vis and fluorescent spectra. The results of density functional theory (DFT) and time-dependent density functional theory (TDDFT) calculations were applied to simulate spectra of the compounds, which show excellent agreement with observed spectra and provide reasonable explanation on the structures, the molecular frontier orbital, and the electronic absorption spectra.INTRODUCTION
In recent years, the preparation of some useful organic intermediate products is still an important challenge to the researchers.1 The highly efficient preparation method is eagerly developed with the increasing in the demanding of these compounds. Microwave irradiation as an effective manner has been widely used in many fields since 1986. Especially, organic synthesis under microwave irradiation has been attracted much attention because of its amazing advantages: fast heating manner, higher yields under milder reaction conditions and easy separation of the product, when compared with conventional heating. Moreover, many intermediates of drugs have been manufactured under microwave irradiation.2-4 Thereby, in order to develop more new powerful protocols in combinational synthesis, microwave irradiation is chose as the energy source to drive chemical reactions.
It is well known that the pyridine derivatives with nitrogen-containing heterocyclic structure possess the important biological activity.5-7 And the aminopyridine is the typical MK-2 inhibitors which can guard against and cure the TNF α mediated disease. At the same time, the aminopyridine is the raw material of the aminomethylpyridine, which is also used in the prevention and cure of diabetes.8-14 Besides, 2-amino-3-cyanopyridine is also an important heterocyclic compound because it is utilized as reactant or reaction intermediate to architect more complex heterocyclic structures.15 Since the pyridines have been further studied, varieties of new techniques are induced in the preparation system. Sakurai and Midorikawa,13 Kambe,17 Li18 and Yan19 had all explored such reactions related to pyridines. However, all of the reactions were carried out under the conventional heating, which needs longer time and more complex separation.
In order to improve efficiency of building these important heterocyclic compounds, microwave as one of the potential green chemistry techniques is applied in the reaction system. Previously, many heterocyclic compounds were synthesized under microwave irradiation in our groups.20 Herein, in the manuscript, an efficient one-pot combinatorial method for preparing a series of 2-amino-4-phenyl-3-cyano-5, 6-dihydrobenzo[h]quinoline is reported. Their structures were characterized by IR, 1H NMR, 13C NMR and ESI-MS. Meanwhile, the reaction conditions under microwave irradiation are optimized by comparing with conventional heating. The photophysical properties of these compounds were detected by UV-Vis and fluorescence techniques. The optimized structures, frontier orbital and optical properties of the compounds were investigated by using density functional theory (DFT) and time dependent density functional theory (TDDFT) to provide theoretical understanding.
RESULTS AND DISCUSSION
Syntheses of the compounds
The synthetic route to compounds 4 (2-amino-4-phenyl-3-cyano-5,6-dihydrobenzo[h]quinoline derivatives) are outlined in Scheme 1. These compounds were obtained from the one-port three-component condensation reaction by using arylaldehyde, 3,4-dihydro-1(2H)-naphthalenone, malononitrile as the starting materials in the presence of the catalyst ammonium acetate under microwave irradiation. Kinds of compounds arylaldehyde with the different substituted groups were introduced into the molecular skeleton to obtain series of compounds 4. They were characterized by melting point measurements, Fourier transform infrared (FTIR) spectroscopy (see Figures S1-S8 in the supplement content), 1H NMR and 13C NMR (see Figures S9-S24 in the supplement content) and high-resolution mass spectrometer (HRMS) (see Figures S25-S32 in the supplement content).
Optimization of conditions under microwave irradiation
In order to optimize the reaction conditions under the microwave irradiation, we did plenty of studies by changing the important conditions including microwave irradiation time and power. As we know, microwave irradiation is a high speed way to heat the reaction system to a certain temperature in short time. If the reaction time is too short, little or evens no product is available. On the other hand, if the reaction system is too long, a large number of by-products will be formed to reduce the selectivity and conversion of the main products. Therefore, the exploration of the influence of time seems even more important in order to obtain high yield and better selectivity of the products under the microwave irradiation.
