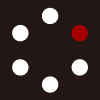
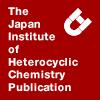
HETEROCYCLES
An International Journal for Reviews and Communications in Heterocyclic ChemistryWeb Edition ISSN: 1881-0942
Published online by The Japan Institute of Heterocyclic Chemistry
e-Journal
Full Text HTML
Received, 29th June, 2010, Accepted, 12th August, 2010, Published online, 13th August, 2010.
DOI: 10.3987/REV-10-676
■ Total Synthesis of Gambierol
Yuji Mori*
Faculty of Pharmacy, Meijo University, 150 Yagotoyama, Tenpaku, Nagoya 468-8503, Japan
Abstract
Gambierol is a marine polycyclic ether toxin isolated from the cultured cells of the ciguatera causative dinoflagellate Gambierdiscus toxicus. The fascinating molecular structure of gambierol, its association with ciguatera poisoning, and its potential biological activity have drawn significant attention from the synthetic community and provided a strong impetus for the development of an efficient path by which a total synthesis can be achieved. We recently accomplished the synthesis of gambierol based on an oxiranyl anion strategy. To date, four total syntheses have been reported. This review focuses on the efforts that culminated in the total synthesis of gambierol.CONTENTS
1. Introduction
2. Sasaki’s Total Synthesis
3. Kadota-Yamamoto’s Total Synthesis
4. Rainier’s Total Synthesis
5. Mori’s Total Synthesis
6. Conclusion
1. INTRODUCTION
Dinoflagellate toxins constitute a large family of compounds, many of which exhibit a wide range of physiological activities. Accordingly, intense activity has been devoted to the isolation and structure determination of toxins. Ciguatera poisoning is a toxicological syndrome resulting from the ingestion of seafood contaminated by certain toxic polycyclic ethers produced by the epiphytic dinoflagellate Gambierdiscus toxicus. The toxin suite comprises ciguatoxins, maitotoxin, and gambierol.1 The large complex architecture of these compounds and their potent neurotoxicity have attracted the attention of chemists and have made them the focus of numerous synthetic efforts.2 Gambierol (1) was isolated as a neurotoxin from the cultured cells of G. toxicus in 1993.3 The absolute stereochemistry was later determined by NMR analysis of the (S)- and (R)-phenylmethoxy(trifluoromethyl)acetate (MTPA) derivatives.4 The toxin exhibits potent toxicity against mice with an LD50 of 50 µg/kg (ip), and its symptoms occurring in mice resemble those displayed in ciguatoxins, indicating that gambierol is also responsible for ciguatera seafood poisoning. The ability of gambierol to inhibit the binding of dihydrobrevetoxin B to voltage-sensitive sodium channels5 has also attracted attention, leading to structure-activity relationship (SAR) studies.6 Further evaluation of its molecular target on the ion channels has revealed that gambierol inhibits the voltage-gated potassium channels in mouse taste cells,7 which might be associated with the taste alteration caused by ciguatera intoxication, and modulates ion fluxes by acting as a partial agonist of sodium channels in human neuroblastoma cells.8 Moreover, gambierol has been reported to act as a functional antagonist of neurotoxin site 5 on voltage-gated sodium channels in cerebellar granule neurons.9
Gambierol (1) consists of a ladder-shaped trans-fused octacyclic ring system that includes 18 stereogenic centers and a partially conjugated triene side chain, including a conjugated (Z,Z)-diene system (Figure 1). The complex architecture and the need for biologically active analogues for SAR study continue to interest organic chemists. To date, three convergent total syntheses by Sasaki’s,10 Kadota-Yamamoto’s,11 and Rainier’s12 groups and one linear iterative synthesis by Mori’s group13 have been achieved. Although, several synthetic efforts directed toward the target have been reported in the literature,14 this review focuses on the works that culminated in the total synthesis of gambierol.
2. SASAKI’S TOTAL SYNTHESIS
2.1. Retrosynthesis. The first total synthesis of gambierol was reported by Sasaki’s group in 2002.10 From the standpoint of developing a convergent strategy, Sasaki’s synthesis provided a demonstration of the great efficacy of B-alkyl Suzuki-Miyaura coupling15 for the convergent synthesis of six-membered ether rings.16 Alkylborane II, prepared from hydroboration of exocyclic enol ether I with 9-BBN, reacted with ketene acetal phosphate III to afford the cross-coupling product IV (Scheme 1). Hydroboration and oxidation provided ketone V, and subsequent acetal formation and reductive etherification enabled the construction of polycyclic ether framework VII in a convergent manner.
Sasaki planned to construct the triene side chain of gambierol (1) by Stille coupling17 of vinyl bromide 2 and tributyl((Z)-penta-1,4-dienyl)stannane (3)18 at a late stage of the synthesis according to the Kadota’s protocol19 (Scheme 2). Retrosynthetic disassembly of the octacyclic intermediate 2 via 4 based on the Suzuki-Miyaura coupling strategy provides the ABC-ring exocyclic enol ether 5 and the EFGH-ring ketene acetal phosphate 6.
2.2. Synthesis of the ABC rings. The synthesis commenced with the known B-ring olefin 7, an intermediate of Nicolaou’s brevetoxin B synthesis, which was prepared from 2-deoxy-D-ribose in 12 steps (Scheme 3).20 The olefin was transformed into allylic alcohol 8 in a conventional three-step operation. In order to introduce the C-6 hydroxy group of the A ring, 8 was subjected to Sharpless epoxidation with the (-)-DET chiral ligand followed by regioselective reductive opening of the resulting epoxy alcohol with Red-Al to afford diol 9. The diol was converted to hydroxy α,β-unsaturated ester 11 in five-step sequences involving: (a) p-methoxybenzylidene acetal formation, (b) regioselective reductive cleavage with DIBALH to form the PMB ether 10, (c) oxidation of the primary alcohol, (d) Wittig reaction, and (e) removal of the TBS group. The A ring was constructed by the intramolecular hetero-Michael addition reaction of hydroxy α,β-unsaturated ester 11 with NaH in THF, resulting in the stereoselective formation of the AB-ring ester 12. Homologation of the ester side chain of 12 and the following sequential manipulations of protecting groups to yield 14 required a six-step operation: DIBALH reduction of the ester, Wittig olefination of the resulting aldehyde to 13, hydroboration/oxidation, benzylation of the primary alcohol, removal of the PMB group, and benzylation. Compound 14 was then converted to olefin 15 in five steps.
