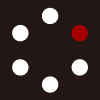
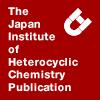
HETEROCYCLES
An International Journal for Reviews and Communications in Heterocyclic ChemistryWeb Edition ISSN: 1881-0942
Published online by The Japan Institute of Heterocyclic Chemistry
e-Journal
Full Text HTML
Received, 24th September, 2016, Accepted, 28th October, 2016, Published online, 16th November, 2016.
■ Synthesis of β-Glycosyl Formamides Through N-Glycosylation of Unprotected Carbohydrates
Yoshiyasu Ichikawa,* Akihito Matsukawa, Mitsutoshi Maeda, Yumiko Tomita, Rika Mimura, Ayumi Kitamori, Hiyoshizo Kotsuki, Keiji Nakano, and Toshiya Masuda
Faculty of Science, Kochi University, 2-5-1, Akebono-cho, Kochi 780-8520, Japan
Abstract
Two new synthetic routes involving N-glycosylation of unprotected carbohydrates were developed for the preparation of β-glycosyl formamides. Using this protocol, D-glucose, D-xylose, D-galactose and D-maltose were transformed to their corresponding β-glycosyl formamides.introduction
Glycosyl isocyanides are an important class of substances due to their wide applicability to the synthesis of biologically active compounds. For example, oxidation of glycosyl isocyanides generates highly reactive glycosyl isocyanates, which react with amines to afford glycosyl ureas.1 This methodology has been successfully employed in the total synthesis of the amino sugar antibiotic, glycocinnasperimicin D.2 In addition, a unique approach has been developed for the synthesis of N-linked glycopeptides that relies on the use of reactions of oligosaccharide isocyanides with peptide thioacetic acids.3 Moreover, an interesting process, which utilizes the Passerini and Ugi reactions of glycosyl isocyanides, has been utilized for construction of a glycoconjugate library.4 Finally, helical polymers have been synthesized by using glycosyl isocyanide as monomeric units.5
Although glycosyl isocyanides can be conveniently prepared by dehydration reactions of glycosyl formamides, the synthesis of glycosyl formamides is rather tedious owing to the need for multistep sequences involving extensive handling of protecting groups and the requirement for activation of anomeric carbons. The most widely used method for the synthesis of glycosyl formamides begins with glycosyl azides, which are prepared by displacement reactions of the protected glycosyl halides with azide anion or Lewis acid-catalyzed reaction of glycosyl acetates with trimethylsilyl azide. Because of the tendency of glycosylamines to undergo dimerization, hydrolysis and isomerization, glycosyl azides are carefully reduced to the corresponding glycosylamines, which are immediately formylated to produce the corresponding glycosyl formamides. As a result of these limitations as well as the need to use expensive reagents, a more efficient and economical synthetic method for the preparation of glycosyl formamides is highly needed. As part of our continuing research endeavor aimed at the development on N-glycosylation reactions of unprotected carbohydrates, we have devised two new synthetic routes for the preparation of glycosyl formamides. The first approach was inspired by the results of our previously reported studies of the synthesis of β-glycosyl ureas (Scheme 1).6 In this effort, we found that unprotected D-glucose (1) reacts with a variety of N-alkyl ureas to form the corresponding β-glucosyl ureas 2. Considering the structural analogy between urea and formamide, we felt that formamide would undergo N-glycosylation reaction with unprotected D-glucose to generate β-glucosyl formamide 3.
The second approach to the preparation of glycosyl formamides stems from a protocol for amination of unprotected carbohydrates reported by Likhosherstov (Scheme 2).7 In this process, unprotected carbohydrates A react with ammonia, generated by dissociation of ammonium carbamate in methanol, to produce the corresponding β-glycosylamines B, which are precipitated as carbamic acid salts C under the reaction conditions. We envisioned that selective formylation of the amine moiety in C followed by acetylation of the hydroxyl groups would efficiently produce β-glycosyl formamides D.
During the course of our studies exploring the methods for synthesis of glycosyl formamides described above, Dömling and his co-workers reported the synthesis of glycosyl formamides by reaction of fromamide with protected carbohydrates.4b This publication prompted us to describe the results of our recent investigations aimed at exploring the strategy for synthesis of glycosyl formamides through the N-glycosylation process of unprotected carbohydrates.
RESULTS AND DISCUSSION
Initial experiments to examine the reaction of D-glucose with formamide were carried out using the classical conditions employed for the synthesis of glucosyl urea8 (Scheme 3). Accordingly, treatment of D-glucose with formamide and 5% aqueous H2SO4 at room temperature for 75 days followed by acetylation and purification gave β-glucosyl formamide 3 in 5% yield. Encouraged by this promising result, we carried out several experiments in which the acid catalysts (HCl, phosphoric acid, CSA and acidic resins), amount of water, a range of temperature and time were varied. This effort led to the observation that reaction of D-glucose using Dowex 50W-X8 as the acid catalyst in a 2:1 (v/v) solution of formamide and water at 60 °C for 10 days, followed by acetylation, resulted in formation of β-glucosyl formamide 3 in a 40–42% yield.1b, 9 1H NMR analysis of the crude product mixture demonstrated that none of the α-isomer of 3 was generated.10 While the yield of this process is only moderate, the two-step synthesis of β-glucosyl formamide 3 starting with D-glucose represents the first example of a variant of Fischer’s glycosylation of formamide using unprotected carbohydrates.11 Moreover, the β-selectivity observed in this reaction suggests that the formamide group does not display an anomeric effect and, as a result, under the thermodynamically controlled reaction conditions the more stable β-glucosyl formamide 3 is generated.
