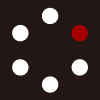
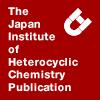
HETEROCYCLES
An International Journal for Reviews and Communications in Heterocyclic ChemistryWeb Edition ISSN: 1881-0942
Published online by The Japan Institute of Heterocyclic Chemistry
e-Journal
Full Text HTML
Received, 8th February, 2016, Accepted, 11th March, 2016, Published online, 22nd March, 2016.
■ Diverse and Important Contributions by Medicinal Chemists to the Development of Pharmaceuticals: An Example of Active Vitamin D3 Analog, Eldecalcitol
Noboru Kubodera*
International Institute of Active Vitamin D Analogs, 35-6, Sankeidai, Mishima, Shizuoka 411-0017, Japan
Abstract
Presented herein are diverse and important contributions by medicinal chemists to different stages of pharmaceutical development. The conceptual elements reviewed, which are intended for young chemists who engage in drug discovery research, draw upon the author’s experience in developing eldecalcitol, an active vitamin D3 analog used to treat osteoporosis. The review covers exploratory research for a lead candidate compound; process development for practical manufacturing; and synthesis of other compounds relevant to the program, such as tritiated compounds, postulated metabolites, and miscellaneous analogs for mode of action studies.CONTENTS
1. Introduction
2. Exploratory research for eldecalcitol
3. Process development for practical production
4. Synthesis of relevant compounds
4-1. Tritiated compounds for ADME studies
4-2. Postulated metabolites for structure determination
4-3. Miscellaneous analogs for mode of action studies
5. Conclusion
1. INTRODUCTION
Osteoporosis, a disease responsible for decreased bone strength, occurs when the rate of bone resorption outpaces the rate of bone formation. The resulting imbalance between the two processes is normally associated with advanced age. Women in particular develop the disease more frequently than men because their bone mineral density (BMD) rapidly decreases after menopause. For more than 30 years in Japan, active vitamin D3 (calcitriol 1) and its synthetic prodrug (alfacalcidol 2) have been widely used to treat osteoporosis, both agents have been considered safe and effective, producing a mild increase in BMD in osteoporotic patients.1 In contrast to the Japanese clinical practice places on active vitamin D3 therapy, American and European therapy typically involves either bisphosphonates (such as sodium alendronate, sodium risedronate and sodium ibandronate) or selective estrogen receptor modulators (SERM) (such as raloxifene hydrochloride and bazedoxifene acetate).2 These agents are typically chosen because they strongly increase BMD, which has been previously described.3 Although SERM and bisphosphonates are gaining ground as an accepted form of therapy in Japan, there is still intense interest in obtaining active vitamin D3 analogs that are more potent than calcitriol/alfacalcidol or comparable to the activity of bisphosphonates/SERM. Analogs are being sought to increase BMD and prevent bone fracture with relatively less hypercalcemic character than what is observed for calcitriol/alfacalcidol.4 One such compound—1α,25-dihydroxy-2β-(3-hydroxypropoxy)vitamin D3 (eldecalcitol 3)—is a calcitriol analog functionalized with a hydroxypropoxy substituent at the 2β-position of calcitriol’s A-ring. Eldecalcitol is a highly effective bone therapy agent.5 Phase III clinical trials comparing eldecalcitol with alfacalcidol for bone fracture prevention in osteoporotic patients yielded excellent results.6,7 In April of 2011, eldecalcitol was launched as a promising medicine for treating osteoporosis. The compound is marketed by Chugai and Taisho Pharmaceuticals under the brand name Edirol, which is now the leader for osteoporosis treatment in Japan.8
The process of discovering eldecalcitol and moving it off of the discovery bench and into the arms of clinicians involved a diverse group, including biologists, pharmacologists, clinicians, and medicinal chemists. When considering the role that medicinal chemists play in translating pharmacological agents into clinical practice, however, we often focus on early stage development activities, particularly the exploratory research that is critical for identifying an active candidate. Nevertheless, the scope of a medicinal chemist’s work is not restricted this and includes a number of key (and oftentimes overlooked) activities, such as process development of manufacturing methods for mass production; and the synthesis of compounds required to advance the biology of a translational program, such as isotope-labeled compounds, postulated metabolites, etc. The importance of such activities cannot be understated, to the extent that a deficit in any of the work will immediately hinder the development of pharmaceuticals. Consequently, the author herein presents diverse and important contributions made by medicinal chemists to various stages of pharmaceutical development, drawing upon the author’s own experience in developing eldecalcitol.9 While the discourse has been designed to engage a diverse audience, the author intentionally targets young chemists involved in drug discovery research. In many cases, the activities described below are depicted with broad strokes, since detailed reaction conditions have already been extensively described in the literature (references included below).