Construction of the C ring was performed in a straightforward manner by employing Nicolaou’s 6-endo hydroxy-epoxide cyclization method.21 Hydroboration/oxidation of the double bond of 15 followed by SO3·pyridine oxidation produced an aldehyde, which was subsequently homologated by Horner-Wadsworth-Emmons reaction to give 16. Conversion of 16 to epoxy alcohol 17 was accomplished by DIBALH reduction and m-CPBA epoxidation. Oxidation and Wittig reaction afforded vinyl epoxide 18. The 6-endo cyclization of the desilylated compound of 18 into a tricyclic ring system 19 was carried out by treatment with PPTS in CH2Cl2. Protection of the newly formed secondary alcohol as the PMB ether, oxidative cleavage of the double bond, and the reduction of the derived aldehyde led to a primary alcohol 20, which was transformed into the ABC-ring exocyclic enol ether 5 through iodination of the primary alcohol and elimination.
2.3. Synthesis of the EFGH rings. Sasaki initially attempted the synthesis of EFGH-ring fragment 6 by the B-alkyl Suzuki-Miyaura coupling between an F-ring exocyclic enol ether and an H-ring ketene acetal phosphate. However, the synthesis required 42 steps from compound 21 and its overall yield was only 6%.10d A more efficient and practical route was next explored; this route was based on the SmI2-promoted reductive cyclization protocol developed by Nakata and co-workers,22 which was employed for the construction of the H and F rings. The second approach for the EFGH-ring fragment 6 is illustrated in Scheme 4. Elaboration of compound 2123 to aldehyde 23, a ketyl radical cyclization precursor, via dithiane 22 was carried out by a six-step sequence including the two-carbon diminution of the unsaturated ester side chain followed by the one-carbon extension with 1,3-dithiane, introduction of a β-alkoxy acrylate moiety by hetero-Michael addition with ethyl propiolate, and hydrolysis of dithioacetal. Treatment of 23 with SmI2 induced reductive cyclization to afford stereoselectively the H-ring lactone 24,22 which was then converted to unsaturated ester 25 in excellent yield. The GH-ring system 27 containing an axial methyl group was constructed according to the Nicolaou’s protocol.20 Thus, the unsaturated ester 25 was transformed to vinyl epoxide 26 by a four-step manipulation, and treatment of the latter with TBAF followed by PPTS caused 6-endo cyclization to afford the GH-ring 27 in a stereocontrolled manner. The vinyl group of 28 was converted to a methyl ketone by a four-step operation, and, after removal of the TBS group, a β-alkoxy acrylate side chain was introduced to afford keto acrylate 29. Exposure of 29 to SmI2 again effected stereoselective ketyl radical cyclization14a to furnish the FGH-ring system 30 having angular 1,3-diaxial dimethyl groups. Chain extension of ester 30 to hydroxy carboxylic acid 31 via Wittig reaction followed by Yamaguchi lactonization24 led to a seven-membered lactone, which was finally converted to the EFGH-ring ketene acetal phosphate 6 with KHMDS and (PhO)2P(O)Cl.25 This second approach to 6 had an overall yield of 22% for the 33 steps from 21, which was much more favorable than the first approach.
2.4. Total synthesis. Completion of the synthesis was then achieved along the lines previously developed using the B-alkyl Suzuki-Miyaura coupling strategy.16 Hydroboration of enol ether 5 with 9-BBN and cross coupling with ketene acetal phosphate 6 in the presence of PdCl2(dppf) afforded 4 in 86% yield (Scheme 5).10e A stereoselective hydroboration with a BH3·THF-oxidation sequence gave a secondary alcohol as a single isomer, which was further oxidized with TPAP to ketone 32. It should be mentioned that if the same hydroboration-oxidation sequence was repeated by employing BH3·SMe2, a 7:1 mixture of diastereoisomers was produced. The D-ring was constructed by removal of the PMB group of 32, mixed thioacetal formation with EtSH and Zn(OTf)2, and desulfurization under radical reductions (Ph3SnH, AIBN) to afford the octacyclic polyether 33 after acetylation. This diacetate 33 was transformed into ketone 34 in three steps. Elaboration of the H-ring of 34 continued with incorporation of the H-ring double bond by Ito-Saegusa oxidation26 of the derived silyl enol ether with Pd(OAc)2 in MeCN to form an α,β-unsaturated ketone and subsequent stereoselective installation of an axial methyl group with MeMgBr in toluene at -78 °C to afford tertiary alcohol 35 in 94% overall yield as the sole product.27 In order to construct the triene side chain of gambierol, two benzyl groups of 35 were reductively removed with LiDBB at this stage and the resulting diol was reprotected with TBDPS and TBS groups to afford alcohol 36 after selective removal of the TBS group of the primary alcohol in 92% overall yield for the five steps. Oxidation of the alcohol followed by Corey-Fuchs reaction28 of the derived aldehyde gave a dibromoolefin, which was stereoselectively mono-debrominated with n-Bu3SnH and Pd(PPh3)429 to afford (Z)-vinyl bromide 37 in 82% yield for the three steps.
Finally, the vinyl bromide side chain was converted into the requisite partially conjugated triene. The Stille coupling reaction of 37 with (Z)-vinyl stannane 3 employing Pd(PPh3)4/CuCl/LiCl30 gave fully protected gambierol in 66% yield, which was then subjected to global deprotection. However, the sterically hindered C-30 TBS ether was not removed under various conditions, such as TBAF, HF·pyridine, and Et3N·3HF. Completion of the synthesis was finally achieved by exchanging the order of the last two steps. Thus, removal of three silyl groups with excess HF·pyridine afforded triol 2. The Stille coupling of the unprotected 2 with (Z)-vinylstannane 318 provided gambierol (1) in 43% yield.