In this paper, we take the compound 4a as the example to observe the effect of microwave irradiation time. The compound 4a was prepared in the solution of ethanol under microwave irradiation with the power of 400 W. Microwave irradiation time was increased from 2 min to 12 min in an interval of 2 min to observe the change of yield of compound 4a. As shown in Figure 1, the yield of compound 4a was speedily improved with the increasing of microwave irradiation time in the range from 2 min to 6 min because microwave irradiation as a dielectric heating method could result in the vigorous molecular vibration of the reactants and enhance the average molecular energy to largely improve the reaction temperature and speed in a short time. However, when the microwave irradiation time exceeded 6 min, the yield of compound 4a decreased because the by-products were largely formed and the product 4a was probably decomposed or carbonized into the other compounds. Then, the yield of compound 4a rapidly reduced. Therefore, the perfect microwave irradiation time is 6 min in the preparation of compound 4a.
The different structures of 2-amino-4-phenyl-3-cyano-5,6-dihydrobenzo[h]quinolines 4 were architected under the same conditions (including solvent and microwave power) to compound 4a but the optimized microwave irradiation time is different in the preparation of these compounds. The results were listed in Table 1. As shown in Table 1, there are obvious differences in the optimized microwave irradiation time and yield to the different products 4. Nevertheless, all of the compounds 4 were prepared in only several minutes (a very short time) under the microwave irradiation.
When the organic reaction was implemented under microwave irradiation, the microwave irradiation power is another important parameter to optimize reaction conditions. Figure 2 indicates the interaction between yield of product 4a and microwave irradiation power. We can find that the microwave irradiation power has large effect on the yield. The compound 4a was prepared under the microwave irradiation power from 200W to 600W in the order of 100W apart for 6 min. If the power was under 400 W, the yield of compound 4a increased with the strengthening of power because the reactants were activated step by step in the increasing of power. However, if the power was larger than the point (400 W), the yield of product 4a rapidly reduced under the higher microwave irradiation power. The obvious differences were observed because a large number of by-products were formed when the reactants were vigorously activated under higher microwave irradiation power. Moreover, the target product were probably decomposed or carbonized by the higher energy resulted from strong irradiation power. Therefore, according to the above analysis, the microwave irradiation power has direct effect on the preparation of compounds 4. Furthermore, the microwave irradiation power of 400 W is the relatively appropriate to synthesize these compounds 4.
In order to further explain the advantage of microwave irradiation as the energy to drive the organic synthesis, the compounds 4 were prepared by conventional heating at the same temperature of 90 oC. We compared the reaction time and yield of compounds under the two different heating methods. When the compounds 4 were synthesized by microwave irradiation, little time was needed to complete the whole reaction, as shown in Table 1. However, when the compounds 4 were synthesized by conventional heating, the whole reaction required 240 min. In addition, there is a large difference in the yield of compounds 4. As shown in Table 1, the yields of compounds 4 were clearly increased when these compounds were prepared by microwave irradiation method. Moreover, the yields of most of compounds 4 under microwave irradiation are 2 times higher than ones of these compounds under conventional heating in average, though the reaction time is 40 times less than the conventional heating method. These results further indicate that the microwave irradiation synthesis is a very effective method of architecting heterocyclic structure in a short time and a high yield.
In order to clearly know how the microwave irradiation drives the preparation of compounds 4, the possible mechanism was studied and deduced according to the relevant knowledge of organic chemistry. As shown in Scheme 2, at first, the Knoevenagel reaction between the aromatic aldehyde 1 and malononitrile 3 occurred to form 2-arylmethylenemalononitrile in the presence of the catalyst ammonium acetate. Simultaneously, 3,4-dihydro-1(2H)-naphthalenone 2 was reacted with ammonium acetate to yield imine 10. Then, the compound 11 was formed via the isomerization of imine. The compound 11 and compound 5 interact with each other by the Michael addition reaction to produce compound 6, followed by cyclization, isomerization, and aromatization to afford the final product. The intermediate 9 is very easy to oxidize to the final product because a larger conjugated system can be formed by an aromatic substitution with different groups in the structure.21 The above mechanism also indicated that the compounds 4 were prepared by the domino reactions.