2. EXPLORATORY RESEARCH FOR ELDECALCITOL
During our studies to distinguish calcitriol’s in vitro differentiation inducing effects from its in vivo calcemic actions, we produced the analog 1α,25-dihydroxy-22-oxavitamin D3 (maxacalcitol 4). The side chain of maxacalcitol is modified to have potent differentiation inducing effects but weak blood calcium raising effects (calcemic actions).10-12 Maxacalcitol was released into the Japanese market as an injection for treating secondary hyperparathyroidism in 2000 and as an ointment for psoriasis vulgaris in 2001. It is now used in clinical practice and is marketed by Chugai Pharmaceuticals under the trade name Oxarol.13,14 Interestingly, an analog with an opposite biological profile (i.e. weak differentiation inducing effects but potent calcemic actions) was identified among A-ring modified derivatives (below).15
In 1981, alfacalcidol was launched in Japan (Chugai and Teijin Pharmaceuticals) as a prodrug of calcitriol.16 Scheme 1 depicts the industrial synthetic route to alfacalcidol starting with commercially available and inexpensive cholesterol (5). The 1,2α-epoxide (6) served as a key intermediate, since hydride reduction of 6 introduces alfacalcidol’s biologically important 1α-hydroxy moiety. Industrial scale production of alfacalcidol through this route not only made the compound available to the clinic, but also generated abundant quantities of compound 6 for exploratory synthetic studies. Nucleophilic
reaction of alkoxides, derived from glycols and potassium tert-butoxide (t-BuOK), with the epoxy-ring in 6 resulted in regio- and stereoselective introduction of the hydroxy-ether group at the 2β-position of 7. Compound 7 was then irradiated and thermally isomerized to 25-deoxy analog (8).17 By producing a series of modified A-rings in this way, we discovered an analog with weak in vitro differentiation inducing properties but potent calcemic actions in vivo. To examine whether this potent calcemic activity is accompanied by an effect on BMD, these derivatives were evaluated using an osteoporosis rat model (OVX rats). Compounds were administered with reduced and adjusted dosage and produced significantly greater effects than alfacalcidol on BMD. This approach was then extended to the 1,2α-epoxide (11). Compound 11, which contains hydroxy group at the 25 position, is prepared from lithocholic acid (9) via 25-hydroxycholesterol (10) by a 24-step procedure.18 Ring-opening compound 11 with alkoxide followed by irradiation/thermal isomerization produced a number of derivatives (12) of various sizes and substituents, such as those with alkoxide or alkyl groups at the 2β-position. One such derivative produced was eldecalcitol, which contains a hydroxypropoxy substituent at the 2β-position (Scheme 2). This promising candidate displayed the most intense effects in terms of increasing BMD in OVX rats,19-21 it was therefore selected for advanced studies and ultimately osteoporosis clinical treatment.
3. PROCESS DEVELOPMENT FOR PRACTICAL PRODUCTION22
Since extremely long, multistep syntheses may be challenging to implement when producing large quantities of drug substance for preclinical/clinical use, we investigated practical syntheses of eldecalcitol for industrial-scale production. As described above, eldecalcitol was initially synthesized in a linear manner in which 1,2α-epoxide (11) served as a key intermediate for incorporating the hydroxypropoxy substituent at the 2β-position (Scheme 2).17 However, this 27-step linear sequence was too long and low yielding (ca. 0.03%) for industrial scale manufacturing. We therefore developed a convergent approach based on the Trost coupling reaction,23,24 in which the A-ring fragment (14) and C/D-ring fragment (16) are coupled to produce the triene system (17) of eldecalcitol in 26% yield (Scheme 3).25-27 Fragment 14 is an ene-yne prepared from the C2 symmetrical epoxide (13) in 12 steps (10.4% overall yield), and fragment 16 is a bromomethylene compound obtained from 25-hydroxyvitamin D3 (calcifediol 15) in 4 steps (27.1% overall yield). Although the overall yield of the convergent synthesis was much better than that of the linear synthesis, complicated reaction conditions meant significant improvements were still necessary to make the approach amenable for manufacturing. Nevertheless, this convergent methodology proved quite useful for synthesizing related compounds, such as postulated metabolites and miscellaneous analogs for mode of action studies (described below).