In the course of studies exploring the second approach to the preparation of glycosyl formamide from D-glucose using the Likhosherstov method, we found that use of excess ammonium carbamate is crucial to obtain reproducible results in gram-scale synthesis (Scheme 4). Amination of D-glucose (1) was carried out with excess ammonium carbamate (ca. 7 equiv.) in methanol at 40 °C for 24 h. The glucosylamine salt 4, precipitated as a white solid, was conveniently isolated by simple filtration. Finally, selective N-formylation of 4 with acetic formic anhydride in DMF and O-acetylation with acetic anhydride in pyridine furnished β-glucosyl formamide 3 in 62% yield. Note that 1H NMR analysis showed that none of the corresponding α-anomer was produced. Importantly, this newly developed three-step sequence enables synthesis of β-glucosyl formamide 3 in gram quantities.
The synthetic method developed in Scheme 4 was extended to the synthesis of β-xylosyl formamide 6 starting with D-xylose 5 (Scheme 5). This process successfully generated β-xylosyl formamide 6 in 48% yield. Here again, 1H NMR analysis of the crude product mixture demonstarted that none of the α-isomer was produced.12 This β-selective synthesis of β-xylosyl formamide 6 stands in sharp contrast to a previously reported synthesis of 6 in which a mixtuer of β- and α-anomers (84:16) was formed.13
In order to extend the scope of the newly developed glycosyl formamide synthetic method described above, we further examined its application to the preparation of galactosyl formamide (Scheme 6). To our surprise, we obsreved that the processes produced the desired galactosyl formamide 10 along with unidentified by-products that made purification difficult even after repeating chromatography and recrystallization. 1H NMR analysis showed that the by-product display a number of small peaks around δ 8.00 ppm, suggesting that partial O-formylation of hydroxy groups in 9 occurred during N-formylation reaction of 8. After extensive experimentation, conditions to suppress by-product formation was developed to give rise to 10 in a 68% yield. Specifically, the improved conditions involve treating formamide 9 with triethylamine and water before the acetylation step. This intervening process presumably hydrolyze the formed formate esters of 9.
The improved method developed in Scheme 6 was successfully applied to the synthesis of -maltosyl formamide 12 (Scheme 7). In this case, formamide 12 was isolated in 61% yield.
Since β-glycosyl formamides, 3, 6, 10 and 12 exist as mixtures of (Z)-and (E)-rotamers, their analysis by using NMR spectroscopy is complicated. Thus, in order to check their homogeneities thorough 1H and 13C NMR spectroscopic analysis, we transformed each β-glycosyl formamide into its corresponding β-glycosyl isocyanide. For this purpose, dehydration reactions of β-glycosyl formamides 3, 6, 10 and 12, were performed by treatment with triphosgene and triethylamine in CH2Cl2 at –20 °C to generate the corresponding β-glycosyl isocyanides 13, 14, 15 and 16 in the yields given in Table 1.14 Inspection of the 1H and 13C NMR spectra of the β-isocyanides confirmed their homogeneities.
Conclusions
The method developed for the synthesis of glycosyl formamides in the study described above involves direct N-glycosylation of unprotected carbohydrates. The procedure, which starts with simple and inexpensive starting materials, is a simple, reliable and efficient synthetic route for the preparation of β-glycosyl formamides.
EXPERIMENTAL
Melting points were recorded on a micro melting point apparatus and are not corrected. Optical rotations were measured at the sodium D line with a 100 mm path length cell, and are reported as follows: [α]DT, concentration (g/100 mL), and solvent. Infrared spectra are reported in wave number (cm–1). 1H NMR data are reported with the solvent resonance as the internal standard relative to chloroform (δ 7.26) as follows; chemical shifts (δ), multiplicity (s = singlet, d = doublet, t = triplet, q = quartet, br = broadened, m = multiplet), coupling constants (J, given in Hz) and integrations. 13C NMR chemical shifts (δ) are recorded in parts per million (ppm) relative to CDCl3 (δ 77.0) and CD3OD (δ 49.0) as internal standards. Each glycosyl formamide, 3, 6, 10, and 12 exists as a mixture of amide rotamers on the 1H and 13C NMR time scale. When two rotamers are observed, signals for the minor rotamers are given in braces. In the 13C NMR spectrum, anomeric carbon resonances of glycosyl fromamides appear around 77 ppm and often overlap with the resonance of CDCl3. To avoid this inconvenience, 13C NMR spectra were recorded using CD3OD as solvent which has no signals around 77 ppm. High-resolution mass spectra (HRMS) are reported in m/z. Reactions were run under an atmosphere of argon when the reactions were sensitive to moisture or oxygen. Dichloromethane was dried over molecular sieves 3 Å. Pyridine and triethylamine were stocked over anhydrous KOH. All other commercially available reagents were used as received.
