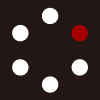
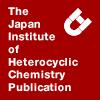
HETEROCYCLES
An International Journal for Reviews and Communications in Heterocyclic ChemistryWeb Edition ISSN: 1881-0942
Published online by The Japan Institute of Heterocyclic Chemistry
e-Journal
Full Text HTML
Received, 8th September, 2013, Accepted, 2nd December, 2013, Published online, 20th December, 2013.
DOI: 10.3987/REV-13-781
■ RECENT PROGRESS IN METAL ASSISTED MULTICOMPONENT SYNTHESES OF HETEROCYCLES
Pradip Kumar Maji,* Rafique Ul Islam,* and Sujit Kumar Bera
Department of Chemistry, Bidhan Chandra College , Asansol, Burdwan, 713304, WB, India
Abstract
This review highlights some remarkable recently made achievements in the application of metal-catalyzed processes to the design of Multicomponent syntheses of Heterocycles. The domino MCRs that are purely based upon metal catalysis opens a vast field for the discovery of new sequences in heterocyclic synthesis. Many of these methods have proven to be the most powerful and are currently applied in target- or diversity-oriented syntheses. These reactions can dramatically reduce the generation of chemical wastes, costs of starting materials, and the use of energy and manpower. Moreover, the reaction period can be substantially shortened. This review article aims at reporting the recent developments devoted to this important area, focusing on the metal catalysis in Multicomponent synthesis of heterocycles.CONTENTS
1. Introduction
2. Construction of five-membered heterocycles
2.1 Heterocycles containing one heteroatom
2.1.1 Synthesis of pyrrole, pyrroline and pyrrolidine derivatives
2.1.2 Synthesis of indoles, isoindoles, indolines and pyrroloindoles
2.1.3 Synthesis of indolizines and oxindoles
2.1.4 Synthesis of furans and furanones
2.1.5 Synthesis of benzofurans and bezofuranones
2.1.6 Synthesis of furobenzopyrane
2.2 Heterocycles containing two heteroatoms
2.2.1 Synthesis of pyrazole derivatives
2.2.2 Synthesis of imidazole and imidazopyridine derivatives
2.2.3 Synthesis of oxazole, isoxazole and oxazolidinone derivatives
2.2.4 Synthesis of dioxolanes and oxasilacycles
2.3 Heterocycles containing three heteroatoms
2.3.1 Synthesis of triazoles
2.4 Heterocycles containing four heteroatoms
2.4.1 Synthesis of tetrazoles
3. Construction of six-membered heterocycles
3.1 Heterocycles containing one heteroatom
3.1.1 Synthesis of pyridine and dihydropyridone derivatives
3.1.2 Synthesis of tetrahydroquinoline, quinoline and isoquinoline derivatives
3.1.3 Synthesis of quinolone and isoquinolone derivatives
3.1.4 Synthesis of pyran and lactone derivatives
3.2 Heterocycles containing two heteroatoms
3.2.1 Synthesis of pyrimidine and dihydropyrimidone derivatives
3.2.2 Synthesis of quinazolinone, quinoxaline and promazine derivatives
3.3 Heterocycles containing three heteroatoms
4. Construction of seven-membered heterocycles
5. Miscellaneous
6. Conclusion
7. Acknowledgement
8. References and Notes
1. INTRODUCTION
There are many heterocyclic frameworks which are widely distributed in nature. Various natural products, many dyestuffs and pigments contains heterocyclic core and they have wide range applications in medicinal field.1 Heterocycles are versatile building blocks in organic synthesis and by virtue of their multiple reactivity profiles and structures, these can be delicately manipulated to achieve required modification. Thus the syntheses of these heterocycles due to their fundamental importance in living system have attracted organic chemists towards the development of novel and more efficient synthetic strategies. Many of these strategies involve the formation of either carbon-carbon or carbon-heteroatom bonds from their corresponding acyclic precursors. Most of the classical methodology utilizes comparatively harsh reaction conditions and suffer various disadvantages such as use of expensive catalyst, elevated temperature, longer reaction time, multistep transformations, poor yield of the final product, tedious work-up etc. Considering the fundamental issues of chemo-, regio-, stereoselectivity, as well as economical and ecological aspects of green and sustainable chemistry,2 a novel, consise and efficient synthetic routes has become a demanding challenge among the synthetic community.3,4 Among several newly developed methodologies, the productive concept of diversity-oriented5 multicomponent reactions (MCRs) have gained a considerable attention among the organic chemists during the last decade. A multicomponent reaction (MCRs) is ideally a synthesis which starts from readily accessible components, proceeds fast and safe, environmentally benign operation, performing multiple steps in a single operation. In this synthesis, several bonds are formed in one sequence without isolating the intermediates in quantitative yield, and can directly build complicated molecules under mild conditions. Many MCRs are well suited too for the construction of various heterocyclic cores. Therefore MCR-based synthesis developed a sustainable use of chemical resources and reduced the amount of waste product by the use and disposal of harsh reactants and reagents as well as minimised the number of steps required for organic transformations.6 As a consequence, the design of novel MCRs and their exploration as tools in especially heterocyclic chemistry receive growing attention internationally. Novel MCRs are not only applied in combinatorial and medicinal chemistry but also in catalysis and more traditional natural products syntheses. This present brief review is entirely devoted to an updated summary of multicomponent reactions (MCRs) approaches towards the synthesis of heterocycles.