To find an alternative to the convergent synthesis, we turned our attention to biomimetic approaches. Since 25-hydroxylation of the steroidal and secosteroidal side chain had been performed microbially,28,29 we examined such an approach in the context of preparing proeldecalcitol (19). 1,2α-Epoxide (6) was cleaved by 1,3-propanediol in the presence of t-BuOK to introduce the hydroxypropoxy group at the 2β-position, giving 18 in 29% yield. Using Amycolata autotrophica ATCC 33796 culture, hydroxylation of 18 at the 25 position was successfully carried out to afford proeldecalcitol in moderate yield (64%). This was converted to eldecalcitol by irradiation and thermal isomerization in 23% yield (Scheme 3).22 The yields for converting the steroidal framework in proeldecalcitol to the secosteroidal structure in eldecalcitol by photolysis and thermal isomerization are usually moderate, since several structural associates such as lumisterol, tachisterol, etc. are also formed. The summary of process development strategies for eldecalcitol, including the linear synthesis, convergent synthesis, and biomimetic synthesis is shown in Table 1. The biomimetic methodology was finally adopted for the practical production of eldecalcitol and has been used in industrial-scale preparation.22
4. SYNTHESIS OF RELEVANT COMPOUNDS
4-1. Tritiated compounds for ADME studies30
ADME studies involve examining a dosed compound’s behavior, namely its absorption (A), distribution (D), metabolism (M), and excretion (E). Compounds labeled with isotopes such as 3H, 14C, 35S, 125I, etc., are usually indispensable for ADME studies. There are many difficulties encountered when preparing isotope-labeled compounds—for example, limited availability of labeling sources; limited compound stability given the high energy of the label; toxicities and hazards associated with labeling reagents, etc.9 In the case of eldecalcitol, two tritiated compounds were prepared independently:30 [2β-(3-3H)]eldecalcitol (22), which was tritiated at the A-ring substituent by reduction with sodium borotritiide (NaB3H4); and [26,27-3H6]eldecalcitol (27), which was labeled at the side chain with tritiated methylmagnesium iodide (C3H3MgI),
We first undertook the synthesis of [2β-(3-3H)]eldecalcitol. Introduction of tritium into the 2β-substituent was accomplished through NaB3H4 reduction of the formyl group in aldehyde (20), which was prepared from proeldecalcitol. Treatment of 20 with 1 Ci of NaB3H4 (46.1 Ci/mmol) gave 393 mCi of the tritiated alcohol (21) in 94% yield. Compound 21 was then converted to [2β-(3-3H)]eldecalcitol by desilylation, irradiation, and thermal isomerization. The specific activity of [2β-(3-3H)]eldecalcitol was found to be 13.2 Ci/mmol (27.0 μCi/μg) (Scheme 4).30 In preliminary metabolic studies of eldecalcitol using [2β-(3-3H)]eldecalcitol, two metabolites were observed: the truncated metabolite (23) and oxidized metabolite (24) (Scheme 4). Since in both of these cases the radiolabel is lost during metabolism,31 we turned our attention to the synthesis of analog [26,27-3H6]eldecalcitol (27), which is radiolabeled at the side chain.
To synthesize [26,27-3H6]eldecalcitol, the ester (25) was prepared from lithocholic acid. Treatment of compound 25 with C3H3MgI, prepared from 19.4 mg of magnesium and 50 Ci of tritiated methyl iodide (80 Ci/mmol), gave 1.4 Ci of the alcohol (26) with a radiochemical purity of 93-96% and a specific activity of 148 Ci/mmol. Compound 26 was then converted to [26,27-3H6]eldecalcitol by desilylation, irradiation, and thermal isomerization. The specific activity of [26,27-3H6]eldecalcitol was found to be 138.0 Ci/mmol (274.4 μCi/μg) (Scheme 4).30 Since the radioactivity of [26,27-3H6]eldecalcitol is retained after in vivo administration, [26,27-3H6]eldecalcitol was used in pharmacokinetic and metabolic studies of eldecalcitol.