Photophysical properties
The UV-Vis absorption spectrum of all the compounds 4 in ethanol are shown in Figure 3. The two absorption bands were clearly observed in the UV-Vis spectra of compounds 4: a relatively low energy band around 285 nm and a broad high intensity bands in the 310-425 nm region. The spectra of the compounds 4a-4e and 4g-4h are very similar, except for the absorption intensity. However, the red shift of the maximum absorption wavelength is observed in the spectra of the compounds 4f in the absorption bands from 310 nm to 425 nm. The result of red shift is tightly related to the molecular geometric configuration of compound 4f. The furan group of compound 4f largely increases the π conjugate effect because the furan ring and 5, 6-dihydrobenzo[h]quinoline is almost in the same plane. Thus, the large π conjugate effect results in the red shift of compound 4f in UV-Vis absorption spectra.
Figure 4 indicated that the fluorescent intensity of compounds 4 were excited by 222 nm in the solution of ethanol (c=2×10-6M). The compounds 4 with the diverse groups in the aromatic ring possess different fluorescent intensity and maximum emission wavelength at the same excited wavelength. The fluorescent intensity of compound 4g is the strongest among these compounds because the large π conjugated structure is formed in the presence of naphthyl group. It is well known that these large π conjugated structure is easy to produce strong fluorescence intensity. However, the fluorescent intensity of compounds 4c, 4d, 4e and 4h are relatively low because of the internal heavy atom effect induced by the fluoro groups in their structures. Besides the above effect of groups in the fluorescent intensity, the position of substituted groups in the aromatic ring also largely affects the fluorescent intensity of these compounds. As shown in Figure 4, the fluorescent intensity of compound 4c is stronger than one of compound 4d because the ortho-position of aromatic ring can enhance the fluorescent intensity. Conversely, the meta-position of aromatic ring decreases the fluorescent intensity.22 In order to further indicate the fluorescent characteristic of these compounds 4, their fluorescence quantum yields were obtained by using L-tryptophan as a reference. The fluorescence quantum yield of compound 4g is 0.73. It is the highest in all of the compounds. However, the fluorescence quantum yield of compound 4e is only 0.33. The results indicate that the compound 4g possesses good fluorescent performance. The fluorescent quantum yields of the other compounds 4a, 4b, 4c, 4d, 4f and 4h are respectively 0.46, 0.45, 0.38, 0.35, 0.43 and 0.34. These differences of fluorescence quantum yield are in accord with the fluorescent intensity showed in Figure 4. Furthermore, these compounds can be used as the potential fluorescent materials.
Geometric optimization and conformational study
The structures of compounds 4a-4h have been optimized with DFT calculation method. Optimized molecular geometry of the most stable form is shown in Figure 3. All of the molecular conformations look like similar structure with two fundamental ring including 2-amino-3-cyano-5,6-dihydrobenzo[h]- quinoline as static part and aromatic ring with different substituent groups as variable part. It is interesting to see that the above two parts in the structure of compounds 4 are not in the same plane except the compound 4f in the optimized geometry. Thus, the bigger conjugation plane is formed in the optimized molecular structure of compound 4f. Molecular orbitals calculations provide a detailed description of orbitals including spatial characteristics, nodal patterns and individual atom contributions. The contour plots of the frontier orbitals including the Highest Occupied Molecular Orbitals (HOMOs) and the Lowest Unoccupied Molecular Orbitals (LUMOs) are also observed in Figure 5. We can find that both orbitals are substantially distributed over 2-amino-3-cyano-5, 6-dihydrobenzo[h]quinoline plane. It can be seen from the Figure 5 that the HOMO orbitals are located on the static part of quinoline ring. On the other hand, the LUMO are localized mainly on the aromatic ring and therefore the different substituted group has an influence on the electron acceptance ability.
In order to understand the main electronic transitions of compounds 4, Time Dependent Density Functional Theory (TDDFT) calculations were used to study the absorption spectra on the basis of the optimized geometries. Table 2 lists the calculated maximum absorption wavelength and oscillator strengths for the most relevant singlet excite states of compounds 4 in ethanol. The calculated maximum absorption maximum of compound 4f shows longest wavelength than that of the other compounds. This is in good agreement with the maximum absorption wavelength in experimental spectra. And, the range of the maximum absorption wavelength is from 340 nm to 420 nm in the experimental spectra of compounds 4. In spite of a little difference, the important features of the spectra have been correctly simulated. The electronic transition with the highest oscillator strength of the calculated maximum absorption wavelength is close to the experimental spectra.