2. CONSTRUCTION OF FIVE-MEMBERED HETEROCYCLES
2.1 HETEROCYCLES CONTAINING ONE HETEROATOM
2.1.1 SYNTHESIS OF PYRROLE, PYRROLINE AND PYRROLIDINE DERIVATIVES
Pyrroles represent an important class of heterocycles in organic chemistry which are a structural units in many natural products and pharmaceuticals as well as a key intermediates for the synthesis of a variety of biologically active molecules and functional materials.7 Most of the conventional methods for the construction of a pyrrole ring are the Hantzsch reaction,8 the Paal-Knorr synthesis,9 and various cycloaddition methods.10 Although a number of metal-catalyzed approaches were also developed for the construction of pyrrole ring11 still, search for a general and an efficient synthetic strategies from simple and readily available precursors are of great value due to the great importance of the pyrrole core. Multicomponent synthesis of 4-iodopyrroles 1 using copper iodide and a catalytic amount of PdCl2(PPh3)2 from the reaction between acyl chlorides, N-Boc protected propargylamines and sodium iodide has been reported. The reacation is believed to be proceeded through a coupling/addition/cyclocondensation sequence. It was subsequently found that these 4-iodopyrroles, upon addition of an another alkyne, undergoes an in situ Sonogashira coupling to yield 2-substituted 4-alkynyl-N-Boc pyrroles 2 (Scheme 1).12
Multicomponent sequence that leads to pyrrole derivatives from the reaction between phenylacetylene, imines derived from ethyl glyoxylate and dialkylzinc derivatives has also been described (Scheme 2).13
Reactions between β-ketoesters or symmetrical β-diketones, diaroylacetylenes and ammonium acetate in the presence of indium trichloride afforded 3,3ˊ-bipyrrole 4 via a double enaminone formation-Michael addition sequence (Scheme 3).14
A one-pot, three component reaction between primary amines, β-ketoesters or β-diketones and propargyl alcohols afforded pyrroles 5 in the presence of the [Ru(η3-2-C3H4Me)(CO)-(dppf)][SbF5] system, where dppf is 1,10-bis(diphenylphosphanyl)ferrocene, in good to excellent yields (Scheme 4).15
dI2/KI has proved to be an efficient catalytic system for the direct synthesis of a variety of carbonylated heterocyclic system starting from suitably functionalized alkynes, by either a cyclocarbonylation or a cyclization-carbonylation reaction sequence. Synthesis of pyrrole-3-carboxylic acid ester derivatives starting from readily available building blocks is achieved by PdI2-catalyzed oxidative heterocyclization-alkoxycarbonylation of N-Boc-1-amino-3-yn-2-ols followed by basic treatment.16 Sonogashira cross-coupling and double cyclization strategy for the formation of hetero dimmers of heterocycles via C-H activation has recently reported by Ohe et al.17 Synthesis of a variety of 2-(2′-furyl)pyrroles 6, 2-(5′-thienyl) furans and 2-(5′-thienyl) pyrroles 7 under mild condition involves several C-C and C-N bond formation in the presence of Pd and Cu catalyst (Scheme 5).
An efficient one-pot three component reaction of primary amines, dialkyl acetylenedicarboxylates, and nitrostyrene derivatives in the presence of FeCl3, leads to tetrasubstituted polyfunctional pyrroles in high yields which follows a sequence of domino Michael addition/cyclization process.18 Regioselective synthesis of polysubstituted pyrroles 8 via the copper-catalyzed three component reaction of diazoketones, nitroalkenes, and amines under aerobic conditions which involves an N-H insertion of carbene, a copper-catalyzed oxidative dehydrogenation of amine, and a [3+2] cycloaddition of azomethine ylide has been reported (Scheme 6).19
Pyrrolo[3,2-d]pyridazines are a class of interesting and useful N-heterocycles with a limited report of synthetic methods. Most of the known pyrrolo[3,2-d]pyridazines were synthesized via condensation of pyrrole-2,3-diones with hydrazine. Recently, one-pot multicomponent synthesis of pyrrolo[3,2-d]-pyridazine derivatives 9 via zirconocene-mediated cyclization of one equivalent of Si-tethered diyne, two equivalents of nitriles, and one equivalent of an azide have reported. Instead of normal azides, if trimethylsilyl azides are used, interestingly the same synthetic procedure affords pyrrole-2,3-diones 10. Further, these functionalized pyrrole-2,3-diones could be transformed into pyrrole-fused heterocycles (Scheme 7).20
Rhodium(II)-catalyzed multicomponent reaction of an imine, diazoacetonitrile (DAN) and an activated alkynyl coupling partner, e.g., dimethyl acetylenedicarboxylate (DMAD), for the synthesis of substituted 1,2-diarylpyrroles has been reported.21 Mechanistically, the diazo compound produces the corresponding metallocarbenoid in the presence of a rhodium(II) salt. The imine then reacts with this electron-deficient metallocarbenoid to generate an azomethine ylide 11. Finally, this 1,3-dipole undergoes a [3+2] cycloaddition with the activated alkynyl dipolarophiles and subsequent elimination to afford the 1,2-diarylsubstituted pyrrole 12 (Scheme 8).
Three component coupling of 1,3-diketones, aldehydes and amines in the presence of low-valent titanium as a catalyst afforded the pyrrole derivatives 13 (Scheme 9).22 TiCl4/Sm gave the best results among different types of low-valent titanium systems such as TiCl4/Sm, TiCl4/Zn, TiCl4/Al, or TiCl4/Mg studied as reagents for this reaction. This protocol highlighted the wide scope of this three component reaction, applicable not only to aromatic aldehydes and aromatic amines bearing either electron-withdrawing or -donating groups, but also to heterocyclic or aliphatic aldehydes and aliphatic amines.
An InCl3-catalyzed one-pot three component synthesis of 2-pyrrolo-3′-yloxindoles, 14 by a sequential Michael addition followed by Paal-Knorr condensation was achieved (Scheme 10). Multicomponent reaction of 3-phenacylideneoxindole, β-ketoester, and ammonium acetate at refluxing condition in ethanol afforded the products in good yields.23
The catalytic efficacy of NiCl2∙6H2O has been demonstrated through four component reaction24 of amine, β-ketoesters or 1,3-dicarbonyl compounds, various aldehydes and nitroalkanes for the sysnthesis of tetrasubstituted pyrrole 15 (Scheme11). It is also reported the binding interaction studies of synthesized pyrrole derivatives with different enzymes.