3. KADOTA-YAMAMOTO’S TOTAL SYNTHESIS
3.1. Retrosynthesis. Kadota and Yamamoto have exploited a novel approach to polycyclic ethers using intramolecular cyclization of γ-alkoxy allylstannanes to α-acetoxy ethers (Scheme 6).31 Ester X prepared from acid VIII and alcohol VIV was converted to allylstannane XI. Partial reduction of the ester was followed by in-situ trapping of the resulting hemiacetal with acetic anhydride to afford α-acetoxy ether XII. Intramolecular allylation of XII with MgBr2·OEt2 induced the formation of a 7-membered ring to give XIII, which was then transformed to the tetracyclic ether-ring system XIV by a ring-closing metathesis (RCM) reaction. The rapid construction of two ether rings by means of this synthesis is noteworthy, and the technology was applied to the synthesis of gambierol.11
A brief retrosynthesis of gambierol is illustrated in Scheme 7. According to the preliminary study for the synthesis of the H ring19 and taking into account Sasaki’s synthesis,10b the triene side chain would be constructed by the Stille coupling employing vinyl stannane 3 and vinyl iodide 38, which is more reactive than vinyl bromide 2. According to the strategy shown in Scheme 6, the D ring of 38 can be constructed by intramolecular allylation of an α-acyloxy ether, and the subsequent ring-closing metathesis would allow formation of the E ring. The key α-acyloxy ether 39 would be retrosynthetically broken down into the ABC-ring acid 40 and the FGH-ring alcohol 41.
3.2. Synthesis of the ABC rings. Synthesis of the ABC rings began with Brown’s asymmetric allylation32 of the aldehyde prepared from the known olefin 720 to afford homoallylic alcohol 42 as the sole product (Scheme 8). Chain extension to α,β-unsaturated ester 43 was achieved by ozonolysis followed by Wittig condensation. Exposure of 43 to K2CO3 in THF-MeOH resulted in the formation of the desired AB-ring system 44 by intramolecular hetero-Michael reaction, which still required an additional carbon atom to form the A-ring side chain.
This homologation was then accomplished via Wittig olefination and hydroboration/oxidation to afford alcohol 45 in four steps. Five-step protecting group manipulation followed by oxidation of 46 to aldehyde and subsequent methylenation led to the Sasaki’s AB-ring fragment 15.10e This intermediate was then converted into aldehyde 48 via hydroxy dithioacetal 47 in six steps. Construction of the C-ring was performed by the SmI2-induced ketyl radical cyclization of 48 to give hydroxy ester 49 in an excellent yield. Protection of the secondary alcohol, reduction of the ester, and oxidation of the resulting primary hydroxy group of 50 produced the ABC-ring acid 40 in quantitative yield.
3.3. Synthesis of the FGH rings. Construction of the second fragment corresponding to the FGH rings began with the transformation of the known ester 52,14a prepared from 2-dexoy-D-ribose via 5120 in fifteen steps, to tosylate 53 in 84% yield for the four steps (Scheme 9). This intermediate was then coupled with allylmagnesium bromide in the presence of CuBr to afford olefin 54. Ozonolysis/reduction and protection of the primary alcohol as a pivalate was followed by acid-catalyzed mixed-acetal formation with tributyl((Z)-3-methoxyallyl)stannane and subsequent elimination of MeOH to furnish (Z)-allylic stannane 55.33 Reductive removal of the pivaloyl group with DIBALH followed by oxidation with SO3·pyridine gave aldehyde 56. Cyclization of 56 mediated by BF3·OEt2 afforded the tricyclic FGH-ring 57 in 99% yield as a single diastereoisomer.34 Ozonolysis of the double bond followed by reduction, benzylidene acetal formation, and selective desilylation of the primary TBS ether provided alcohol 58. Synthesis of the required FGH-ring olefinic alcohol 41 from 58 was completed by selenylation, oxidative elimination, and desilylation.
3.4. Total synthesis. Union of the two segments 40 and 41 was now effected by Yamaguchi conditions,24 and subsequent removal of the TBS group afforded ester 59 in 93% yield for the two steps (Scheme 10). Acetal formation with (Z)-methoxyallylstannane and acetal cleavage furnished allylic stannane 60.
The ester 60 was then converted to a 3:2 mixture of α-chloroacetoxy ether 39 by partial reduction with DIBALH followed by in-situ acylation with chloroacetic anhydride according to the Rychnovsky protocol.35 Construction of the D ring was initially attempted using 39 (R = COCH3) with MgBr2·OEt2 to give a 36:64 ratio of 61 and its C-16 epimer. The ratio was finally improved to 64:36 by treatment of α-chloroacetyl derivative 39 (R = COCH2Cl) with BF3·OEt2, and the desired product 61 was isolated in 56% yield. This diene was then subjected to RCM reaction using the second-generation Grubbs catalyst36 to provide octacyclic ether 62 in 88% yield. A series of hydrolysis, selective protection, and oxidation steps provided ketone 63. Hydrogenation followed by a protecting group exchange afforded ketone 64. Saegusa oxidation26 of the derived silyl enol ether afforded enone 65, which was stereoselectively methylated with MeMgBr in toluene27 to give the primary alcohol 66 after a protection-deprotection sequence. Finally, completion of the synthesis was accomplished by oxidation of 66 with PCC, diiodoolefination with PPh3/CI4, and stereoselective hydrogenolysis with Zn-Cu/AcOH to provide (Z)-vinyl iodide 67. Removal of the pivaloyl group with DIBALH and the two silyl groups with SiF4 yielded trihydroxy (Z)-vinyl iodide 38, which was subjected to modified Stille coupling with 3 using Pd2(dba)3·CHCl3/P(furyl)3/CuI conditions19,37 to afford synthetic gambierol (1) in 72% yield.