EXPERIMENTAL
General method for the synthesis of 2-amino-4-phenyl-3-cyano-5,6-dihydrobenzo[h]quinoline under microwave irradiation:
According to the Scheme 1, The aromatic aldehyde 1 (2 mmol), 3,4-dihydro-1(2H)-naphthalenone (2mmol), malononitrile 3 (2 mmol), NH4OAc (16 mmol) and absolute EtOH (3 mL) were added in the 50 mL of three-necked, round-bottomed flask. The mixtures were placed into the microwave reaction system. The reaction condition was controlled at 90 oC and the microwave power was 400 W for 4-6 min. A small amount of reaction mixture was used to monitor the reaction progress by thin layer chromatography (TLC). Then, the reaction mixture was cooled for several hours and was filtered to the crude product. The solid product was crystallized with ethanol to obtain the compound 4.
General method for the synthesis of 2-amino-4-phenyl-3-cyano-5,6-dihydrobenzo[h]quinoline under classical conditions:
The aromatic aldehyde 1 (2mmol), 3,4-dihydro-1(2H)-naphthalenone 2 (2mmol), malononitrile 3 (2 mmol), NH4OAc (16 mmol) and absolute EtOH (2 mL) were added in the 50 mL of three-necked, round-bottomed flask. The mixture was heated in the oil bath and kept refluxing at 90 oC for 4 h. Then, the reaction mixture was cooled and filtered. The compound 4 was obtained by crystallizing with EtOH.
COMPOUND CHARACTERIZATION
Microwave reaction was carried out in WF-4000C microwave system. Melting points were measured on X-4 digital display melting point meter. The 1HNMR spectra were recorded on Bruker AC-400 (400 MHz) NMR spectrometer using DMSO-d6 as the solvent. All of the chemical shifts were reported in parts per million relative to internal standard tetramethylsilane, positive chemical shifts denote higher frequency from the given reference. The 13C NMR spectra were recorded on Bruker AV-400 (400 MHz) NMR spectrometer using DMSO-d6 as the solvent. The FTIR spectrum were determined using Nicolet Is5 FTIR spectrometer in the region from 4000 to 400cm-1 at 298K, and all of the samples were mixed with KBr at the ratio of 3:97 (mol/mol) and then pressed. The MS (ESI) were detected on Aglient 6550 iFunnel Q-TOF. Purity of the compounds was checked by thin layer chromatography (TLC). The UV-Vis absorption spectra were obtained on an Agilent-8453 UV-Visible spectroscopy using the ethanol as the solvent. The values of wavelength are expressed in nanometers (nm). The fluorescence spectra were recorded using a Luminescence Spectrometer (Perkin Elmer. precisely LS55).
2-Amino-4-phenyl-5,6-dihydrobenzo[h]quinoline-3-carbonitrile (4a). The compound was obtained as orange crystals, yield 63%; mp 145-147 ℃; IR (KBr, v, cm-1) : 3475, 3359 ν(NH2, Ar) , 3211, 2943, 2831 ν(=C-H), 2213 ν(CN), 1619, 1554, 1497, 1460 v(C=C, Ar), 1431 v(C=N), 771, 752,700,655 δ(C-H) cm-1;1H NMR (400 MHz, DMSO-d6) (δ, ppm): 2.50 (t, J=8.36Hz, 2H, CH2), 2.74 (t, J=7.18 Hz, 2H, CH2), 6.81 (s, 2H, NH2), 7.25-7.27 (m, 1H, ArH), 7.36-7.40 (m, 4H, ArH), 7.48-7.57 (m, 3H, ArH), 8.18-8.21 (m, 1H, ArH); 13C NMR (100MHz, DMSO-d6) (δ, ppm): 23.9, 27.3, 88.7, 116.8, 118.1, 125.6, 126.7, 127.8, 128.4, 128.5, 128.7, 130.1, 133.4, 136.0, 139.1, 153.1, 154.0, 158.8; HRMS(ESI) m/z: 298.1361 [M+H]+, 320.1181 [M+Na]+.