2.1.2 SYNTHESIS OF INDOLES, ISOINDOLES, INDOLINES AND PYRROLOINDOLES
The indole core is reknowned as a “privileged motif” because of its presence in many natural products and pharmaceuticals.25 Much effort has been paid toward the synthesis and functionalization of indoles, and methods for the preparation have been well developed.26-29 Classical routes for indole formation includes Fischer synthesis27, Batcho-Leimgruber synthesis, Gassman synthesis, reductive cyclization28 and more recently, transition metal catalysis and multicomponent reactions have also been widely applied.29 Indoles 16 from haloalkenes, amines and o-dihaloarenes promoted by palldium-catalyst cascade process has been developed.30 It is important to note that in this three component reaction, the palladium-catalyst promotes three different and independent reactions: 1) formation of the imine by alkenyl amination 2) α-arylation of the imine and 3) intramolecular N-arylation. The key in the success of this cascade process is the high chemoselectivity of the different cross-coupling events. Thus, the higher reactivity of the bromoalkenes was compared with haloarenes in the oxidative addition to palladium to permit the formation of 17, instead of the arylamination reaction. The dihaloarene is incorporated in the second step only when all the alkenyl halide has been consumed (Scheme 12).
Literature reveals the wide developed of copper-catalyzed multicomponent reaction of a sulfonyl azide, a terminal alkyne and a nucleophile which involves azide-alkyne cycloaddition reaction generating a ketene amine intermediate through a ring opening rearrangement of triazoles. Recently Wu et al.31 reported the synthesis of a variety of polysubstituted indole derivatives 18 by using copper-catalyzed multicomponent reaction of ethynylaniline, sulfonyl azide and nitroolefines in the presence of a base triethylamine at room temperature involving a ketenimine intermediate through a ring opening rearrangement of triazoles (Scheme 13).
Synthesis of benzo[f]pyrido[1,2-a]indole-6,11-dione derivatives 19 via copper(II)-catalyzed three component reactions of acyl bromide, 1,4-naphthoquinone, and pyridine (or isoquinoline) is reported.32 The reaction proceeds via a sp2C−H difunctionalization of naphthoquinone followed by intramolecular cyclization and oxidative aromatization. Use of 1,3-dicarbonyl compounds instead of acyl bromide also afford benzo-fused pyridoindole derivatives in excellent yields (Scheme 14).33
Azaindole nucleus are known to be part of many biologically active compounds, occupying a unique position in medicinal and pharmaceutical chemistry and also serve as building blocks in synthetic chemistry.34 One-pot multicomponent reaction of an amine, aldehyde and a terminal alkyne for the synthesis of a range of quinoline derivatives linked to azaindole 20 by a Povarov reaction using SnCl2, a cheap Lewis acid is described (Scheme 15).35
A variety of α-alkynyl indoles 21 have been synthesized36 through constructing one Csp2-Csp and two Csp2-N bond by a novel and an efficient multicomponent reaction of o-bromo(dibromovinyl)benzenes, terminal alkynes and arylamines using palladium as catalyst (Scheme 16).
Ketenimine,37 as an useful intermediate, has attracted much attention due to its easy formation, relative reactivity, and diverse chemistry.38 The most attractive and sustainable method generating ketenimines could be attributed to the copper-catalyzed azide-alkyne cycloaddition (CuAAC) which is established by Fokin et al.39 Various groups40 have developed a number of multicomponent reactions by trapping ketenimines generated in situ from sulfonyl azides and terminal alkynes via CuAAC reaction. Wang et al.41 has described recently a copper-catalyzed three component cascade reaction of sulfonyl azides, 2-bromophenylacetylenes and amines, which furnished 2-sulfonyliminoindolines 22 in moderate to good yields (Scheme 17).
Copper(I)-catalyzed domino reaction provides pyrroloindole 23 and dipyrroloindole derivatives 24 from 4,6-diethynyl-1,3-phenylenediamine or its pyridine congener with paraformaldehyde and secondary amines (Scheme 18).42 The controlled Mannich-type reaction and cyclization of 4,6-diethynyl-1,3-phenylenediamine or its pyridine congener with paraformaldehyde and secondary amines in the presence of copper catalyst leads to the formation of mono- or bis(aminomethylated) pyrroloindoles 23 (or 24) in moderate to excellent yields.
2.1.3 SYNTHESIS OF INDOLIZINES AND OXINDOLES
Multicomponent coupling/cycloisomerisation reaction of heteroarylaldehydes, amines, and alkynes under solvent free conditions or in water, in the presence of a gold catalyst, provides rapid access to substituted aminoindolizines 25 (Scheme 19).43
Biologically important aminoindolizine framework 26 has been synthesized44 by a facile, atom economic, one-pot multicomponent reaction of pyridine-2-carboxaldehyde or quinoline-2-carboxaldehyde, secondary amine and terminal alkyne using CuCl as catalyst in refluxing condition at PEG. This methodology demands green credentials due to use of non-toxic, inexpensive catalyst and green solvent (Scheme 20).
Oxindoles and its derivatives occupy a key place among the various classes of heterocyclic organic compounds45 that possess a common basic framework in natural products46 and pharmaceutically active compounds. Substituted oxindoles have recently attracted attention due to the utility of such structure in the development of biologically active compounds as well as new drugs. A palladium-catalyzed three component synthesis of unsymmetrically substituted 3-(diarylmethylene)indolin-2-ones 27 in good yield starting from aryl bromides, alkyl propiolamides and aryl iodides has been described.47 The reaction involved a sequence of N-arylation, carbopalladation, C-H activation, and carbon-carbon bond formation, and the oxindoles were produced as both E- and Z-isomers with up to 1:4 (E/Z) regioselectivity (Scheme 21).
Multicomponent reaction using [Pd(PPh3)4] as a catalyst in the presence of triphenylphosphine from 2-iodoaniline, phenylacetylene and carbon monoxide provides 2-benzylideneindoxyl 28 as a sole product. This procedure is extended to a variety of indoxyls, mainly obtained as the (Z)-isomer (Scheme 22).48 On the other hand, 2-substituted 4-quinolones 29 are obtained through a one-pot two-step phosphine free multi-catalysis using sequentially [PdCl2(dppp)] and HNEt2 as catalysts. An extensive study varying the nature of the palladium catalysts, ligands, bases, additives and solvents has also been reported to elucidate factors controlling the selectivity toward 2-substituted 4-quinolones.