4-2. Postulated metabolites for structure determination32
Based on the possible metabolic pathways of candidate compounds, medicinal chemists must synthesize postulated metabolites, which are helpful when determining the structure of actual metabolites during the course of pharmacokinetic and metabolic studies. As the first step of its metabolism, calcitriol is hydroxylated at the 24 position of its side chain by CYP24A1 to produce 24-hydroxylated calcitriol.33-37 We hypothesized that the hydroxylation pathway of eldecalcitol and calcitriol would be similar and therefore synthesized 24-hydroxylated eldecalcitol in 24(S) and 24(R) forms (28 and 29 respectively) (pathway 1 in Scheme 5).38
24-Hydroxylated calcitriol is further hydroxylated at the 23 and 26 positions of the side chain and subsequently oxidized (either by CYP24A1 or CYP27A1) to a keto-alcohol, lactone (calcitriol lactone), or carboxylic acid (calcitroic acid).33-37 We recognized that the metabolic pathway of eldecalcitol might be more complicated than calcitriol because, in addition undergoing side chain metabolism, eldecalcitol could also be metabolized on the substituent at the 2β-position of the A-ring (pathway 2 in Scheme 5). In fact, in our preliminary metabolic studies of eldecalcitol, we found both the anticipated 24-hydroxylated eldecalcitol metabolites (28 and 29) and several other metabolic products that may have been produced either by metabolism of the hydroxypropoxy substituent of the 2β-position or by combined metabolism of both the side chain and 2β-position substituent (pathway 3 in Scheme 5). Therefore, we synthesized several other postulated metabolites: the methyl ester derivative (30) as an ester standard of the oxidized carboxylic acid (24) at the 2β-position; the tetraol derivative (23) as a truncated metabolite at the 2β-position; and the pentaols (31 and 32 respectively) in 24(S) and 24(R) forms,32 which arise from combined metabolism of the side chain and 2β-position. These six postulated metabolites, 28, 29, 30, 23, 31, and 32, were invaluable authentic samples that were used to elucidate the structures of eldecalcitol metabolites. The metabolic studies demonstrated that pathway 2 was the major metabolic pathway in play. This pathway involved both carboxylic acid (24), which was a biliary metabolite, and truncated tetraol (23), which was a major inactivated circulating metabolite. Pathway 1 was the minor metabolic pathway because eldecalcitol shows resistance to CYP24A1 (Scheme 5).31
4-3. Miscellaneous analogs for mode of action studies39
Although eldecalcitol clearly possessed greater biological potency than alfacalcidol/calcitriol, what remained to be described in more detail were physiological properties and the mechanism through which eldecalcitol mediated its enhanced activity toward bone. To this end, we examined the impact of structural modifications to the eldecalcitol scaffold on its fundamental biological activity by synthesizing a series of eldecalcitol-related analogs. These include epimeric analogs at the 1, 3, and 20 positions and deoxy analogs at the 1 and 25 positions:39 20-epieldecalcitol (33), 3-epieldecalcitol (34), 1-epieldecalcitol (35), 1,3-diepieldecalcitol (36), 25-deoxyeldecalcitol (37), and 1-deoxyeldecalcitol (38). While the synthetic details are not included, the convergent coupling reactions were useful methodology in this context (Figure 2).
20-Epieldecalcitol (33)40,41 According to reports, 20-epicalcitriol, a calcitriol diastereomer that possesses an inverted methyl substituent at the 20 position of the side chain, possessed remarkably enhanced biological activity compared to calcitriol.42 For example, 20-epicalcitriol was 18 times
more potent (compared to calcitriol) in inducing differentiation in human myeloid leukemia cells (HL-60).43 Furthermore, it was 50 times more effective in inhibiting the proliferation of human histiocytic lymphoma cells (U937)44; and it produced a 4.5 fold increase in the concentration of osteocalcin in human osteosarcoma cells (MG-63).45 Given these findings, we became interested in the biological properties of an analog of eldecalcitol which was epimerized at the 20 position. We synthesized 20-epieldecalcitol by convergent methodology using the Trost coupling reaction.40,41 As anticipated, 20-epieldecalcitol showed greatly enhanced activity (compared to eldecalcitol) in terms of its induction of HL-60 differentiation (122.7 times); inhibition of U937 proliferation (178.0 times); and ability to increase osteocalcin concentrations in MG-63 (198.7 times).40,41
3-Epieldecalcitol (34)46,47 It is well-known that the synthesis and secretion of parathyroid hormone (PTH) is regulated by calcitriol.48 Interestingly, during the clinical development of eldecalcitol, serum intact PTH in osteoporotic patients did not change significantly upon treatment with eldecalcitol. The reason for this lack of PTH change with eldecalcitol was unclear.49 The Brown group reported that epimerization of calcitriol at the 3 position plays a major role in hormone activation and inactivation, especially in parathyroid cells.50 They also reported that 3-epicalcitriol, an epimer of calcitriol at the 3 position, showed equipotent and prolonged activity compared to calcitriol at suppressing PTH secretion.51 Since eldecalcitol possesses a bulky hydroxypropoxy substituent at the 2 position, epimerization of eldecalcitol at the adjacent and sterically hindered 3 position may have been blocked in vivo. We hypothesized that this may be the reason why eldecalcitol showed weak potency in PTH suppression during clinical studies. To examine the impact of eldecalcitol epimerization at the 3 position on suppressing PTH production, we synthesized and evaluated the biological activity of 3-epieldecalcitol. However in our biological evaluation, 3-epicalcitriol did not suppress PTH secretion more strongly than calcitriol, although the reason was not clear. The inhibitory potency of analogs was calcitriol > eldecalcitol > 3-epicalcitriol >> 3-epieldecalcitol, and corresponds to the affinity of human recombinant vitamin D receptor (VDR).46,47 Eldecalcitol and 3-epieldecalcitol appeared to be inherently weak agents for suppressing PTH.