2-Amino-4-(3-methylphenyl)-5,6-dihydrobenzo[h]quinoline-3-carbonitrile (4b). The compound was obtained as pale yellow crystals, yield 85%; mp 131-133 ℃;IR (KBr, v, cm-1): 3485, 3375 v(NH2, Ar), 3205, 3039, 2934 v(=C-H), 2205 v(CN), 1613, 1556, 1488, 1460 v(C=C, Ar), 1437 v(C=N), 765, 756, 736, 714 δ(C-H)cm-1; 1H NMR (400 MHz, DMSO-d6) (δ, ppm): 2.38 (s, 3H, CH3), 2.48 (t, J=9.66Hz, 2H, CH2) , 2.74 (t, J=7.12Hz, 2H, CH2), 6.79 (s, 2H, NH2), 7.16-7.32 (m, 4H, ArH), 7.36-7.44 (m, 3H, ArH), 8.18-8.20 (m, 1H, ArH); 13C NMR (100MHz, DMSO-d6) (δ, ppm): 21.0, 23.9, 27.3, 88.7, 116.9, 118.1, 125.5, 125.6, 126.7, 127.8, 128.5, 128.8, 129.4, 130.1, 133.4, 136.0, 137.8, 139.1, 153.2, 153.9, 158.8; HRMS(ESI) m/z: 312.1518 [M+H]+, 334.1338 [M+Na]+.
2-Amino-4-(2-fluorophenyl)-5,6-dihydrobenzo[h]quinoline-3-carbonitrile (4c). The compound was obtained as yellow crystals, yield 52%; mp 164-166 ℃; IR (KBr, v, cm-1): 3472, 3362 v(NH2, Ar), 3211, 2942, 2838 v(=C-H), 2213 v(CN), 1616, 1557, 1496, 1452 v(C=C, Ar), 1432 v(C=N), 1249 v(C-F), 769, 756, 741, 660 δ(C-H)cm-1; 1H NMR (400MHz, DMSO-d6) (δ, ppm): 2.44 (t, J=9.48Hz, 2H, CH2), 2.77 (t, J=8.48Hz, 2H, CH2), 6.91 (s, 2H, NH2), 7.27-7.29 (m, 1H, ArH), 7.38-7.49 (m, 5H, ArH), 7.56-7.62 (m, 1H, ArH), 8.18-8.21 (m, 1H, ArH): 13C NMR(100MHz,DMSO-d6) (δ, ppm): 23.89, 27.3, 89.1, 116.1, 118.9, 123.4, 125.0, 125.7, 126.9, 128.0, 130.4, 131.0, 131.7, 133.2, 139.2, 147.2, 154.3 , 157.3, 158.9, 159.7; HRMS(ESI) m/z: 316.1267 [M+H]+, 338.1087 [M+Na]+.
2-Amino-4-(3-fluorophenyl)-5,6-dihydrobenzo[h]quinoline-3-carbonitrile (4d). The compound was obtained as Orange crystals, yield 54%; mp 159-161 ℃; IR (KBr, v, cm-1): 3489, 3373 v(NH2,Ar), 3207, 3063, 2852 v(=C-H), 2206 v(CN), 1628, 1549, 1488 v(C=C, Ar), 1446 v(C=N), 1247v(C-F), 765, 738, 713, 698δ(C-H)cm-1; 1H NMR (400MHz, DMSO-d6) (δ, ppm): 2.60 (t, J=5.80Hz, 2H, CH2), 2.76 (t, J=7.22Hz, 2H, CH2), 6.86 (s, 2H, NH2), 7.24-7.28 (m, 2H, ArH), 7.32-7.39 (m, 4H, ArH), 7.57-7.62(m, 1H, ArH), 8.18-8.20 (m, 1H, ArH); 13C NMR (100MHz, DMSO-d6)(δ, ppm): 23.8, 27.2, 88.5, 115.7, 116.7, 118.0, 124.8, 125.6, 126.7, 127.9, 130.2, 130.7, 133.2, 138.3, 139.1, 151.6, 154.1, 158.8, 160.7, 163.1; HRMS(ESI) m/z: 316.1261 [M+H]+, 338.1087 [M+Na]+.