Transition metal-catalyzed carbonylation reactions, among them mainly palladium-catalyzed amino and alkoxycarbonylation and carbonylative coupling reactions, are well documented and widely used in the area of synthetic chemistry. 2-Bromobenzaldehyde undergoes carbonylative cyclization with aromatic and aliphatic primary amines under carbon monoxide pressure in the presence of a palladium catalyst to give isoindolin-1-ones 31.49 Recently, a highly chemoselective intramolecular cycloaminocarbonylation of bifunctional 2-iodobenzyl-bromide towards the similar N-substituted 1-isoindolinones is reported.50 When 2-iodobenzylbromide in place of 2-bromobenzaldehyde is reacted with various primary amines, aniline under atmospheric carbon monoxide pressure in DMF in the presence Pd(0) catalysts generated in situ from Pd(II) acetate catalytic precursor the same 1-isoindolinone derivatives 31 is obtained in good yields. They have also reported51 a three component palladium-catalyzed cascade hydrazinocarbonylation reaction of iodoarenes, carbon monoxide and various hydrazine derivatives for the formation of tetrahydrophthalazine and phthalamide derivatives (Scheme 23).
2.1.4 SYNTHESIS OF FURANS AND FURANONES
Concise synthesis of 4,5-subsituted γ-butyrolactones 32 from dimethyl itaconate, aromatic aldehyde, and aryl bromides via a multicomponent one-pot reaction in the presence of zinc dust and cobalt bromide is described. As per their report52 various aldehyde bearing an electron-withdrawing and electron-donating groups provides satisfactory yields except 3-pyridinecarboxaldehyde. Mechanistically this cascade reaction involves the following steps: the formation of zinc reagent, Michael addition, aldol condensation and cyclization to provide the product (Scheme 24).
A novel carbon dioxide triggered and copper-catalyzed domino reaction for the efficient synthesis of highly substituted 3(2H)-furanones 33 from nitriles and propargylic alcohols has been developed.53 Carbon dioxide is a prerequisite for achieving the present catalytic transformation, and one of the oxygen atoms of carbon dioxide is inserted into the 3(2H)-furanones (Scheme 25). Nitrile acts as the reaction solvent as well as the reactant and copper salts play dual roles of activating both the propargylic alcohols and nitriles. An increase in temperature from room temperature to 70 oC dramatically increased the yield from 8% to 82%.
Transition metal-catalyzed CO2 activation reactions are widely studied for the formation of new carbon-carbon bonds. Highly regio- and stereoselective methyl-carboxylation of homopropargylic alcohols with ZnMe2 and CO2 for the synthesis of alkylidene-γ-butyrolactones 34 catalyzed by Ni(0) is described (Scheme 26).54
2.1.5 SYNTHESIS OF BENZOFURANS AND BENZOFURANONES
A copper-catalyzed multicomponent coupling of salicylaldehydes, amines, and alkynes dihydrobenzofurans has been reported.55 However; the alkynes employed are limited to those aliphatic alkynes bearing a heteroatom, such as propargyl alcohols or propargylamines in most cases producing dihydrobenzofuran as a major product. Recently copper-catalyzed three component reaction of salicyldehydes, amines and alkynes for the construction of benzofuran has been demonstrated.56 Under this report, aliphatic alkynes bearing a heteroatom, such as propargyl alcohols or propargylamines in most cases produced benzofuran 35 in stead of dihydrobenzofuran from this novel cascade A3-coupling-annulation reaction. Similar multifunctionalized benzofuran derivatives have been synthesized from the multicomponent coupling reaction of alkynylsilane, salicylaldehyde and secondary amine using Cu(I)-Cu(II) combined catalysts in moderate to excellent yield. Yield of the products are largely depends upon the ratio of amine used as a component. According to the report, high loading of the catalyst (10 %) also decreases the yield of the products due to coordination of the catalyst with amine, with deactivation of the neucleophilicity of the amine. Mechanistically the reaction proceeds via the formation of copper acetylide from alkynylsilane, and in situ generation of iminium intermediate from aldehyde and amine provides propargylamine, which on intramolecular neucleophilic attack by hydroxy group via 5-exo-dig mode leads to the benzofuran derivatives 35 (Scheme 27).57
2.1.6 SYNTHESIS OF FUROBENZOPYRANE
Palladium-catalyzed cascade 1,4-addition and cyclization for the synthesis of furochromenes 36 is developed. Suitability of various bases, DIPEA is proved to be the best for this coupling reaction. Compared to other aryl iodides, those having electron-withdrawing functional group favour the faster oxidative addition and afford the corresponding products. Better yields are obtained depending upon the electron-donating nature of R1 group in arynyl moieties of chromones (Scheme 28).58
2.2 HETEROCYCLES CONTAINING TWO HETEROATOMS
2.2.1 SYNTHESIS OF PYRAZOLE DERIVATIVES
Pyrazoles, five-membered heterocycles with two adjacent nitrogen atoms, display a rich chemistry having various biological and medicinal applications.59-61 A tandem reaction for the synthesis of pyrazole derivatives has been developed 62 from an acid chloride, terminal alkynes and hydrazine hydrate using palladium and copper catalyst under conventional heating in acetonitrile. Acid chloride is coupled with terminal alkynes to give α,β-unsaturated ynones and converted in situ into pyrazoles 37 by the cycloaddition of hydrazines (Scheme 29).