Synthesis of β-D-glucosyl formamide 3 using reaction conditions in Scheme 3. To a solution of D-glucose (500 mg, 2.80 mmol) dissolved in a mixture of formamide (2.50 mL) and water (1.25 mL) was added Dowex 50W-X8 (2.0 g). The resulting mixture was heated at 60 °C for 10 days. The resulting brown reaction mixture was filtered and filtrated Dowex 50W-X8 was washed with MeOH. The combined filtrate was concentrated under vacuum, and the resulting residue was dissolved in a mixture of acetic anhydride (13.5 mL) and pyridine (27.0 mL). The solution was stirred at room temperature overnight and then heated at 70 °C for 20 min. Water (80 mL) was added and stirring was continued for 20 min. The solution was extracted with AcOEt (30 mL, × 3), and the combined organic layers were washed with aqueous 1 M KHSO4 (30 mL), water (30 mL), aqueous saturated NaHCO3 (30 mL) and brine (20 mL). After drying (Na2SO4), concentration under reduced pressure gave a residue (896 mg), which was subjected to silica gel chromatography (AcOEt/hexane 2:1) to yield 3 (434 mg, 42%) as a white solid: mp 142–143 °C (recrystallized from AcOEt/hexane) (lit.9a 147–148 °C; lit.9b146–148 °C); [α]D24 +20.8 (c 1.00, CHCl3) (lit.9a [α]578 +21, c 0.5, CHCl3; lit.9b [α]D20 +20.7, c 0.5, CHCl3); IR (KBr) νmax 3368, 1742, 1686 cm-1 [lit.9a ν(KBr) 3340m, 1725s, 1665m, 1490m; lit.9b ν(KBr) 3400, 1750, 1700]; 1H NMR (500 MHz, CDCl3) α 2.01 (s, 3H), 2.03 (s, 3H), {2.04 (s)}, {2.05 (s)}, 2.06 (s, 3H), 2.08 (s, 3H), {2.09 (s)}, {3.79 (ddd, J = 10.3, 4.6, 2.3 Hz)}, 3.83 (ddd, J = 10.2, 4.6, 2.3 Hz, 1H), 4.08 (dd, J = 12.6, 2.3 Hz, 1H), {4.27 (dd, J = 12.0, 4.6 Hz)}, 4.31 (dd, J = 12.6, 4.6 Hz, 1H), {4.70 (t, J = 9.7 Hz)}, 4.95 (t, J = 9.5 Hz, 1H), {4.98 (t, J = 9.2 Hz)}, 5.06 (t, J = 9.7 Hz, 1H), {5.08 (t, J = 9.7 Hz)}, 5.31 (t, J = 9.5 Hz, 1H), 5.32 (t, J = 9.5 Hz, 1H), {6.27 (t, J = 10.6 Hz)}, 6.41 (d, J = 9.7 Hz, 1H), {8.19 (d, J = 10.6 Hz)}, 8.21 (s, 1H); [lit.9a (200 MHz, CDCl3, Z-isomer) δ 2.02, 2.06, 2.08, 2.10 (each 3H, each s, 4 × OAc), 3.85 (1H, ddd, 5-H), 4.10 (1H, dd, J5,6’ = 2.1 Hz, 6-H’), 4.35 (1H, dd, J5,6 = 4.8, J6,6’ = 13.1 Hz, 6-H), 4.98 (1H, t, 2-H), 5.08 (1H, t, J4,5 = 9.6 Hz, 4-H), 5.33 (1H, t, J1,2 = 9.6 Hz, 1-H), 5.36 (1H, t, J2,3 = J3,4 = 9.6 Hz, 3-H) , 6.51 (1H, d, JNH,1 = 9.3 Hz, NH), 8.26 (1H, s, JNH,CHO ~ 0 Hz, CHO); (200 MHz, CDCl3, E-isomer) δ 2.02–2.11 (12H, 4 × OAc), 3.81 (1H, ddd, 5-H), 4.12 (1H, dd, J5,6’ = 2.1 Hz, 6-H’), 4.28 (1H, dd, J5,6 = 4.8 Hz, J6,6’ = 13.1 Hz, 6-H), 4.73 (1H, t, J1,2 = 9.6 Hz, 1-H), 5.02 (1H, t, 2-H), 5.10 (1H, t, J4,5 = 9.6 Hz, 4-H), 5.31 (1H, t, J2,3 = J3,4 = 9.5 Hz, 3-H), 6.42 (1H, t, JNH,1 = 10.5 Hz, NH), 8.22 (1H, d, JNH,CHO = 10.5 Hz, CHO); lit.9b (200 MHz, CDCl3, ppm): δ 2.03, 2.04, 2.07, 2.09 (s, 3H, 4 COCH3), 3.83 (ddd, J5,6a= 2.2 Hz, J5,6b = 4.5 Hz, J5,4 = 9.5 Hz, 1H, 5-H), 4.09 (dd, J6a,5 = 2.2 Hz, J6a,6b = 12.5 Hz, 1H, 6a-H), 4.30 (dd, J6b,5 = 4.5 Hz, J6b,6a = 12.5 Hz, 1H, 6b-H), 4.94 (t, J2,1 = J2,3 = 9.5 Hz, 1H, 2-H), 5.07 (t, J4,5 = J4,3 = 9.5 Hz, 1H, 4-H), 5.31 (t, J1,2 = J1,NH = 9.5 Hz, 1H, 1-H), 5.32 (t, J3,2 = J3,4 = 9.5 Hz, 1H, 3-H), 6.32 (d, JNH,1 = 9.5 Hz, 1H, NH), 8.25 (s, 1H, CHO).]; 13C NMR (CDCl3, 125 MHz) δ 20.51, 20.54, 20.6, 20.7, 61.5, {61.6}, {67.8}, 68.0, {70.0}, 70.3, {72.4}, 72.5, 73.7, 76.5, {81.9}, 161.1, {163.9}, {169.4}, 169.5, 169.8, {170.0}, {170.55}, 170.59, 170.9; 13C NMR (CD3OD, 125 MHz) δ 20.5, 20.57, 20.59, 20.62, 63.1, {63.2}, {69.4}, 69.5, {71.8}, 71.9, {74.53}, {74.57}, 74.65, 74.72, 77.3, {83.0}, 164.0, {167.3}, {171.0}, {171.22}, 171.24, 171.28, 171.52, {171.56}, 172.3; [lit.9a (50 MHz, CDCl3, Z-isomer) δ 20.5, 20.6, 20.7, (OCOCH3), 61.7 (C-6), 68.1 (C-4), 70.4 (C-2), 72.7, 73.7 (C-3, -5), 76.5 (C-1), 161.2 (NHCO), 169.4, 169.6, 170.7, 170.9 (OCOCH3); (50 MHz, CDCl3, E-isomer) δ 20.5–20.7 (OCOCH3), 61.8 (C-6), 68.0 (C-4), 70.1 (C-2), 72.6, 73.7 (C-3, -5), 81.9 (C-1), 163.6 (NHCO), 169.4–170.9 (OCOCH3); lit.9b (50 MHz, CDCl3): δ 20.4, 20.5, 20.6, 20.7 (COCH3), 61.3 (C-6), 67.9 (C-4), 70.3 (C-2), 72.7 (C-3), 73.7 (C-5), 76.6 (C-1), 161.0 (NHCO), 169.4, 169.5, 170.1, 170.7 (COCH3)]. HRMS (ESI): m/z calcd for C22H33N2O12 [M+H]+ 517.2028, found 517.2025.
Synthesis of β-D-glucosyl formamide 3 using reaction conditions in Scheme 4. A solution of D-glucose (5.00 g, 27.8 mmol) and finely divided ammonium carbamate (15.7 g, 201 mmol) dissolved in MeOH (160 mL) was stirred at 40 °C for 24 h. The solution was cooled at –20 °C for 2 h. The white precipitate containing β-D-glucopyranosylammonium carbamate was isolated by filtration, washed with a small amount of MeOH and Et2O, dried under vacuum for 10 min and suspended in DMF (80 mL). To this suspension was added acetic formic anhydride (9.0 mL) to result in a homogeneous solution. After stirring at room temperature overnight, the solution was concentrated under reduced pressure to give crude glucosyl formamide (9.73 g). The crude glucosyl formamide was dissolved in a mixture of acetic anhydride (25 mL) and pyridine (50 mL). After stirring at room temperature overnight, water (150 mL) was added, and stirring was continued for 1 h. The reaction mixture was extracted with AcOEt (50 mL, × 3), and the combined organic layers were washed with aqueous 1 M KHSO4 (340 mL), water (40 mL) and brine (30 mL). After drying (Na2SO4), concentration under reduced pressure afforded crude product (8.68 g), which was subjected to recrystallization from a hot mixture of AcOEt (8 mL) and Et2O (40 mL) to furnish 3 as white crystals (5.88 g, 56%). Concentration of the mother liquor gave a residue (2.22 g), which was purified by silica gel chromatography (AcOEt/hexane 1:1 to 3:2) to give additional 3 (662 mg, 6.3%, total yield 62%).