4. RAINIER’S TOTAL SYNTHESIS
4.1. Retrosynthesis. The third synthesis in this area is that of Rainier in 2005.12 An iterative strategy developed to synthesize polycyclic ethers was the generation of C-glycoside XVII from cyclic enol ether XV via nucleophilic addition of organometalic reagents to epoxide XVI (Scheme 11). Transformation of the acylated C-glycoside XVIII into cyclic enol ether XX was performed through the use of an enol ether-olefin RCM reaction of XIX.38
Rainier’s plan to synthesize gambierol was a convergent one that centered on the combined use of the above C-glycosylation and enol ether-olefin RCM reactions (Scheme 12). The octacyclic (Z)-vinyl iodide 38 would be dissected to cyclic enol ether 68. Disassembly of 68 based on the enol ether-olefin RCM strategy would provide the ABC-ring acid 69 and the FGH-ring 70, which could be prepared from 72 and 74, respectively, by iteration of the C-glycosylation strategy. Final steps to incorporate the skipped triene side chain using Kadota’ protocol11 were planned.
4.2. Synthesis of the ABC rings. Preparation of the ABC-ring fragment 69 was initiated by asymmetric hetero Diels-Alder cycloaddition between aldehyde 75 and Danishefsky’s diene 7612d (Scheme 13). Jacobsen’s tridentate Cr(III) catalyst 7739 catalyzed the reaction to give the A ring unit 72 in both high yield and enantiomeric purity. Reduction of the ketone using Luche conditions40 followed by protection with a PMB group afforded 78. Although the C-6 stereocenter was epimeric to that needed for gambierol, this center was employed to control the facial selectivity in the subsequent epoxidation with DMDO. Reaction of 78 with DMDO followed by addition of allylmagnesium chloride afforded β-glycoside in 78% yield with 7.5:1 diastereoselectivity, which was acetylated to give acetate 79. When the acetate was subjected to the Takai-Utimoto reagent,41 a 1:1 mixture of cyclic and acyclic enol ethers, 71 and 80, was isolated. Further treatment of the mixture with the Grubbs II catalyst 81 yielded cyclic enol ether 71 in 74% yield. Iteration of the above C-glycoside strategy (78 → 79) could be used to construct the ester 86 from 71. However, this strategy was unsuccessful because the addition of allylmagnesium chloride to the epoxide derived from 71 resulted in the formation of the undesired C-11 diastereoisomer. This problem was solved by the use of a Claisen rearrangement of an allyl enol ether. Thus, treatment of 71 with m-CPBA in MeOH followed by O-allylation provided 82. Claisen rearrangement of 82 in pyridine in the presence of PPTS at 100 °C afforded 84 in 86% yield. Inversion of the C-6 stereogenic center of 84 was accomplished in four steps involving a Mitsunobu reaction to give 85, which was subjected to reduction with NaBH4 and esterification with 3-(triisopropylsilyloxy)propanoic acid to provide olefinic ester 86. Acyclic enol ether formation using the Takai-Utimoto protocol followed by enol ether-olefin RCM afforded the tricyclic enol ether 87 in 70% yield. Hydroboration followed by exchange of the benzyl ether for a TBDPS ether and hydrolysis of the primary TIPS ether yielded the diol 88. Finally, bis-TES ether formation, selective hydrolysis of the primary TES ether, and oxidation afforded the ABC-ring acid 69 in 89% overall yield.
4.3. Synthesis of the FGH rings. Synthesis of the FGH-ring fragment 70 began with D-glucal (Scheme 14).12c Oxidation of 74 with DMDO under Messengeur’s acetone free conditions42 followed by addition of 2-methyl-2-propenymagnesium chloride gave 90 in 92% yield. Removal of the TBDPS group with NaH in HMPA, selective silylation of the C-25 hydroxy group, and esterification with acid 91 afforded hydroxy ester 92 after hydrolysis of the TMS ether. The Barton-McCombie deoxygenation of the remaining hydroxy group with Bu3SnH led to olefinic ester 93, which was converted into the FG-ring enol ether 94 in 52% yield by Takai-Utimoto reaction followed by RCM reaction using the Grubbs II catalyst 81. Construction of the F-ring still required the additional C-20 and C-21 stereocenters. To this end, 94 was subjected to DMDO oxidation and subsequent DIBALH reduction to afford the desired isomer 73 in a >30:1 diastereomeric ratio. The cyclic silylene group was removed and the resulting triol was transformed into the primary triflate and secondary TBS ether derivative. Coupling reaction of the triflate with allylmagnesium chloride in the presence of CuI43 provided diol 96 after desilylation.
Esterification of the secondary alcohol with (t-butyldimethylsilyloxy)acetic acid followed by trimethylsilylation of the tertiary alcohol gave olefinic ester 97. Sequential exposure of 97 to the Takai-Utimoto conditions and Schrock’s molybdenum catalyst 9844 afforded a 62% yield of the FGH tricyclic enol ether 99 over the two steps. The Grubbs II catalyst was less effective than the Schrock catalyst, resulting in the generation of 99 in 35-39% overall yield from 97. The DMDO oxidation and DIBALH reduction gave alcohol 100 as a single diastereoisomer. The remaining transformation required the introduction of a double bond and a tertiary methyl group to the H-ring. Accordingly, 100 was converted to enone 101 by TPAP and Saegusa oxidations. Addition of MeMgBr in toluene followed by silylation afforded 102. A series of deprotection, oxidation, olefination, deprotection, and reprotection steps provided the FGH-ring fragment 70.
4.4. Total synthesis. Coupling of the two fragments 69 and 70 was then effected by the Yamaguchi method24 to provide ester 103 (Scheme 15). Subsequent RCM reaction with Takai-Utimoto ethylidene reagent generated from dibromoethane afforded the cyclic enol ether 68 in 60% yield. The attempted RCM reactions employing titanium methylidene reagent prepared from dibromomethane were not successful in this case, producing 68 in a capricious 10-30% yield. Single-flask DMDO oxidation of the cyclic enol ether 68 and reduction of the resulting epoxide with DIBALH led to a secondary alcohol, which was oxidized with TPAP to provide ketone 104. Construction of the D ring was achieved by desilylation, cyclic O,S-acetalization, and reductive desulfurization under radical conditions to afford the octacyclic alcohol 105 in 77% yield. Completion of the synthesis was then accomplished along the lines previously developed by the Kadota-Yamamoto group.11 Thus, oxidation of 105 to the aldehyde was followed by Corey-Fuchs reaction to form diiodoolefin 106. Stereoselective reduction of 106 to (Z)-vinyl iodide and global deprotection afforded triol 38. Stille coupling of the resulting triol 38 with dienyl stannane 3 provided synthetic gambierol (1) in 85% yield.