Müller et al. have synthesized the pyrazoles 37 in good to excellent yields via one-pot two-step sequential reactions in the presence of palladium catalyst under microwave heating. After formation of alkynones, hydrazines and acetic acid are reacted in the same reaction vessel under microwave irradiation. Best results for the formation of pyrazoles are obtained by heating in the microwave oven at 150 °C for 10 min in the presence of methanol (Scheme 29).63
Recently the same group has reported64 one-pot four-step syntheses of tetrasubstituted pyrazoles based on consecutive sequence of Sonogashira cross-coupling, cyclocondensation, halogenation and Suzuki cross-coupling reaction using the same palladium-based catalyst. Acid chloride reacts with terminal alkyne followed by cyclocondensation with methyl hydrazine which affords the intermediate pyrazole ring. Bromination using NBS and Suzuki cross-coupling with boronic acid finally gives highly fluorescent 1,2,3,4-tetrasubstituted pyrazoles (Scheme 30).
Fully substituted pyrazoles 39 via one-pot three component reaction of aldehydes, phenylhydrazine, and 1,3-dicarbonyl compounds using Yb(PFO)3 (ytterbium perfluorooctanonate) as catalyst under solvent free conditions have been developed (Scheme 31).65
Coupling of a primary amine, an alkyne, and isonitrile followed by treatment with hydrazines yield pyrazoles 40 via titanium-catalyzed three component reaction. Modifications of the catalyst results in good control of regioselectivity for some of the substrates (Scheme 32).66
Singh et al.67 developed an efficient, atom economy, simple environmentally benign solvent free microwave-assisted multicomponent synthesis of pyrazole derivatives 41 from phenyl hydrazine, aldehyde and ethyl acetoacetate using Sc(OTf)3 as a reusable catalyst (Scheme 33).
Lee et al.68 has recently reported one-pot three component reaction of a primary amine, 2-bromobenzaldehyde and sodium azide catalyzed by Cu(I) salt by consecutive condensation, C-N and N-N bond formation for the preparation of 2H-indazoles 42. On screening with various metal salts of Fe, Pd, Ni and Co, they have found that only CuI is successful to afford the reaction product. C-N bond formation takes place between the aryl bromide and the azide. The Azide group is then activated by means of co-ordination of terminal nitrogen atom of the azide to the copper catalyst which attacks the imine adduct of aniline and bromobenzaldehyde resulting the N-N bond formation (Scheme 34).69
Transition metal-catalyzed cascade reaction of an alkyne, aldehydes and amines and subsequent nucleophilic cyclization onto an alkyne moiety affords various heterocyclic systems. Gold-catalyzed annualation for the direct and regioselective synthesis of polysubstituted dihydropyrazoles 43 from an alkyne, hydrazines and carbonyl compounds is recently reported by Ohno et al. (Scheme 35).70
Four component coupling reaction in the presence of palladium catalyst from a wide range of halides, terminal alkynes, molybdenum hexacarbonyl and hydrazine has been described for the construction of highly substituted pyrazole derivatives 44 and 45 as shown in the Scheme 36.71 It is interesting to note that Mo(CO)6 has been used to overcome the limitation of using toxic CO.
An efficient, novel and green multicomponent approach is described72 for the synthesis of fully substituted pyrazole 46a from the mixture of aldehyde, arylhydrazine and ethylacetoacetate in the presence of Zn(OTf)2 heated under solvent free condition. It is also described the synthesis of highly substituted bispyrazoles 46b where 1,4- or 1,3-dialdehyde is used instead of a monoaldehyde (Scheme 37).
Wang et al. reported73 an one-pot copper-catalyzed synthesis of 5-sulfonamidopyrazoles 47 from three component reaction of terminal alkynes, sulfonyl azides and hydrazones through a Lewis acid-catalyzed electrocyclic reaction and a dehydrogenation (Scheme 38).
Synthesis of 2-amino-H-pyrazolo[5,1-a]isoquinolines 48 via a silver(I)-catalyzed and copper(I) as a co-catalyst three component reaction of Nˊ-(2-alkynylbenzylidene)-hydrazide, alkyne, and sulfonyl azide have been described recently (Scheme 39).74
2.2.2 SYNTHESIS OF IMIDAZOLE AND IMIDAZOPYRIDINE DERIVATIVES
Imidazole and its derivatives are an important class of heterocyclic compounds, which are widely used in biology as inhibitors,75 in medicinal chemistry76 and also as functionalized materials.77 Classical methods for the synthesis of imidazoles includes Debus-Radziszewski imidazole synthesis,78 Weidenhagen imidazole synthesis,79 and Van Leusen imidazole synthesis.80 Recently, a number of multicomponent syntheses of imidazoles have also been developed.81 Nonetheless, development of more efficient and versatile approaches to achieve functionalized imidazoles are still remains very important. The utility of InCl3.3H2O protocol for the multicomponent synthesis of 2,4,5-trisubstituted imidazoles 49 has been explored. Highly polar methanol played an important role and beneficial for increasing the yield of the product compared to low polar ethanol, iso-propanol and t-butanol. Various other solvents like acetonitrile, chloroform, dichloroform and toluene have found to be ineffective for the system (Scheme 40). Initially indium catalyst increases the electrophilicity of carbonyl group of aldehyde to facilitates the formation of diamine which condensed to benzil followed by rearrangement and [1,5] hydrogen shift to furnish the product.82
Li and Pan have recently reported the CuI-PPh3-catalyzed multicomponent approach for the synthesis of substituted imidazolines 50.83 The scope of the reaction has been investigated to a variety of olefins, including α,β-unsaturated ketones, α,β-unsaturated esters, and simple alkenes (Scheme 41).
A palladium-catalyzed multicomponent synthesis of imidazolinium carboxylates 51 and imidazolines 52 from a coupling of two imines, acid chloride, and carbon monoxide is described by Arndtsen et al. Imidazolines are produced via the initial generation of munchnone intermediates, followed by their cycloaddition with an in situ generated protonated imine (Scheme 42).84
Imidazoles are important class of small molecules found in many biological active compounds, useful in coordination chemistry, serving as N-donor ligands in various metal complexes or as precursors to N-heterocyclic carbenes. Palladium-catalyzed multicomponent synthesis of imidazolinium carboxylates from imines, acid chlorides and CO through a carbonylation followed by a thermal decarboxylation has been reported.85 Recently Arndtsen et al.86 has reported that a palladium-catalyzed multicomponent synthesis of 2-imidazoline proceeds via the coupling of imines, acid chlorides and carbon monoxide to form imidazolinium carboxylates, followed by a decarboxylation. Although decarboxylation in the presence of CHCl3 is found to result in a mixture of imidazolinium and imidazolium salts but the addition of benzoic acid selectively generates the trans-disubstituted imidazolines in good yield by suppressing aromatization.