Synthesis of β-D-xylosyl formamide 6. D-Xylose (2.00 g, 13.3 mmol) and finely divided ammonium carbamate (7.00 g, 89.7 mmol) was dissolved in MeOH (70 mL) and heated at 40 °C for 24 h. The solution was cooled at –20 °C for 2 h. The precipitated white solids were filtered, washed with a small amount of MeOH and Et2O, dried under vacuum for 10 min. The crude product (4.94 g) was suspended in DMF (30 mL). To this suspension was added acetic formic anhydride (4.3 mL). A slightly exothermic reaction was observed to afford the homogeneous solution. After stirring at room temperature overnight, the solution was concentrated under reduced pressure to give crude residue, which was dissolved in a mixture of acetic anhydride (12 mL) and pyridine (20 mL). After stirring at room temperature overnight, water (50 mL) was added, and stirring was continued for 1 h. The reaction mixture was extracted with AcOEt (× 3), and the combined organic layers were washed with aqueous 1 M KHSO4, water, saturated aqueous NaHCO3 and brine. After drying (Na2SO4), concentration under reduced pressure afforded crude product (2.86 g), which was purified by silica gel chromatography (AcOEt/hexane 1:1) to furnish 6 (1.93 g, 6.3%, 48%): mp 75–76 °C; [α]D24 +28.3 (c 1.00, CHCl3); IR (KBr) νmax 3330, 3026, 2944, 2873, 1747, 1704, 1525, 1224 cm-1; 1H NMR (CDCl3, 500 MHz) δ 2.02 (s, 3H), 2.03 (s, 3H), {2.04, s}, 2.05 (s, 3H), {3.38 (t, J = 11.5 Hz)}, 3.43 (t, J = 11.5 Hz, 1H), 4.07 (dd, J = 11.5, 5.5 Hz, 1H), {4.11 (dd, J = 11.5, 5.5 Hz)}, {4.62 (t, J = 9.5 Hz, H-1)}, 4.91 (t, J = 9.5 Hz, 1H), 4.94–5.04 (m, 1H), 5.23 (t, J = 9.5 Hz, 1H, H-1), 5.29 (t, J = 9.5 Hz, 1H), {6.63 (t, J = 11.5 Hz, N-H)}, 6.74 (d, J = 9.5 Hz, N-H), {8.16 (d, J = 11.5 Hz, CHO)}, 8.20 (s, CHO, 1H); 13C NMR (CDCl3, 125 MHz) δ 20.5, 20.58, 20.61, {64.34}, 64.48, {68.49}, 68.8, {70.3}, 70.6, {72.0}, 72.17, 76.95 (C-1), {82.4 (C-1)}, 161.4, {164.2}, {169.76}, 169.79, 169.88, {169.94}, {170.0}, 170.9; 13C NMR (CD3OD, 125 MHz) δ 20.49, 20.53, 20.60, {65.3}, 65.4, {70.1}, 70.3, {71.93}, 72.01, {74.20}, 74.28, 78.0 (C-1), {83.7, (C-1)}, 164.1, {167.3}, {171.1}, 171.3, {171.46}, 171.51, 171.58, {171.63}. HRMS (ESI): m/z calcd for C12H17NO8 [M+H]+ 304.1027, found 304.1028.
Synthesis of β-D-galactosyl formamide 10. A solution of D-galactose (1.00 g, 5.56 mmol) and ammonium carbamate (2.30 g, 29.5 mmol) dissolved in MeOH (30.0 mL) was heated at 40 °C for 24 h, and cooled at –20 °C for 2 h. The precipitated white solids were filtered, washed with a small amount of MeOH and Et2O, dried under vacuum for 10 min. The crude product (1.49 g) was suspended in DMF (15.0 mL) and then treated with acetic formic anhydride (1.50 mL). After stirring for 30 min, the reaction mixture became homogeneous solution, which was stirred overnight and concentrated under reduced pressure to afford crude formamide as white solids. These white solids were suspended in a mixture of MeOH (10 mL), water (2.0 mL) and triethylamine (1.0 mL). The reaction mixture was stirred overnight and the solvent was removed by evaporation. The resulting white solids were dissolved in a mixture of acetic anhydride (5.0 mL) and pyridine (10.0 mL). After stirring at room temperature overnight, the reaction mixture was diluted with water and extracted with ethyl acetate (× 3). The combined organic layers were washed with aqueous 1 M KHSO4, water, saturated aqueous NaHCO3 and brine. After drying (Na2SO4), concentration under reduced pressure afforded crude product (1.74 g), which was purified by silica gel chromatography (AcOEt/hexane 2:1) to furnish 10 (1.41 g, 68%) as white solids: mp 174–175 °C; (lit.9a 167–169 °C); [α]D26 +35.7 (c 1.00, CHCl3); (lit.9a [α]578 +35, c 0.5, CHCl3); IR (KBr) νmax 3271, 3225, 3059, 1760, 1677, 1551, 1226 cm-1; [lit.9a ν(KBr) 3230m, 3180m, 1730s, 1660m, 1530m]; 1H NMR (CDCl3, 500 MHz) δ 1.939 (s, 3H), {1.943 (s)}, 1.99 (s, 3H), {2.00 (s)}, 2.01 (s, 3H), 2.10 (s, 3H), {2.11 (s)}, 3.97–4.10 (3H), {4.65 (t, J = 10.0 Hz), H-1}, 5.05–5.15 (2H), 