2-Amino-4-(4-fluorophenyl)-5,6-dihydrobenzo[h]quinoline-3-carbonitrile (4e). The compound was obtained as pale yellow crystals, yield 73%; mp 168-170 ℃; IR (KBr, v, cm-1): 3466, 3293 v(NH2, Ar), 3168, 3069, 2948 v(=C-H), 2212 v(CN), 1603, 1583, 1511, 1461v(C=C, Ar), 1430 v(C=N), 1222 v(C-F), 847, 770, 740, 668 δ(C-H)cm-1; 1H NMR (400MHz, DMSO-d6) (δ, ppm): 2.48 (t, J=13.3Hz, 2H, CH2), 2.75 (t, J=7.18 Hz, 2H, CH2), 6.82 (s, 2H, NH2), 7.36-7.41 (m, 4H, ArH), 7.45-7.49 (m, 2H, ArH), 8.18-8.20 (m, 1H, ArH); 13C NMR (100MHz, DMSO-d6)(δ,ppm): 23.9, 27.3, 88.8, 115.4, 115.7, 116.8, 118.2, 125.6, 126.7, 127.8, 130.2, 130.8, 132.3, 133.3, 139.1, 152.1, 154.1, 158.8, 161.0, 163.4; HRMS(ESI) m/z: 316.1270 [M+H]+, 338.1090 [M+Na]+.
2-Amino-4-(2-furyl)-5,6-dihydrobenzo[h]quinoline-3-carbonitrile (4f). The compound was obtained as yellow crystals, yield 70%; mp 137-139 ℃; IR (KBr, v, cm-1): 3458, 3307 v(NH2, Ar), 3196, 2933, 2836 v(=C-H), 2203 v(CN), 1633, 1605, 1541, 1480 v(C=C, Ar), 1438 v(C=N), 795, 752, 669, 593 δ(C-H)cm-1 ; 1H NMR (400MHz, DMSO-d6) (δ, ppm): 2.80 (s, 4H, CH2), 6.75-6.76 (m, 1H, ArH), 6.84 (s, 2H, NH2), 6.95 (d, J=3.22Hz, 1H ArH), 7.28-7.31 (m, 1H, ArH), 7.35-7.42 (m, 2H, ArH), 7.98 (d, J=1.36Hz, 1H ArH), 8.14-8.16 (m, 1H, ArH) ; 13C NMR (100MHz, DMSO-d6)(δ, ppm): 24.1, 27.2, 86.4, 111.7, 113.7, 117.0, 118.0, 125.6, 126.7, 127.7, 130.2, 133.2, 139.0, 140.6, 144.6, 147.1, 154.6, 159.4; HRMS(ESI) m/z: 228.1154 [M+H]+, 310.0976 [M+Na]+.
2-Amino-4-(1-naphthyl)-5,6-dihydrobenzo[h]quinoline-3-carbonitrile (4g). The compound was obtained as yellow crystals, yield 63%; mp 223-225 ℃; IR (KBr,v, cm-1): 3484, 3343 v(NH2,Ar), 3212, 3056, 2931 v(=C-H), 2220 v(CN), 1623, 1555, 1506, 1459 v(C=C, Ar), 1434 v(C=N), 793, 775, 738, 667 δ(C-H)cm-1 ; 1H NMR (400MHz, DMSO-d6) (δ, ppm): 2.12-2.28 (m, 2H, CH2), 2.68 (t, J=7.20Hz, 2H, CH2), 6.90 (s, 2H, NH2), 7.22-7.24 (m, 1H, ArH), 7.36-7.45 (m, 3H, ArH), 7.49-7.53 (t, J=6.92Hz, 2H, ArH), 7.57-7.61 (t, J=7.20Hz, 1H, ArH), 7.65-7.69 (t, J=7.66Hz, 1H, ArH), 8.06-8.09 (t,J=7.26Hz, 2H, ArH), 8.25-8.27 (m, 1H, ArH); 13C NMR (100MHz, DMSO-d6)(δ, ppm): 23.8, 27.2, 89.7, 116.6, 119.1, 124.4, 125.6, 125.7, 126.4, 126.4, 126.8, 127.2, 127.9, 128.5, 129.0, 130.0, 130.2, 133.0, 133.3, 133.7, 139.1, 151.7, 153.9, 158.9 ; HRMS(ESI) m/z: 348.1512 [M+H]+, 370.1335 [M+Na]+.