Tetrasubstituted imidazoles 53 can be synthesised from propargylamines, sulfonyl azides, and terminal alkynes by generating ketenimines as the intermediate via a copper-catalyzed azide-alkyne cycloaddition and cascade cyclization (Scheme 43).87
Microwave-assisted one-pot four component coupling of 2-aminopyridine-5-boronic acid pinacol ester, aldehyde and isocyanide through a stepwise sequence (Scheme 44) to 3-amino-imidazopyridines 54 is described.88 Mixture containing MgCl2 (Ugi Lewis catalyst) and Pd(dppf)Cl2 (Suzuki catalyst) is failed to produce any desire product under one-pot reaction condition. First Ugi condensation product is formed from 2-aminopyridine-5-boronic acid pinacol ester which then converted into the desired product in the presence of Suzuki catalyst.
Ugi type Groebke-Blackburn-Bienayme three component reaction89 of 2-aminopyridine or aminoimidazothiazole, aldehyde and isocyanide under solvent free condition using maghemite nanoparticles (γ-Fe2O3@SiO2-OSO3H) leads to the formation of biologically interest aminoimidazopyridine or aminoimidazothiazole derivatives 55 in good yields (Scheme 45).
Coupling of 2-aminopyridine, aldehyde and teminal alkyne providing imidazopyridines 56 through one-pot three component reactions in the presence of indium tribromide as catalyst in good yield has been described.90 In situ formation of imine from aldehyde and 2-aminopyridine followed by addition of alkyne which is activated by indium catalyst and deprotonation by triethylamine afforded product 56 (Scheme 46).
A novel three component reaction of 2-aminopyridines, aldehydes and alkynes in the presence of CuSO4/TsOH as catalyst (Scheme 47) towards the synthesis of imidazo[1,2-a]pyridines 57 has been described.91 The co-catalyst is a strong Bronasted acid such as TsOH which facilitates the protonation of imine and hence to some extent activates the neucleophilic addition of the alkyne to imine. Another role of the co-catalyst is to prevent the catalyst from the co-ordination with nitrogen atom of 2-aminopyridine. This methodology is ineffective for functional groups on aromatic moieties bearing hydroxyl, dialkylamino, and bromo functionalities. Another reports on the synthesis of imidazo[1,2-a]pyridines 57 and the mechanistic study of its formation through one-pot multicomponent cascade reaction using the same components in the presence of copper(I) iodide combined with NaHSO4.SiO2 as co-catalyst refluxing in toluene has been reported (Scheme 47).92 Recently a facile and straightforward synthesis of imidazopyridines 57 via one-pot multicomponent reaction of 2-aminopyridine, various aldehydes and terminal alkynes using a magnetic nano-Fe3O4 catalyst with KHSO4.SiO2 as additive in anhydrous toluene at refluxing condition has been achieved.93 It is also reported the recovery, reusability and activity of catalyst for new catalytic cycle (Scheme 47).
The multicomponent reaction of aldehyde with amine and alkyne (A3-coupling) and its incorporation in cascade processes of tethered cyclization with activation of triple bond in propargylamine affords an access to versatile molecular complex compounds. The coinage metal (Cu, Ag, and Au) salts are efficient enough in catalysis for alkyne C−H activation in alkynylation with σ-activated imine and π-activation of triple bond toward cyclization. A mixed Cu(I)−Cu(II) system in situ generated from partial reduction of CuSO4 with glucose can be used in ethanol (nonanhydrous) under open air, that efficiently catalyzes the multicomponent reaction of heterocyclic azine and aldehyde with alkyne followed by cycloisomerization to afford N-fused imidazoles 58 (Scheme 48).94
Utilization of sodium azide as a source of nitrogen for the synthesis of benzimidazoles 59 by the copper-catalyzed, one-pot, three component reaction of 2-haloanilines, aldehydes, and sodium azide have been described very recently (Scheme 49).95
2.2.3 SYNTHESIS OF OXAZOLE, ISOXAZOLE, OXAZOLIDINONE DERIVATIVES
The isoxazoles 60 are obtained in moderate to excellent yields from one-pot coupling-cycloaddition reaction in the presence of PdCl2(PPh3)2 and CuI catalytic system. Modified Sonogashira coupling of acid chlorides with terminal alkynes furnished the expected alkynones, which subsequently, on microwave irradiation with hydroximinoyl chlorides in the presence of triethylamine underwent 1,3-dipolar cycloaddition to afford the isoxazoles (Scheme 50).96
Multicomponent reactions have the advantages over conventional multistep sequences which includes the savings the costs of reagents and solvents, along with other materials required for purification and isolation. Palladium-catalyzed three component reactions of an aryl halide, isocyanide, and an amino alcohol for the formation of oxazolines 61 and benzoxazoles 62 in excellent yield is reported (Scheme 51).97
An efficient one-pot aminocarbonylation and cyclocarbonylation for the synthesis of 2-oxazolidinones 63 has been described.98 The one-pot method consisted of the reaction of α,α-disubstituted 2-ynylamines with CO, O2, and morpholine in the presence of catalytic amounts of PdI2 in conjunction with KI and H2O (PdI2/KI/1/2/H2O molar ratio1:10:100:500:500), in DME as the solvent at 100 oC under 20 atm of a 4:1 mixture of CO/air afforded 63 in excellent yield. The reaction in the presence of secondary amine like piperidine and diethylamine also proceeded smoothly with good yield of oxazolidiones. In the case of an α-monosubstituted propargylamine, such as benzyl-[1-(1-ethylpropyl)prop-2-ynyl]amine under the same reaction conditions, the initially formed oxazolidinone undergoes a spontaneous double bond shift with the formation of 3H-oxazol-2-one derivative 64 in excellent yield (Scheme 52).