5.27 (t, J = 8.5 Hz 1H, H-1), 5.39 (d, J = 2.5 Hz, 1H), {6.55 (t, J = 10.0 Hz)}, 6.66 (d, J = 9.0 Hz, 1H), {8.15 (d, J = 10.0 Hz)}, 8.16 (s, 1H); [lit.9a (200 MHz, CDCl3, Z-isomer) δ 2.01, 2.06, 2.08, 2.16 (each 3H, each s, 4 × OAc), 3.97–4.17 (3H, m, 5-H and 6-H2), 5.12–5.17 (2H, m, 2- and 3-H), 5.31 (1H, t, J1,2 = 8.3 Hz, 1-H), 5.45 (1H, d, J3,4 = 0.5 Hz, J4,5 ~ 0 Hz, 4-H), 6.41 (1H, d, JNH,1 = 8.3 Hz, NH), 8.23 (1H, s, CHO)]; [lit.9a (200 MHz, CDCl3, E-isomer) δ 2.0–2.2 (12H, 4 × OAc), 3.97–4.17 (3H, m, 5-H and 6-H2), 4.67 (1H, t, J1,2 = 9.3 Hz), 5.12–5.41 (2H, m, 2- and 3-H), 5.45 (1H, d, 4-H), 6.23 (1H, t, JNH,1 = 9.2 Hz, NH), 8.20 (1H, d, JNH,CHO = 9.2 Hz, CHO)]; 13C NMR (CDCl3, 125 MHz) δ 20.37, 20.44, 20.51, 20.58, 61.04, {61.1}, {66.8}, 67.0, {67.6}, 68.0, {70.60}, 70.65, {72.27}, 72.38, 76.67 (C-1), {82.2, C-1}, 161.5, {164.0}, 169.6, {169.7}, 169.9, {170.0}, 170.3, 170.1; 13C NMR (CD3OD, 125 MHz) δ 20.49, 20.51, 20.53, 20.57, 62.6, {62.7}, {68.8}, 68.9, {69.4}, 69.5, {72.75}, 72.79, {73.4}, 73.6, 77.5, {83.4}, 164.0, {167.3}, {171.2}, 171.38, {171.42}, 171.5, 171.9, 172.1; [lit.9a (50 MHz, CDCl3, Z-isomer) δ 20.5, 20.6, 20.7 (OCOCH3, 61.1 (C-6), 67.1 (C-4), 68.1 (C-2), 70.7, 72.5 (C-3, -5), 76.9 (C-1), 160.9 (NHCO), 169.9, 170.0, 170.4, 171.3 (OCOCH3); (50 MHz, CDCl3, E-isomer)δ 20.5–20.7 (OCOCH3), 61.2 (C-6), 66.8 (C-4), 67.6 (C-2), 70.7, 72.4 (C-3, -5), 82.3 (C-1), 163.9 (NHCO), 169.9–171.3 (OCOCH3)). HRMS (ESI): m/z calcd for C15 H22NO10 [M+H]+ 376.1238, found 376.1238.
Synthesis of β-D-maltosyl formamide 12. A solution of D-maltose monohydrate (1.00 g, 2.78 mmol) and ammonium carbamate (1.40 g, 17.9 mmol) dissolved in MeOH (15.0 mL) was heated at 40 °C for 24 h, and cooled at –20 °C for 2 h. The precipitated white solids were filtered, washed with a small amount of MeOH and Et2O, dried under vacuum for 10 min. The crude product was suspended in DMF (9.0 mL) and then treated with acetic formic anhydride (0.90 mL, 11.3 mmol). After stirring at room temperature overnight, the reaction mixture was concentrated under reduced pressure to afford crude formamide as white solids. These white solids were suspended in a mixture of MeOH (10 mL), water (1.0 mL) and triethylamine (1.0 mL). After stirring overnight, the solvent was removed by evaporation. The resulting white solids were dissolved in a mixture of acetic anhydride (4.0 mL) and pyridine (8.0 mL). After stirring at room temperature overnight, the reaction mixture was diluted with water and extracted with AcOEt (× 3), and the combined organic layers were washed with aqueous 1 M KHSO4, water, saturated aqueous NaHCO3 and brine. After drying (Na2SO4), concentration under reduced pressure afforded crude product (1.46 g). NMR analysis of the crude products showed that acetylation was uncompleted. Further acetylation of the crude products using the similar conditions described before gave the crude product (1.54 g), which was purified by silica gel chromatography (AcOEt/hexane 2:1 to 4:1) to furnish 12 (1.19 g, 61%) as an oil: [α]D30 +85.0 (c 1.00, CHCl3); IR (KBr) νmax 3360, 2962, 1750, 1524, 1433, 1048 cm-1; 1H NMR (CDCl3, 500 MHz) δ 2.00 (s, 3H), 2.02 (s, 6H), 2.03 (s, 3H), {2.05 (s)}, 2.06 (s, 3H), 2.10 (s, 3H), 2.13 (s, 3H), {2.14 (s)}, 3.76–3.83 (m, 1H), 3.90–3.97 (m, 1H), 3.97 (t, J = 10.0 Hz, 1H), 4.05 (dd, J = 12.0, 2.0 Hz, 1H), 4.20–4.26 (m, 2H), 4.44 (dd, J = 12.0, 2.0 Hz, 1H), {4.73 (t, J = 10.0 Hz, H-1)}, 4.78 (t, J = 10.0 Hz, 1H), 4.86 (dd, J = 10.0, 4.0 Hz, 1H), 5.06 (t, J = 10.0 Hz, 1H), 5.32–5.42 (m, 4H), {6.03 (t, J = 10.0 Hz)}, 6.18 (d, J = 10.0 Hz, 1H), {8.18 (d, J = 10.0 Hz)}, 8.20 (s, 1H); 13C NMR (CD3OD, 125 MHz) δ 20.57, 20.60, 20.68, 20.8, 21.2, 63.1, 64.31, {64.34}, 69.6, 69.84, {69.88}, {70.65}, 70.68, {71.60}, 71.63, {72.4}, 72.6, {74.7}, 74.8, {75.2}, 75.3, 76.9, 77.0, {82.6}, 97.2, 163.9, {167.2}, {171.14}, 171.16, {171.22}, 171.4, 171.50, {171.54}, 171.67, {171.74}, {171.93}, 171.98, 172.2. HRMS (ESI): m/z calcd for C27H38NO18 [M+H]+ 664.2083, found 664.2084.