2-Amino-4-(3,4-difluorophenyl)-5,6-dihydrobenzo[h]quinoline-3-carbonitrile (4h). The compound was obtained as pale yellow crystals, yield 56%; mp 131-133 ℃; IR (KBr, v, cm-1): 3467, 3300 v(NH2,Ar), 3174, 3082, 2950 v(=C-H), 2212 v(CN), 1630, 1555, 1517, 1439 v(C=C, Ar), 1407 v(C=N), 1282, 1248 v(C-F), 826, 758, 739, 667 δ(C-H)cm-1; 1H NMR (400MHz, DMSO-d6) (δ, ppm): 2.50 (m, 2H, CH2), 2.75 (t, J=6.96Hz, 2H, CH2), 6.87 (s, 2H, NH2), 7.29-7.39 (m, 4H, ArH), 7.60-7.66 (m, 2H, ArH), 8.17-8.19 (m, 1H, ArH); 13C NMR (100MHz, DMSO-d6)(δ, ppm): 23.8, 27.2, 88.6, 116.6, 117.8, 118.0, 118.1, 118.2, 118.3, 125.6, 126.0, 126.8, 127.8, 130.3, 133.2, 139.1, 148.4, 150.8, 154.2, 158.7 ; HRMS(ESI) m/z: 334.1169 [M+H]+, 356.0994 [M+Na]+.
CALCULATIONS
The density functional theory (DFT) methods with the B3LYP hybrid functional and the 6-31G(d,p) basis set, using the Gaussian 09 package,23 have been performed to optimize the molecular structures. Based on the B3LYP/6-31G(d,p) optimized geometries, TD-DFT approaches were used to calculate vertical excitations with linear response to verify the effects of these excitations on the absorption spectra of compounds 4. The polarized continuum (overlapping spheres) solvation model (PCM) was used to ensure that solvent effects (ethanol) were incorporated into all of the aforementioned calculations.
SUPPLEMENTARY INFORMATION
The materials of the structural characterization with of HRMS (ESI), 1H NMR, 13C NMR, IR, and mp for this article is available online at the supplementary information.
ACKNOWLEDGEMENTS
Financial supports from National Natural Science Foundation of China (No. 21106069) 、China Scholarship Council and Science Foundation for Young Scientists of Nanjing University of Technology (No. 39704011) are gratefully acknowledged. The authors thank Dr. Wang xinzhi for his help with the DFT and TDDFT calculations.
References
1. S. L. Schreiber, Science, 2000, 287, 1964. CrossRef
2. M. Julian and M. Piotr, Z. Naturforsch., 1986, 41B, 1471.
3. A. Lew, P. O. Krutzik, M. E. Hart, and A. R. Chamberlin, J. Comb. Chem., 2002, 4, 95. CrossRef
4. D. Dallinger and C. O. Kappe, Chem. Rev., 2007, 107, 2563; CrossRef S. A. Galema, Chem. Soc. Rev., 1997, 26, 233; CrossRef J. Alcazar, J. Comb. Chem., 2005, 7, 353; CrossRef R. S. Varma, Green Chem., 1999, 1, 43; CrossRef A. G. Takvorian and A. P. Combs, J. Comb. Chem., 2004, 6, 171; CrossRef W. S. Bremner and M. G. Organ, J. Comb. Chem., 2007, 9, 14. CrossRef
5. C. J. Temple, G. A. Rener, W. R. Waud, and P. E. Noker, J. Med. Chem., 1992, 35, 3686. CrossRef
6. M. Larhed and A. Hallberg, Drug Discov. Today, 2001, 6, 406. CrossRef
7. I. Hermecz, L. Vasvari-Debreczy, and P. Matyus, In Comprehensive Heterocyclic Chemistry (Pergamon: London), ed. by A. R. Katritzky, C. W. Rees, and E. V. F. Scriven, 1996, Ch. 6, pp. 563-595.