1-Epieldecalcitol (35)52,53 While we had not clarified the mode of action through which eldecalcitol gained enhanced bone activity over calcitriol/alfacalcidol, eldecalcitol’s enhanced biological effects might be explained by its long residency in the blood stream, which arises from its strong affinity for vitamin D binding protein (DBP) (4.2 times in comparison with calcitriol). We were therefore highly interested in an analog with strong affinity for DBP.54,55 It was reported that the epimerization of calcitriol at the 1 position remarkably enhances the affinity for DBP. The Norman group reported that 1-epicalcitriol showed a 65.7-fold increase in affinity for DBP as compared to calcitriol.56 These findings prompted our interest in the biological profile of 1-epieldecalcitol, which is epimerized eldecalcitol at the 1 position. 1-Epieldecalcitol was synthesized by the Trost coupling reaction,52,53 and as anticipated, showed enhanced affinity for DBP (1.6 times in comparison with eldecalcitol). Further in vivo biological evaluation of 1-epieldecalcitol using OVX rats were highly interesting.
1,3-Diepieldecalcitol (36)57 After completing the syntheses of 3-epieldecalcitol and 1-epieldecalcitol, and to further explore structure-activity relationships between eldecalcitol and related analogs, we focused significant attention (in terms of synthesis and biological profiling) on an epimer of eldecalcitol at both 1 and 3 positions of the A-ring: namely 1,3-diepieldecalcitol.57,58 Although 1,3-diepieldecalcitol was prepared by the convergent reaction and was expected to enhance our understanding of eldecalcitol’s mode of action, a detailed comparison of the activity of 1,3-diepieldecalcitol to eldecalcitol, 3-epieldecalcitol, and 1-epieldecalcitol remains to be clarified.57
25-Deoxyeldecalcitol (37)17 As described previously, 25-deoxyeldecalcitol was synthesized during our exploratory research for eldecalcitol to obtain an analog with weak in vitro differentiation inducing properties but potent calcemic actions in vivo. 25-Deoxyeldecalcitol was synthesized by linear methodology starting from cholesterol.17 After oral administration of 25-deoxyeldecalcitol to rats (6.25 μg/kg/day for 5 days), the plasma calcium levels in rats on a low calcium (0.003%) and vitamin D deficient diet significantly increased and reached an almost normal range.17 The structural relationship between 25-deoxyeldecalcitol and eldecalcitol corresponds to the relationship between alfacalcidol and calcitriol. Since alfacalcidol can be metabolically converted to calcitriol, we are interested in the potential analogs hydroxylation (in liver or bone) of 25-deoxyeldecalcitol at the 25 position to produce eldecalcitol, which hitherto had not been investigated.
1-Deoxyeldecalcitol (38)59 Considering the metabolic pathway and biological effects of vitamin D3 (cholecalciferol), the Nordin group suggested that calcitriol was responsible for its calcemic activity. Conversely, they suggested that the long residency of 25-hydroxyvitamin D3 (calcifediol 15) in blood (due to its strong binding to DBP) was responsible for an anabolic effect on bone, which increased BMD.60 Since the structural relationship between calcitriol and calcifediol corresponds to that between eldecalcitol and 1-deoxyeldecalcitol, we were interested in the biological action of 1-deoxyeldecalcitol: e.g. possible hydroxylation of 1-deoxyeldecalcitol to eldecalcitol in the kidney; affinity for DBP; duration in blood stream; and anabolic effect on bone. We therefore synthesized 1-deoxyeldecalcitol by the convergent methodology.59 The detailed biological action of 1-deoxyeldecalcitol in comparison with eldecalcitol has not yet been investigated.