Typical procedure for the synthesis of β-D-glycosyl isocyanides: synthesis of β-D-galactosyl isocyanide 15. To a solution of formamide 10 (0.50 g, 1.33 mmol) and triethylamine (1.50 mL, 10.6 mmol) dissolved in CH2Cl2 (10 mL) cooled to –20 °C was added a solution of triphosgene (0.79 g, 2.66 mmol) in CH2Cl2 (4.0 mL) dropwise. After stirring at –20 °C for 3 h, the reaction mixture was poured into ice-cooled solution of NaHCO3 (8.50 g) dissolved in water (100 mL), and vigorously stirred at 0 °C for 30 min. The separated aqueous layer was extracted with CH2Cl2 (× 3). The combined organic extracts were dried (Na2SO4), and concentrated under reduced pressure. The resultant residue was purified by silica gel chromatography (AcOEt/hexane 1:1) to afford galactosyl isocyanide 13 (378 mg, 79%) as a white solid: mp 171–172 °C; [α]D27 +29.2 (c 1.00, CHCl3); IR (KBr) νmax 2985, 2152, 1744, 1378, 1220 cm-1; 1H NMR (CDCl3, 500 MHz) δ 1.99 (s, 3H), 2.06 (s, 3H), 2.14 (s, 3H), 2.18 (s, 3H), 3.96 (brt, J = 6.5 Hz, 1H), 4.14 (d, J = 6.5 Hz, 2H), 4.78 (d, J = 9.5 Hz, 1H, H-1), 4.98 (dd, J = 9.5, 3.5 Hz, 1H), 5.40 (d, J = 3.5 Hz, 1H), 5.41 (t, J = 9.5 Hz, 1H); 13C NMR (CDCl3, 125 MHz) δ 20.41, 20.48, 20.52, 20.58, 61.1, 66.5, 68.3, 70.1, 73.4, 79.8 (C-1), 164.1, 168.9, 169.8, 169.9, 170.3. HRMS (ESI): m/z calcd for C15H19NNaO9 [M+H]+ 380.0952, found 380.0952.
β-D-Maltosyl isocyanide 16. Starting with 12 (797 mg, 1.20 mmol), triethylamine (1.80 mL, 12.9 mmol), CH2Cl2 (2.0 mL) and a solution of triphosgene (269 mg 12.6 mmol, dissolved in 3.0 mL of CH2Cl2), β-maltosyl isocyanide 16 (596 mg, 83%) was obtained as an oil: [α]D30 +65.1 (c 1.00, CHCl3); IR (KBr) νmax 2963, 2146, 1751, 1434, 1371, 1227, 1037 cm-1; 1H NMR (CDCl3, 500 MHz) δ 1.99 (s, 3 H), 2.01 (s, 6H), 2.02 (s, 3H), 2.08 (s, 3H), 2.09 (s, 3H), 2.16 (s, 3H), 3.73 (ddd, J = 9.5, 4.5, 2.5 Hz, 1H), 3.93 (brd, J = 10.0 Hz, 1H), 4.02 (t, J = 9.5, 1H), 4.04 (dd, J = 12.0, 2.0 Hz, 1H), 4.20 (dd, J = 12.0, 4.5 Hz, 1H), 4.23 (dd, J = 12.0, 4.0 Hz, 1H), 4.49 (dd, J = 12.0, 2.5 Hz, 1H), 4.84 (dd, J = 10.0, 4.5 Hz, 1H), 4.86 (d, J = 9.5 Hz, 1H, H-1), 5.00 (t, J = 9.5 Hz, 1H), 5.04 (t, J = 10.0 Hz, 1H), 5.20 (t, J = 9.5 Hz, 1H), 5.32 (t, J = 10.0 Hz, 1H), 5.39 (d, J = 4.5 Hz, 1H); 13C NMR (CDCl3, 125 MHz) δ 20.3, 20.46, 20.49, 20.51, 20.60, 20.69, 20.72, 61.3, 62.1, 67.8, 68.7, 69.1, 69.9, 71.7, 71.9, 74.4, 74.7, 78.9 (C-1), 95.8, 164.3, 169.2, 169.3, 169.8, 170.0, 170.2, 170.39, 170.41. HRMS (ESI): m/z calcd for C27H35NNaO17 [M+ Na]+ 668.1797, found 668.1795.