8. E. J. Reinhard, S. G. Hegde, D. R. Anderson, L. F. Lee, and W. F. Vemier, PCT Int. Appl. Patent 2004, WO2004054504 A2.
9. D. R. Anderson, N. W. Stehle, S. A. Kolodziej, and E. J. Reinhard, PCT Int. Appl. Patent 2004, WO 2004055015 A1.
10. C. J. Shishoo, M. B. Devani, V. S. Bhadti, S. Ananthan, and G. V. Ullas, Tetrahedron Lett., 1983, 24, 4611. CrossRef
11. K. Deo, K. Avasthi, R. Pratap, D. S. Bhakuni, and M. N. Joshi, Indian J. Chem. B., 1990, 29B, 459.
12. P. L. Barili, G. Biagi, O. Livi, L. Mucci, and V. Scartoni, J. Heterocycl. Chem., 1987, 24, 997. CrossRef
13. A. Sakurai and H. Midorikawa, Bull. Chem. Soc. Jpn., 1968, 41, 430. CrossRef
14. J. U. Peters, S. Weber, S. Kritter, P. Weiss, A. Wallier, D. Zimmerli, M. Boehringer, M. Steger, and B. M. Leoffler, Bioorg. Med. Chem. Lett., 2004, 14, 3579. CrossRef
15. M. A. Gouda, M. A. Berghot, G. E. Abd El Ghani, and A. EL-Galil M. Khalil, Synth. Commun., 2014, 44, 297. CrossRef
16. M. Boehringer, B. M. Loeffler, J. U. Peters, C. Riemer, and P. Weiss, EP1476429 A1, 2004.
17. S. Kambe and K. Saito, Synthesis, 1980, 366. CrossRef
18. M. Li, W. S. Guo, L. R. Wen, Y. F. Li, and H. Z. Yang, J. Mol. Catal. A: Chem., 2006, 258, 133. CrossRef
19. J. Sun, L. L. Zhang, and D. M. Liu, Chinese J. Org. Chem., 2008, 28, 1616.
20. Y. X. Zhou, J. Q. Wang, R. J. Du, G. H. Zhang, W. Wang, and C. Guo, Synth. Commun., 2011, 41, 3167.
21. J. Tang, L. M. Wang, Y. F. Yao, L. Zhang, and W. B. Wang, Tetrahedron. Lett., 2011, 52, 509. CrossRef
22. V. Bernard, Molecular Fluorescence: Principles and Applications, Wiley-VCH Verlag GmbH, Weinheim, 2001.
23. M. J. Frisch, G. W. Trucks, H. B. Schlegel, G. E. Scuseria, M. A. Robb, J. R. Cheeseman, G. Scalmani, V. Barone, B. Mennucci, G. A. Petersson, H. Nakatsuji, M. Caricato, X. Li, H. P. Hratchian, A. F. Izmaylov, J. Bloino, G. Zheng, J. L. Sonnenberg, M. Hada, M. Ehara, K. Toyota, R. Fukuda, J. Hasegawa, M. Ishida, T. Nakajima, Y. Honda, O. Kitao, H. Nakai, T. Vreven, J. A. Montgomery, Jr., J. E. Peralta, F. Ogliaro, M. Bearpark, J. J. Heyd, E. Brothers, K. N. Kudin, V. N. Staroverov, T. Keith, R. Kobayashi, J. Normand, K. Raghavachari, A. Rendell, J. C. Burant, S. S. Iyengar, J. Tomasi, M. Cossi, N. Rega, J. M. Millam, M. Klene, J. E. Knox, J. B. Cross, V. Bakken, C. Adamo, J. Jaramillo, R. Gomperts, R. E. Stratmann, O. Yazyev, A. J. Austin, R. Cammi, C. Pomelli, J. W. Ochterski, R. L. Martin, K. Morokuma, V. G. Zakrzewski, G. A. Voth, P. Salvador, J. J. Dannenberg, S. Dapprich, A. D. Daniels, O. Farkas, J. B. Foresman, J. V. Ortiz, J. Cioslowski, and D. J. Fox, Gaussian, Inc., Wallingford CT, 2010.