Apart from our synthetic studies, during the course of their studies on calcitriol’s A-ring, the Kittaka group synthesized 2-epieldecalcitol (39) and 2,20-diepieldecalcitol (40) analogs with high VDR affinity.43 19-Noreldecalcitol (41) was also synthesized by the DeLuca group as an analog of their 19-norseries of calcitriol; the goal was to obtain a selective activity profile that exhibits high potency in inducing differentiation of malignant cells with very low or no bone calcification activity (Figure 3).61
There are still many challenges ahead in attempting to fully understand the mode of action of eldecalcitol. By advancing our knowledge in this arena, it will be easier to develop even more effective and sophisticated pharmaceutical products. In this regard, medicinal chemists, biologists, and physicians will continue to work together to develop safer vitamin D3 analog for osteoporosis.39
5. CONCLUSION
The various and important roles played by medicinal chemists in the pharmaceutical development of eldecalcitol have been presented. In many ways, research, development, production, and distribution of pharmaceuticals bear some analogy with an ekiden, a long-distance relay road race. The product is like the tasuki—a baton for the ekiden. In an ekiden, it does not matter if one runner runs the fastest in his own section. If there are any weaknesses throughout the race, team members must compensate; make the entire team stronger; and reach the goal in the best possible condition. The tasuki passed from the previous runner carries his sweat and heart for the ekiden, as well as his passion for the race. The author wishes the processes of creating and developing pharmaceuticals to have similar spirit, and would like to become a medicinal chemist who may only have a slight strength in the series of processes, but have ideals and dreams to make some contribution for the patients who suffer day and night.9
ACKNOWLEDGMENTS
The above-mentioned studies were conducted during the author’s employment at Chugai Pharmaceutical Co., Ltd. The author deeply acknowledges his associates for their outstanding contributions to eldecalcitol development. The author thanks Professor Susumi Hatakeyama and his associates at Nagasaki University for their helpful supports during the convergent methodology development and its applications to the synthesis of eldecalcitol and analogs. The author would like to express his sincere appreciation to Professor David A. Horne of the Department of Molecular Medicine, Beckman Research Institute at City of Hope for editing manuscript and suggestions.
References
1. R. Eastell and B. L. Riggs, ‘Vitamin D and Osteoporosis’ Vitamin D Second Edition, ed. by D. Feldman, J. W. Pike, and F. H. Glorieux, Elsevier Academic Press, Burlington, 2005, pp. 1101-1120.
2. S. E. Papapoulos, ‘Pharmacology and Use in the Treatment of Osteoporosis’ Osteoporosis, ed. by R. Marcus, D. Feldman, and J. Kelsey, Academic Press, San Diego, 1996, pp. 1209-1234.
3. Y. Nishii and T. Okano, Steroids, 2001, 66, 137. CrossRef
4. K. Eto, A. Fujiyama, M. Kaneko, K. Takahashi, J. Ishihara, S. Hatakeyama, Y. Ono, and N. Kubodera, Heterocycles, 2009, 77, 323. CrossRef
5. N. Kubodera and F. Takahashi, ‘Analogs for the Treatment of Osteoporosis’ Vitamin D Third Edition, ed. by D. Feldman, J. W. Pike, and J. S. Adams, Elsevier Academic Press, Burlington, 2011, pp. 1489-1496.
6. T. Matsumoto, M. Ito, Y. Hayashi, T. Hirota, Y. Tanigawara, T. Sone, M. Fukunaga, M. Shiraki, and T. Nakamura, Bone, 2011, 49, 605. CrossRef
7. T. Matsumoto, T. Takano, S. Yamakido, F. Takahashi, and N. Tsuji, J. Steroids Biochem. Mol. Biol., 2010, 121, 261.
8. L. Cianferotti. C. Cricelli, J. A. Kanis, R. Nuti, J.-Y. Reginster, J. D. Ringe, R. Rizzoli, and L. Brandi, Endocrine, 2015, 50, 12. CrossRef