References
1. a) Y. Ichikawa, T. Nishiyama, and M. Isobe, Synlett, 2000, 1253; CrossRef b) Y. Ichikawa, T. Nishiyama, and M. Isobe, J. Org. Chem., 2001, 66, 4200. CrossRef
2. a) T. Nishiyama, M. Isobe, and Y. Ichikawa, Angew. Chem. Int. Ed., 2005, 44, 4372; CrossRef b) T. Nishiyama, Y. Kusumoto, K. Okumura, K. Hara, S. Kusaba, K. Hirata, Y. Kamiya, M. Isobe, K. Nakano, H. Kotsuki, and Y. Ichikawa, Chem. Eur. J., 2010, 16, 600. CrossRef
3. a) X. Li and S. J. Danishefsky, J. Am. Chem. Soc., 2008, 130, 5446; CrossRef b) X. Wu, Y. Yuan, X. Li, and S. J. Danishefsky, Tetrahedron Lett., 2009, 50, 4666. CrossRef
4. a) T. Ziegler, H.-J. Kaisers, R. Schlömer, and C. Koch, Tetrahedron, 1999, 55, 8397; CrossRef b) C. G. Neochoritis, J. Zhang, and A. Dömling, Synthesis, 2015, 47, 2407. CrossRef
5. R. J. M. Nolte, J. A. J. Van Zomeren, and J. W. Zwikker, J. Org. Chem., 1978, 43, 1972. CrossRef
6. a) Y. Ichikawa, S. Kusaba, T. Minami, Y. Tomita, K. Nakano, and H. Kotsuki, Synlett, 2011, 1462; CrossRef b) Y. Ichikawa, T. Minami, S. Kusaba, N. Saeki, Y. Tonegawa, Y. Tomita, K. Nakano, H. Kotsuki, and T. Masuda, Org. Biomol. Chem., 2014, 12, 3924. CrossRef
7. a) L. M. Likhosherstov, O. S. Novikova, and V. N. Shibaev, Dokl. Chem., 2002, 383, 89; CrossRef b) L. M. Likhosherstov, O. S. Novikova, and V. N. Shibaev, Dokl. Chem., 2003, 389, 73; CrossRef c) L. M. Likhosherstov, O. S. Novikova, A. O. Zheltova, and V. N. Shibaev, Russ. Chem. Bull., Int. Ed., 2004, 53, 709; d) C. P. R. Hackenberger, M. K. O'Reill, and B. Imperiali, J. Org. Chem., 2005, 70, 3574. CrossRef
8. M. H. Benn and A. S. Jones, J. Chem. Soc., 1960, 3837. CrossRef
9. a) R. Babiano, C. Duran, J. Plumet, E. Roman, E. Sanchez, J. A. Serrano, and J. Fuentes, J. Chem. Soc., Perkin Trans. 1, 1989, 1923; b) D. Prosperi, S. Ronchi, L. Lay, A. Rencurosi, and G. Russo, Eur. J. Org. Chem., 2004, 395. CrossRef
10. While the syntheses and its characterization data of the β-glucosyl formamide 3 have been reported in the references 9 and 6b, no characterization data has been reported for the corresponding α-anomer. An authentic sample of α-glucosyl formamide was prepared by hydrolysis of the corresponding α-isocyanide. For details, see SI.
11. Synthesis of glycosides using unprotected carbohydrates dates back to the report by Emil Fischer in 1888. Reaction of D-glucose in MeOH with hydrogen chloride as an acid catalysis produces a mixture of α- and β-methyl glycosides. This Fischer’s synthesis has been extensively employed for the preparation of simple O-glycosides. a) B. Helferich and W. Schäfer, Org. Synth., 1926, 6, 64; CrossRef b) S. Hanessian and B. Lou, Chem. Rev., 2000, 100, 4443. CrossRef
12. An authentic sample of α-xylosyl formamide was prepared by hydrolysis of the corresponding α-isocyanide. For details, see SI.
13. Y. Ichikawa, H. Watanabe, H. Kotsuki, and K. Nakano, Eur. J. Org. Chem., 2010, 6331. CrossRef
14. Characterization data of isocyanides 13 and 14 have been previously reported in the references, 1 and 13. For synthesis and characterization data of 15 and 16, see the experimental section.