5. MORI’S TOTAL SYNTHESIS
5.1. Retrosynthesis. Our laboratory has been interested in the synthesis of bioactive polycyclic ether natural products through the use of oxiranyl anions in an iterative manner. We have developed a unique method for the formation of tetrahydropyran XXV by the reaction of triflate XXII with oxiranyl anion XXIII followed by a sulfonyl-assisted 6-endo cyclization of the product XXIV (Scheme 16).45 Using this approach, we achieved direct formation of oxepane XXVI by employing a ring-expansion reaction of XXV.46 Such structural units found in gambierol make it an attractive target for possible applications of our methodology, and we have completed the fourth synthesis of gambierol based on the iterative use of oxiranyl anion chemistry.13
Our linear synthetic strategy is outlined in Scheme 17. The reported total syntheses have successfully employed the Stille coupling reaction of (Z)-vinyl bromide 2 or the corresponding vinyl iodide 38 with a (Z)-dienyl stannane 3 at the final step. This conversion to gambierol is efficient and reliable from the perspective of constructing the stereochemically labile conjugated (Z,Z)-diene system. As a result, our synthetic plan has also focused on the construction of 38. We envisioned that two seven-membered E and H rings in 38 would be constructed by a ring-expansion reaction of tetrahydropyranyl rings at suitable stages of synthesis, so we tentatively regarded 107 as a hypothetical polyepoxide precursor for retrosynthetic disassembly. Disconnection of 107 at the indicated bonds with the aid of sulfonyl groups allowed for generation of the ABCD-ring fragment 108, the optically active epoxy sulfones 109, and 110 as building blocks. The advanced CD-ring fragment 111 was then retrosynthetically broken by considering the intermediate 112 to afford epoxy sulfone 113 and triflate 114 as potential starting materials. In order to facilitate the gram-scale preparation of the starting epoxy sulfone, we decided to employ racemic 113, which could readily be prepared as a mixture of cis- and trans-isomers according to the reported method.47
5.2. Synthesis of the ABCD rings. According to the retrosynthesis, rings D, C, B, and A were annulated sequentially in this order, starting with an oxiranyl anion coupling between epoxy sulfone 113 and triflate 114 (Scheme 18). The triflate 114 was prepared from D-glucal in four steps.48 The product 115 obtained in 88% yield was treated with MgBr2·OEt2 to give a 1:1 mixture of α-bromoketones, which was then subjected to cycloetherification with DBU to afford the D-ring ketone 116 in 88% yield for the two steps.49 Reduction of the ketone, protection of the resulting alcohol as the acetate, and subsequent Wacker oxidation of the double bond gave ketone 117. Deacetylation followed by hetero-Michael addition with ethyl propiolate provided keto acrylate 118 in 90% overall yield. Treatment of 118 with SmI2 effected ketyl radical cyclization22 to afford, after silylation, the CD ring ester 119 as a single diastereoisomer. Elaboration of the ester to allylic alcohol 120 was carried out by a three-step sequence involving reduction of the ester with DIBALH, Wittig olefination, and the DIBALH reduction of the unsaturated ester. Construction of the B ring containing sterically congested 1,3-diaxial dimethyl groups was achieved according to the Nicolaou’s protocol.20 Thus, stereoselective epoxidation of the allylic alcohol 120 followed by oxidation and Wittig olefination provided epoxy olefin 121. Detrimethylsilylation followed by treatment with PPTS effected 6-endo cyclization to afford the BCD-ring fragment 122 in good yield.
Construction of the A ring is a challenging issue, because the α-axial hydroxy group at C-6 and the β-equatorial side chain at C-4 have to be installed stereoselectively. To this end, vinyl alcohol 122 was subjected to the homoallylic hydroxy-directed epoxidation with t-BuO2H in the presence of VO(acac)2 to afford the desired epoxide with 93:7 diastereoselectivity.50 Protection of the hydroxyl group as a TES ether provided epoxide 123 in 82% yield for the two steps. Addition of lithiodithiane generated from 124 gave dithioacetal 125 quantitatively. It should be mentioned that when the benzyl-protected derivative of 124 was employed only 28% yield of the product was realized because of decomposition of the benzyl-protected dithiane via anion formation at the benzylic position. The dithioacetal 125 was then transformed to the dihydroxy ketone 126 in three steps including hydrolysis of the dithioacetal group. Reductive etherification of 126 was accomplished with triethylsilane in the presence of SnCl4 to afford the ABCD-ring system 127 in 91% yield as the sole product. A three-step protecting group manipulation provided diol 128,13a which underwent a subsequent one-pot triflation and triethylsilylation to afford the ABCD-ring triflate 108.
5.3. Synthesis of the ABCDEFGH rings. Construction of the seven-membered E-ring began with the coupling reaction of triflate 108 and the oxiranyl anion derived from epoxy sulfone 109 with n-BuLi at -100 °C to give, after desilylation, hydroxy epoxy sulfone 129 in 83% yield (Scheme 19). Treatment with BF3·OEt2 effected 6-endo cyclization to give 130 quantitatively,45b which was followed by ring expansion with trimethylsilyldiazomethane in the presence of BF3·OEt2 and desilylation of the resulting α-trimethylsilyl ketone with TBAF to afford the ABCDE-ring ketone 131.46 Reduction of the ketone and dehydrobromination of vinyl bromide with TBAF in DMF gave acetylene 132.51 Mercuric triflate-catalyzed hydration52 of the terminal acetylene followed by reaction with methyl propiolate provided keto acrylate 133. Treatment of 133 with SmI2-induced reductive cyclization to afford the ABCDEF-ring fragment 134 as a single diastereoisomer after silylation of the secondary hydroxy group. Elaboration of 134 to 136 required the one-carbon diminution of the ester side chain. To this end, the ester was converted to olefin 135 by reduction and selenylation-oxidative elimination. Oxidative cleavage of the double bond followed by reduction and desilylation provided diol 136, from which point the oxiranyl anion chemistry could be repeated for the construction of the G and H rings.