9. N. Kubodera, J. Synth. Org. Chem. Jpn., 1996, 54, 139. CrossRef
10. N. Kubodera, J. Synth. Org. Chem. Jpn., 2005, 63, 728. CrossRef
11. N. Kubodera, Curr. Bioact. Compd., 2006, 2, 301. CrossRef
12. N. Kubodera, Mini-Rev. Med. Chem., 2009, 9, 1416. CrossRef
13. N. Kubodera, J. Synth. Org. Chem. Jpn., 2010, 68, 904. CrossRef
14. N. Kubodera, Heterocycles, 2010, 80, 83. CrossRef
15. N. Kubodera, Vitamins, 2013, 87, 95.
16. N. Kubodera, Molecules, 2009, 14, 3869. CrossRef
17. K. Miyamoto, E. Murayama, K. Ochi, H. Watanabe, and N. Kubodera, Chem. Pharm. Bull., 1993, 41, 1111. CrossRef
18. K. Miyamoto, N. Kubodera, E. Murayama, K. Ochi, T. Mori, and I. Matsunaga, Synth. Commun., 1986, 16, 513. CrossRef
19. Y. Ono, H. Watanabe, A. Shiraishi, S. Takeda, Y. Higuchi, K. Sato, N. Tsugawa, T. Okano, T. Kobayashi, and N. Kubodera, Chem. Pharm. Bull., 1997, 45, 1626. CrossRef
20. Y. Ono, A. Kawase, H. Watanabe, A. Shiraishi, S. Takeda, Y. Higuchi, K. Sato, T. Yamauchi, T. Mikami, M. Kato, N. Tsugawa, T. Okano, and N. Kubodera, Bioorg. Med. Chem., 1998, 6, 2517. CrossRef
21. N. Tsugawa, K. Nakagawa, M. Kurobe, Y. Ono, N. Kubodera, K. Ozono, and T. Okano, Biol. Pharm. Bull., 2000, 23, 66. CrossRef
22. N. Kubodera and S. Hatakeyama, Anticancer Res., 2012, 32, 303.
23. B. M. Trost and J. Dumas, J. Am. Chem. Soc., 1992, 114, 1924. CrossRef
24. B. M. Trost, J. Dumas, and M. Villa, J. Am. Chem. Soc., 1992, 114, 9836. CrossRef
25. N. Kubodera and S. Hatakeyama, Heterocycles, 2009, 79, 145. CrossRef
26. S. Hatakeyama, T. Ikeda, J. Maeyama, T. Esumi, Y. Iwabuchi, A. Kawase, and N. Kubodera, Bioorg. Med. Chem. Lett., 1997, 7, 2871. CrossRef
27. J. Maeyama, H. Hiyamizu, K. Takahashi, J. Ishihara, S. Hatakeyama, and N. Kubodera, Heterocycles, 2006, 70, 295. CrossRef
28. J. Sasaki, A. Mikami, K. Mizoue, and S. Omura, Appl. Environ. Microbiol., 1991, 57, 2841, and references cited therein.
29. K. Takeda, T. Asou, A. Matsuda, K. Kimura, K. Okamura, R. Okamoto, J. Sasaki, T. Adachi, and S. Omura, J. Ferment. Bioeng., 1994, 78, 380. CrossRef
30. H. Watanabe, A. Kawase, K. Okano, T. Mitsui, Y. Ishitani, K. Morikawa, and N. Kubodera, J. Labelled Conpd. Radiopharm., 1999, 42, 519. CrossRef
31. Y. Ono, J. Steroids Biochem. Mol. Biol., 2014, 139, 88.
32. Y. Ono, H. Watanabe, I. Taira, K. Takahashi, J. Ishihara, S. Hatakeyama, and N. Kubodera, Steroids, 2006, 71, 529. CrossRef
33. Y. Takasaki, T. Suda, S. Yamada, H. Takayama, and Y. Nishii, Biochemistry, 1981, 20, 1681. CrossRef
34. S. Yamada, M. Ohmori, H. Takayama, Y. Takasaki, and T. Suda, J. Biol. Chem., 1983, 258, 457.
35. R. Kruse, G. Popjak, J. E. Bishop, and A. W. Norman, Biochemistry, 1983, 22, 1798. CrossRef
36. N. Ikekawa and Y. Fujimoto, J. Synth. Org. Chem. Jpn., 1988, 46, 455. CrossRef
37. N. Ikekawa, Med. Res. Rev., 1987, 7, 333. CrossRef
38. S. Hatakeyama, A. Kawase, Y. Uchiyama, J. Maeyama, Y. Iwabuchi, and N. Kubodera, Steroids, 2001, 66, 267. CrossRef
39. N. Kubodera, Heterocycles, 2012, 86, 69. CrossRef
40. S. Hatakeyama, M. Yoshino, K. Eto, K. Takahashi, J. Ishihara, Y. Ono, H. Saito, and N. Kubodera, J. Steroids Biochem. Mol. Biol., 2010, 121, 25.