Imine obtained from condensation of aldehyde and amine in the presence of CuI catalyst reacts with acetylide to form propargylamine derivatives which on cyclization with carbon dioxide furnishes the 3,4,5-trisubstituted 1,3-oxazolidin-2-one 65 quantitatively (Scheme 53).99
Inexpensive and reusuable ligand free CuO nanoparticles catalyzed three component reaction100 of 2-iodoaniline, carbon disulfide and amine to synthesize N-substituted benzothiazole derivative 66 in good yield has been described recently (Scheme 54).
A domino condensation/S-arylation/heterocyclization process is described101 for the synthesis of benzothiazoles 67 usning Cu(I) catalyst. Condensation of carbon disulfide with thiols in the presence of K2CO3 generates carbonotrithioate salts in situ, which then undergoes coupling with 2-iodoanilines and subsequent intramolecular condensation and elimination under the assistance of CuBr to afford 2-thiosubstituted benzothiazole derivatives (Scheme 55).
An efficient and convenient multicomponent reaction has been developed by Majumdar et al.102 Different o-haloamine compounds, carbon disulfide, and various amines in the presence of copper salt and potassium carbonate as a base undergoes multicomponent reaction and afforded 2-aminothiazole derivatives 68 in good yields. Initially carbon disulfide and amine formed unstable dithiocarbamic acids which readily formed potassium salt of dithicarbamate. Then Ullmann type coupling between o- haloamines and potassium salt of dithiocarbamate followed by elimination of hydrogen sulfide gives the desired products (Scheme 56).
2.2.4 SYNTHESIS OF DIOXALANES AND OXASILACYCLES
Chemo- and diastereoselective synthesis of spiro-indolodioxolanes 69 by three component reaction from cyclic diazoamides, one mole of electron-donating and one mole of electron-withdrawing aldehyde in the presence of rhodium acetate as a catalyst at room temperature has been reported.103 Electron-deficient carbenoid carbon of cyclic rhodium carbeniod which is produced from cyclic diazoamide chemoselectively reacts with electron-donating aldehyde to form carbonyl ylide. Subsequent 1,3-dipolar cycloaddition of the ylide with electron-withdrawing aromatic aldehyde which acts as dipolarophiles, diastereoselectively afforded dioxolanes (Scheme 57).
Nickel(0)-catalyzed asymmetric formation of oxasilacyclic derivatives via three component cycloaddition is first reported104 by Baxter et al. Oxasilacycles 70 are synthetically useful and can be prepared by oxidative cyclization of aldehyde, alkyne and dialkylsilane in the presence of Ni(0) catalyst (Scheme 58).
2.3 HETEROCYCLES CONTAINING THREE HETEROATOMS
2.3.1 SYNTHESIS OF TRIAZOLES
The Copper-catalyzed Huisgen 1,3-dipolar cycloaddition of azides and alkynes for the synthesis of 1,4-disubstituted 1,2,3-triazoles has become very popular among the synthetic chemist since the discovery by the groups of Meldal and Sharpless.105-108 The superb reliability of the CuAAC reaction has increasingly attracted the attention from a diverse range of the areas of chemistry.109 As a result, various modifications have been developed to increase efficiency. Among them, use of supported and unsupported metal nanoparticles as an heterogeneous catalyst109-110 and use of green and ecofriendly conditions are well investigated.111 One of the important modification is the multicompnent reaction112 in which organic azides are generated in situ from azide precursor, sodium azide and an alkyne by minimising the hazardness from the isolation and handling of azides and also by avoiding one additional step. Reports on the copper-catalyzed multicomponent synthesis of triazoles from organic halides in water, using heterogeneous catalysts, have described in the literature.113 A chemo- and regioselective one-pot, four component coupling of alkynes with sodium azide has been developed for an elegant modular synthesis of fully substituted 1,2,3-triazoles 71 (Scheme 59).114 This protocol is expected to find extensive applications as it allows for the use of alkyl- and aryl-substituted alkynes.
Copper(I)-catalyzed three component cycloaddition reaction of alkynes with sodium azide and formaldehyde to furnish 2-hydroxymethyl-2H-1,2,3-triazoles 72 which are versatile synthetic precursors that allow an easy access to a wide variety of triazole derivatives, is reported by Fokin et al. (Scheme 60).115
Copper triflate has also been used as a catalyst for both azidation of benzylic acetates and subsequent click reaction in one-pot (Scheme 61).116
Copper triflate has also been used as a catalyst for both azidation of benzylic acetates andAliphatic or aromatic primary amines in conjunction with TfN3 and copper sulfate as catalyst has been used in one-step and sequential one-step procedures117 for diazo transfer and azide alkyne cycloaddition reaction for the synthesis of triazoles derivatives 74 (Scheme 62).
Recently a practical and efficient one-pot diazotization-azidation procedure for the synthesis of 1,4-substituted triazoles 75 utilizing t-BuONO and TMSN3 with amines have developed.118 Microwave irradiation is found to be significantly enhanced the rate of the formation of the products 75.119 This methodology smoothly proceeded with electron-deficient anilines and aromatic as well as aliphatic alkynes bearing electron-rich and electron-deficient functionalities (Scheme 63).
Multicomponent click reaction of epoxide, sodium azide and alkyne for the preparation of β-hydroxytriazole derivatives 76 has been reported (Scheme 64).120
Copper nanoparticles on various support have been found to effectively catalyze the click reaction affording 1,2,3-triazoles in excellent yield with minimum catalyst loadings.121 Recently, Yus et al. reported122 the use of copper nanoparticles which can catalyze the multicomponent synthesis of triazoles 77 and 78 from epoxides. A wide range of β-hydroxytriazoles have been synthesized from epoxides, sodium azide, and terminal alkynes with Cu-NPs suppoted on actived carbon as an inexpensive, safe catalyst and under green solvent as water (Scheme 65).