Triflation and trimethylsilylation of 136 in one pot gave triflate, which was then treated with the oxiranyl anion generated from 110 to afford epoxy sulfone 137 in 93% yield. Exposure of 137 to BF3·OEt2 caused desilylation and 6-endo cyclization to form the G-ring ketone 138 in 91% yield. Stereoselective reduction followed by removal of the TBDPS group and one-pot triflation and silylation furnished triflate 139 in 91% yield for the three steps. The sequence described above was employed iteratively with equal efficiency to construct the H-ring. Thus, lithiation of 110 in the presence of 139 with n-BuLi at -100 °C afforded, after desilylation, 140 in 92% yield. Cyclization of 140 was then induced by exposure of BF3·OEt2, which gave rise to octacyclic ketone 141 in 83% yield.
5.4. Total synthesis. With construction of the quasi-octacyclic core 141 of gambierol completed, conversion to the target required the elaboration of the six-membered H ring to the fully functionalized seven-membered H ring having a skipped triene side chain. To this end, ketone 141 was subjected to ring expansion with trimethylsilyldiazomethane and desilylation of the derived α-trimethylsilyl ketone with PPTS to afford the requisite octacyclic core 142 in 81% yield (Scheme 20). The remaining functionalization of the H ring was then achieved along the lines previously developed for gambierol.10,11 Thus, Saegusa oxidation of the silyl enol ether derived from 142 afforded enone 143. Methylation with MeMgBr in toluene gave a tertiary alcohol as a single diastereoisomer, which was followed by removal of the TBDPS group, bis-triethylsilylation, and selective mono-desilylation to provide the primary alcohol 144 in good yield.
In the work previously performed by Sasaki’s and Kadota’s groups, the robust C-1 and C-6 benzyl protecting groups were replaced by more easily removable silyl groups or silyl and pivaloyl groups prior to installation of a (Z)-vinyl bromide or vinyl iodide, respectively. However, if debenzylation were feasible in the presence of the vinyl iodide functionality, a straightforward route to reaching triol 38 would be made possible. Accordingly, alcohol 144 was oxidized with TPAP to furnish aldehyde 145, which was subjected to iodomethylenation with iodomethyltriphenylphosphonium iodide and NaHMDS53 to afford (Z)-vinyl iodide 146 in 63% yield for the two steps. Debenzylation was now critical for the successful completion of our route, as it needed to be executed in the presence of the labile (Z)-vinyl iodide, cyclic allylic ether, and TES ether functionalities. Upon considerable experimentation, it was finally accomplished by heating 146 with DDQ in the presence of water and diallyl ether in dichloroethane at 50 °C for 3 h,54 leading to, after removal of the TES ether, the desired triol 38 in 78% overall yield. Stille coupling of 38 with dienyl stannane 3 using Kadota’s conditions afforded a 68% yield of gambierol.
CONCLUSION
Gambierol stood for more than ten years as a challenge to synthetic chemists. The necessity for the development of new synthetic methods for the construction of gambierol’s skeletons stimulated the invention of convergent and iterative protocols for the regio- and stereoselective polytetrahydropyran and oxepane ring system. These synthetic methods demonstrated the great efficiency in the total synthesis of gambierol and have proved to be applicable to complex polycyclic ether natural products. However, the syntheses still required more than 70 steps in total: Sasaki’s synthesis, 107 steps (the longest linear sequence from 2-deoxy-D-ribose is 71 steps with 0.57% overall yield); Kadota’s synthesis, 102 steps (the longest linear sequence from 2-deoxy-D-ribose is 66 steps with 1.2% overall yield); Rainier’ synthesis, 69 steps (the longest linear sequence from D-glucal is 44 steps with 1.5% overall yield); Mori’s synthesis, 74 steps (the longest linear sequence from tri-O-acetyl D-glucal is 74 steps with 0.1% overall yield). Therefore, the development of more efficient and concise synthetic routes is a remaining challenge.
ACKNOWLEDGEMENTS
This research was supported by Grants-in-Aid for Scientific Research on Priority Areas (18032079) and for Scientific Research (C) (21590029) from The Ministry of Education, Culture, Sports, Science, and Technology. We thank Professors M. Sasaki (Tohoku University) and I. Kadota (Okayama University) for providing the 1H and 13C NMR spectra of gambierol.
References
1. For reviews on marine polycyclic ethers, see: (a) T. Yasumoto and M. Murata, Chem. Rev., 1993, 93, 1897; CrossRef (b) M. Murata and T. Yasumoto, Nat. Prod. Rep., 2000, 17, 293; CrossRef (c) T. Yasumoto, Chem. Rec., 2001, 1, 228. CrossRef
2. For recent reviews of the synthesis of marine polycyclic ethers, see: (a) M. Inoue, Chem. Rev., 2005, 105, 4379; CrossRef (b) T. Nakata, Chem. Rev., 2005, 105, 4314; CrossRef (c) M. Sasaki and H. Fuwa, Nat. Prod. Rep., 2008, 25, 401. CrossRef