41. M. Yoshida, K. Eto, K. Takahashi, J. Ishihara, S. Hatakeyama, Y. Ono, H. Saito, and N. Kubodera, Heterocycles, 2010, 81, 381. CrossRef
42. L. Binderup, E. Binderup, W. O. Godtfredsen, and A.-M. Kissmeyer, ‘Development of New Vitamin D Analogs’ Vitamin D Second Edition, ed. by D. Feldman, J. W. Pike, and F. H. Glorieux, Elsevier Academic Press, Burlington, 2005, pp. 1489-1510.
43. N. Saito, Y. Suhara, M. Kurihara, T. Fujishima, S. Honzawa, H. Takayanagi, T. Kozono, M. Matsumoto, M. Ohmori, N. Miyata, H. Takayama, and A. Kittaka, J. Org. Chem., 2004, 69, 7463. CrossRef
44. L. Binderup, S. Latini, E. Binderup, C. Bretting, M. Calverley, and K. Hansen, Biochem. Pharmacol., 1991, 42, 1569. CrossRef
45. S. Ryhanen, A. Mahonen, T. Jaaskelainen, and P. H. Maenpaa, Eur. J. Biochem., 1996, 238, 97. CrossRef
46. S. Hatakeyama, S. Nagashima, N. Imai, K. Takahashi, J. Ishihara, A. Sugita, T. Nihei, H. Saito, F. Takahashi, and N. Kubodera, J. Steroid Biochem. Mol. Biol., 2007, 103, 222. CrossRef
47. S. Hatakeyama, S. Nagashima, N. Imai, K. Takahashi, J. Ishihara, A. Sugita, T. Nihei, H. Saito, F. Takahashi, and N. Kubodera, Bioorg. Med. Chem., 2006, 14, 8050. CrossRef
48. J. Silver and T. Naveh-Many, ‘Vitamin D and the Parathyroids’ Vitamin D Second Edition, ed. by D. Feldman, J. W. Pike, and F. H. Glorieux, Elsevier Academic Press, Burlington, 2005, pp. 537-549.
49. T. Matsumoto, T. Miki, H. Hagino, T. Sugimoto, S. Okamoto, T. Hirota, Y. Tanigawara, Y. Hayashi, M. Fukunaga, M. Shiraki, and T. Nakamura, J. Clin. Endocrinol. Metab., 2005, 90, 5031. CrossRef
50. A. J. Brown, C. Ritter, A. S. Weiskopf, P. Vouros, G. J. Sasso, M. R. Uskokovic, G. Wang, and G. S. Reddy, J. Cell. Biochem., 2005, 96, 569. CrossRef
51. A. J. Brown, C. Ritter, E. Slatopolsky, K. R. Muralidharan, W. H. Okamura, and G. S. Reddy, J. Cell. Biochem., 1999, 73, 106. CrossRef
52. Y. Ono, H. Watanabe, A. Kawase, N. Kubodera, T. Okano, N. Tsugawa, and T. Kobayashi, Bioorg. Med. Chem. Lett., 1994, 4, 1523. CrossRef
53. K. Eto, A. Fujiyama, M. Kaneko, K. Takahashi, J. Ishihara, S. Hatakeyama, Y. Ono, and N. Kubodera, Heterocycles, 2009, 77, 323. CrossRef
54. C. Verboven, A. Rabijns, M. De Maeyer, H. Van Baelen, R. Bouillon, and C. De Ranter, Nat. Struct. Biol., 2002, 9, 131. CrossRef
55. C. S. Ritter and A. J. Brown, J. Cell. Biochem., 2011, 112, 1348. CrossRef
56. A. W. Norman, R. Bouillon, M. C. Farach-Carson, J. E. Bishop, L.-X. Zhou, I. Nemere, J. Zhao, K. R. Muralidharan, and W. H. Okamura, J. Biol. Chem., 1993, 268, 20022.
57. A. Fujiyama, M. Kaneko, K. Takahashi, J. Ishihara, S. Hatakeyama, and N. Kubodera, Heterocycles, 2007, 71, 2263. CrossRef
58. N. Kubodera and S. Hatakeyama, Anticancer Res., 2009, 29, 3571.
59. H. Sasaki, K. Eto, K. Takahashi, J. Ishihara, S. Hatakeyama, and N. Kubodera, Heterocycles, 2011, 83, 1385. CrossRef
60. A. G. Need and B. E. C. Nordin, Bone, 2008, 42, 1021. CrossRef
61. R. R. Sicinski, K. L. Perlman, and H. F. DeLuca, J. Med. Chem., 1994, 37, 3730 CrossRef