3. M. Satake, M. Murata, and T. Yasumoto, J. Am. Chem. Soc., 1993, 115, 361. CrossRef
4. A. Morohashi, M. Satake, and T. Yasumoto, Tetrahedron Lett., 1998, 54, 12630.
5. M. Inoue, M. Hirama, M. Satake, K. Sugiyama, and T. Yasumoto, Toxicon, 2003, 41, 469. CrossRef
6. H. Fuwa, N. Kainuma, K. Tachibana, C. Tsukano, M. Satake, and M. Sasaki, Chem. Eur. J., 2004, 10, 4894. CrossRef
7. (a) V. Ghiaroni, M. Sasaki, H. Fuwa, G. P. Rossini, G. Scalera, T. Yasumoto, P. Pietra, and A. Bigiani, Toxicol. Sci., 2005, 85, 657; CrossRef (b) V. Ghiaroni, H. Fuwa, M. Inoue, M. Sasaki, K. Miyazaki, M. Hirama, T. Yasumoto, G. P. Rossini, G. Scalera, and A. Bigiani, Chemical Senses, 2006, 31, 673. CrossRef
8. M. Louzao, E. Cagide, M. R. Vieytes, M. Sasaki, H. Fuwa, T. Yasumoto, and L. M. Botana, Cell. Physiol. Biochem., 2006, 17, 257. CrossRef
9. (a) E. Cuypers, A. Yanagihara, J. D. Rainier, and J. Tytgat, Biochem. Biophys. Res. Commun., 2007, 361, 214; CrossRef (b) K. T. LePage, J. D. Rainier, H. W. B. Johnson, D. G. Baden, and T. F. Murray, J. Pharmacol. Exp. Ther., 2007, 323, 174; CrossRef (c) E. Cuypers, Y. Abdel-Mottaleb, I. Kopljar, J. D. Rainier, A. L. Raes, D. J. Snyders, and J. Tytgat, Toxicon, 2008, 51, 974; CrossRef (d) F. Pietra, J. Phys. Org. Chem., 2008, 21, 997; CrossRef (e) I. Kopljar, A. J. Labro, E. Cuypers, H. W. B. Johnson, J. D. Rainier, J. Tytgat, and D. J. Snyders, Proc. Natl. Acad. Sci. U.S.A., 2009, 106, 9896. CrossRef
10. (a) H. Fuwa, M. Sasaki, M. Satake, and K. Tachibana, Org. Lett., 2002, 4, 2981; CrossRef (b) H. Fuwa, N. Kainuma, K. Tachibana, and M. Sasaki, J. Am. Chem. Soc., 2002, 124, 14983; CrossRef (c) H. Fuwa, N. Kainuma, K. Tachibana, C. Tsukano, M. Satake, and M. Sasaki, Chem. Eur. J., 2004, 10, 4894; CrossRef (d) H. Fuwa, M. Sasaki, and K. Tachibaha, Tetrahedron, 2001, 57, 3019; CrossRef (e) H. Fuwa, M. Sasaki, and K. Tachibaha, Org. Lett., 2001, 3, 3549. CrossRef
11. (a) I. Kadota, H. Takamura, K. Sato, A. Ohno, K. Matsuda, and Y. Yamamoto, J. Am. Chem. Soc., 2003, 125, 46; CrossRef (b) I. Kadota, H. Takamura, K. Sato, A. Ohno, K. Matsuda, M. Satake, and Y. Yamamoto, J. Am. Chem. Soc., 2003, 125, 11893. CrossRef
12. (a) H. W. B. Johnson, U. Majumder, and J. D. Rainier, J. Am. Chem. Soc., 2005, 127, 848; CrossRef (b) U. Majumder, J. M. Cox, H. W. B. Johnson, and J. D. Rainier, Chem. Eur. J., 2006, 12, 1736; CrossRef (c) H. W. B. Johnson, U. Majumder, and J. D. Rainier, Chem. Eur. J., 2006, 12, 1747; CrossRef (d) J. M. Cox and J. D. Rainier, Org. Lett., 2001, 3, 2919; CrossRef (e) U. Majumder, J. M. Cox, and J. D. Rainier, Org. Lett., 2003, 5, 913. CrossRef
13. (a) H. Furuta, M. Hase, R. Noyori, and Y. Mori, Org. Lett., 2005, 7, 4061; CrossRef (b) H. Furuta, Y. Hasegawa, and Y. Mori, Org. Lett., 2009, 11, 4382; CrossRef (c) H. Furuta, Y. Hasegawa, M. Hase, and Y. Mori, Chem. Eur. J., 2010, 16, 7586. CrossRef
14. (a) Y. Sakamoto, G. Matsuo, H. Matsukura, and T. Nakata, Org. Lett., 2001, 3, 2749; CrossRef (b) T. Saito, T. Takeuchi, M. Matsuhashi, and T. Nakata, Heterocycles, 2007, 72, 151; CrossRef (c) T. Saito and T. Nakata, Org. Lett., 2009, 11, 113. CrossRef
15. (a) A. Suzuki and N. Miyaura, Chem. Rev., 1995, 95, 2457; CrossRef (b) S. R. Chemler, D. Trauner, and S. J. Danishefsky, Angew. Chem. Int. Ed., 2001, 40, 4544. CrossRef
16. M. Sasaki and H. Fuwa, Synlett, 2004, 1851. CrossRef
17. (a) J. K. Stille, Angew. Chem., Int. Ed. Engl., 1986, 25, 508; CrossRef (b) V. Farina, V. Krishnamurthy, and W. J. Scott, ‘Organic Reactions’ Vol. 50, ed. by L. A. Paquette, John Wiley and Sons, New York, 1997, pp. 1-652.
18. (a) E. Shirakawa, K. Yamasaki, H. Yoshida, and T. Hiyama, J. Am. Chem. Soc., 1999, 121, 10221; CrossRef (b) Y. Matsukawa, N. Asao, N. Kitahara, and Y. Yamamoto, Tetrahedron, 1999, 55, 3779. CrossRef
19. I. Kadota, A. Ohno, Y. Matsukawa, and Y. Yamamoto, Tetrahedron Lett., 1998, 39, 6373. CrossRef
20. K. C. Nicolaou, D. A. Nugiel, E. Couladouros, and C.-K. Hwang, Tetrahedron, 1990, 46, 4517. CrossRef
21. K. C. Nicolaou, C. V. C. Prasad, P. K. Somers, and C.-K. Hwang, J. Am. Chem. Soc., 1989, 111, 5330. CrossRef
22. (a) N. Hori, H. Matsukura, G. Matsuo, and T. Nakata, Tetrahedron Lett., 1999, 40, 2811; CrossRef (b) N. Hori, H. Matsukura, and T. Nakata, Org. Lett., 1999, 1, 1099. CrossRef
23. K. C. Nicolaou, P. A. Wallace, S. Shi, M. A. Ouellette, M. E. Bunnage, J. L. Gunzner, K. A. Agrios, G.-Q. Shi, P. Gärtner, and Z. Yang, Chem. Eur. J., 1999, 5, 618. CrossRef
24. M. Yamaguchi, J. Inanaga, K. Hirata, and T. Katsuki, Bull. Chem. Soc. Jpn., 1979, 52, 1989. CrossRef
25. K. C. Nicolaou, G.-Q. Shi, J. L. Gunzner, P. Gärtner, and Z. Yang, J. Am. Chem. Soc., 1997, 119, 5467. CrossRef
26. Y. Ito, T. Hirao, and T. Saegusa, J. Org. Chem., 1978, 43, 1011. CrossRef
27. F. Feng and A. Murai, Chem. Lett., 1992, 1587. CrossRef
28. E. J. Corey and P. L. Fuchs, Tetrahedron Lett., 1972, 13, 3769. CrossRef
29. (a) J. Uenishi, R. Kawahama, and O. Yonemitsu, J. Org. Chem., 1996, 61, 5716; CrossRef (b) J. Uenishi, R. Kawahama, Y. Shiga, and O. Yonemitsu, Tetrahedron Lett., 1996, 37, 6759; CrossRef (c) J. Uenishi, R. Kawahama, O. Yonemitsu, and J. Tsuji, J. Org. Chem., 1998, 63, 8965. CrossRef
30. X. Han, B. M. Stolz, and E. J. Corey, J. Am. Chem. Soc., 1999, 121, 7600. CrossRef
31. (a) I. Kadota, A. Ohno, K. Matsuda, and Y. Yamamoto, J. Am. Chem. Soc., 2001, 123, 6702; CrossRef (b) I. Kadota, A. Ohno, K. Matsuda, and Y. Yamamoto, J. Am. Chem. Soc., 2002, 124, 3562. CrossRef
32. U. S. Racherla and H. C. Brown, J. Org. Chem., 1991, 56, 401. CrossRef
33. I. Kadota, T. Sakaihara, and Y. Yamamoto, Tetrahedron Lett., 1996, 37, 3195. CrossRef
34. (a) Y. Yamamoto, J. Yamada, and I. Kadota, Tetrahedron Lett., 1991, 32, 7069; CrossRef (b) I. Kadota, M. Kawada, V. Gevorgyan, and Y. Yamamoto, J. Org. Chem., 1997, 62, 7439. CrossRef
35. (a) V. H. Dahanukar and S. D. Rychnovsky, J. Org. Chem., 1996, 61, 8317; CrossRef (b) D. J. Kopecky and S. D. Rychnovsky, J. Org. Chem., 2000, 65, 191. CrossRef
36. M. Scholl, S. Ding, C. W. Lee, and R. H. Grubbs, Org. Lett., 1999, 1, 953. CrossRef
37. (a) L. S. Liebeskind and R. W. Fengl, J. Org. Chem., 1990, 55, 5359; CrossRef (b) V. Ferina, S. Kapadia, B. Krishnan, C. Wang, and L. S. Liebeskind, J. Org. Chem., 1994, 59, 5905. CrossRef
38. S. P. Allwein, J. M. Cox, B. H. Howard, H. W. B. Johnson, and J. D. Rainier, Tetrahedron, 2002, 58, 1997. CrossRef
39. A. G. Dossetter, T. F. Jamison, and E. N. Jacobsen, Angew. Chem. Int. Ed., 1999, 38, 2398. CrossRef
40. A. L. Gemal and J.-L. Luche, J. Org. Chem., 1979, 44, 4187. CrossRef
41. (a) K. Takai, T. Kakiuchi, Y. Kataoka, and K. Utimoto, J. Org. Chem., 1994, 59, 2668; CrossRef (b) J. Hibino, T. Okazoe, K. Takai, and H. Nozaki, Tetrahedron Lett., 1985, 26, 5579. CrossRef
42. M. Ferrer, M. Gilbert, S.-F. Baeza, and A. Messeguer, Tetrahedron Lett., 1996, 37, 3585. CrossRef
43. H. Kotsuki, I. Kadota, and M. Ochi, J. Org. Chem., 1990, 55, 4417. CrossRef
44. (a) R. R. Schrock, J. S. Murdzek, G. C. Bazan, J. Robbins, M. DiMare, and M. B. O’Regan, J. Am. Chem. Soc., 1990, 112, 3875; CrossRef (b) O. Fujimura, G. C. Fu, and R. H. Grubbs, J. Org. Chem., 1994, 59, 4029. CrossRef
45. (a) Y. Mori, K. Yaegashi, and H. Furukawa, J. Am. Chem. Soc., 1996, 118, 8158; CrossRef (b) H. Furuta, T. Takase, H. Hayashi, R. Noyori, and Y. Mori, Tetrahedron, 2003, 59, 9767. CrossRef
46. (a) Y. Mori, K. Yaegashi, and H. Furukawa, Tetrahedron, 1997, 53, 12917; CrossRef (b) Y. Mori, K. Nogami, H. Hayashi, and R. Noyori, J. Org. Chem., 2003, 68, 9050. CrossRef
47. T. Satoh, T. Oohara, Y. Ueda, and K. Yamakawa, J. Org. Chem., 1989, 54, 3130. CrossRef
48. Y. Mori and H. Hayashi, J. Org. Chem., 2001, 66, 8666. CrossRef
49. Y. Mori, K. Yaegashi, and H. Furukawa, Tetrahedron Lett., 1999, 40, 7239. CrossRef
50. A. S. Rao ‘Comprehensive Organic Synthesis’ Vol. 7, ed. by B. M. Trost, Pergamon Press, Inc., Oxford, 1991, pp. 357-387.
51. (a) M. Okutani and Y. Mori, Tetrahedron Lett., 2007, 48, 6856; CrossRef b) M. Okutani and Y. Mori, J. Org. Chem., 2009, 74, 442. CrossRef
52. M. Nishizawa, M. Skwarczynski, H. Imagawa, and T. Sugihara, Chem. Lett., 2002, 12. CrossRef
53. (a) D. Seyferth, J. K. Heeren, G. Singh, S. O. Grim, and W. B. Hughes, J. Organomet. Chem., 1966, 5, 267; CrossRef (b) G. Stork and K. Zhao, Tetrahedron Lett., 1989, 30, 2173; CrossRef c) W. R. F. Goundry, J. E. Baldwin, and V. Lee, Tetrahedron, 2003, 59, 1719. CrossRef
54. A. Hamajima and M. Isobe, Angew. Chem. Int. Ed., 2009, 48, 2941. CrossRef