Phthalazine derivatives, constituting a bridgehead hydrazine, have received considerable attention in the literature as a consequence of their role as pharmacophores and their exciting biological properties.123a,b The recent protocols directed toward designing structural motifs containing the phthalazine ring fragment with 1,2,3-triazole moiety, employed an one-pot four component condensation strategy from the reaction of aromatic propargyloxy aldehydes, azides, dimedone/1,3-cyclohexanedione, and phthalhydrazide in high yield and excellent atom economy (Scheme 66).123c
Coupling of secondary alcohols, alkynes and trimethylsilyl azide affording disubstituted triazoles has been reported. Under this multicomponent click reaction copper catalyst facilitates nucleophilic substitution of alcohols with TMSN3 to form azides and also activates alkynes resulting 1,3-dipolar cycloaddition (Scheme 67).124
CuAAC “click and activate” strategy presents a powerful approach in diversity-oriented, multiple component condensation sequences that led to a trisubstituted triazolylpyridazinone 81 from 2-substituted 4,5-dihalopyridazinones, an azide and a terminal alkyne.125 The regioselective triazole formation not only stitches together an alkyne and an azide but also switches the reactivity of the neighboring group, triggering the facile in situ introduction of a nucleophile to it. The formation of the triazole should switch the reactivity of the 4-(chloro) position from a “neutral” or “deactivated” to an “activated” state toward nucleophilic attack due to the subtle electronic effect of triazole (Scheme 68).
Usually phenol and aromatic aldehyde is ineffective for this click reaction. A multicomponent, one-pot approach for the synthesis of α-alkoxytriazoles 82 from aldehydes, alcohols, azides, and alkynes via a four component reaction, proceeding via the formation of hemi-acetal followed by azidation and then click reaction in the presence of Cu(II) salt and metallic copper in acetonitrile has been reported (Scheme 69).126
A convenient route for the synthesis of 1,2,3-triazole-linked glycoconjugates 83 and 84 from gluclals, trimethylsilyl azide and alkynes via a tandem Ferrier and click reaction has been developed. The reaction is believed to proceed via Frrier rearrangement followed by [3+2] cycloaddition (Scheme 70).127
Microwave-assisted multicomponent reaction of substituted benzyl halide, sodium azide and o-trimethylsilylphenyl triflate undergoes multicomponent reaction in the presence of cesium fluoride to produce benzotriazole 85 has been described. In this reaction in situ aryne is generated from o-trimethylsilylphenyl triflate which undergoes click reaction to provide benzotriazole derivative (Scheme 71).128
A click reaction for the synthesis of triazole derivative 86 has been developed129 from benzyl bromide, phenylacetylene and sodium azide using CuFe2O4 nanocomposite as a catalyst in aqueous medium at 70 oC. The reusability of the catalyst is also examined (Scheme 72). Same component in the presence of Cu2O catalayst undergoes multicomponent click reaction leading to the formation of 5-alkynyl-1,2,3-triazole derivatives 87 under room temperature130 in place of triazole derivative 86. They have also reported the incorporation of deuterium in the 5-position of the 1,2,3-triazole to disclose the mechanistic path for the formation of product 88 by changing the solvent as CD3OD (Scheme 72).
A practical synthesis of triazole derivative in good yield through one-pot three component131 reaction of (arylethylene)trimethylsilanes, alkyl bromides and sodium azide using copper sulphate as catalyst by Huisgen cycloaddtion reaction is developed. The reaction undergoes in situ alkyl azide formation, alkyne deportection followed by cycloaddition for the formation of 1,4-disubstituted 1,2,3-triazole 89 as major product and 1,5-regioisomeric cycloadduct 90 as minor product (Scheme 73).
Sequential one-pot microwave irradiated multicomponent reaction catalyzed by copper is described.132 Glycosides, alkynes and TMSN3 in the presence of Cu(OTf)2 and KI under microwave irradiation provides triazole-O-glycoconjugates 91 and 92 via Huisgen 1,3-dipolar cycloaddition reaction (Scheme 74).
erminal acetylene, carbon monoxide, aryl iodide and sodium azide under multicomponent carbonylative Sonograshira reaction provides 4,5-disubstituted 1,2,3-(NH)-triazoles 93 and 94 in the presence of palladium catalyst.133 It is interesting to note that carboxide-free 4,5-disubstituted 1,2,3-(NH)-triazoles are obtained instead of 4,5-disubstituted 1,2,3-(NH)-triazoles containing carbonyl group when aryl iodide with nitro group is taken, due to the strong electrophilic nature of nitro group of aryl iodide directed to Sonograshira coupling with terminal acetylenes instead of carbonylative Sonogashira reaction. Carbonylative Sonograshira coupling and subsequent 1,3-dipolar cycloaddition leads to the formation of the product. Substituent effect of acetylene is also examined and found that the branch chain aliphatic terminal acetylene smoothly undergoes this reaction, even long chain aliphatic terminal acetylene can promote the process and produce better yield. On the other hand, aromatic acetylene with electron-donating and electron-withdrawing substituent can also readily produce the desire product (Scheme 75).
1,4-Disubstituted 5-iodo-1,2,3-triazole 95 is synthesized from azide, alkynes by trapping the carbanion intermediate with in situ generated electrophilic reagent (I+) from CuI and NBS through the click reaction. During the reaction CuI provides Cu+ and I-, Cu+ catalyzed the cycloaddition reaction whereas I- in the presence of NBS oxidized to I+ which then trapped the carbanion and attach to the 5-position of the triazole derivative (Scheme 76).134
One-pot multistep tandem click transformations135 to the synthesis of 1,2,3-triazole 96 from commercially available diazonium salts, terminal or trimethylsilyl-protected alkynes and sodium azide has also been investigated (Scheme 77).
Copper(I)-catalyzed 1,3-dipolar cycloaddition of azides and terminal alkynes106,107,136 is considered to be one of the most popular reaction for the synthesis of 1,4-disubstituted 1,2,3-triazoles. In situ preparation of aryl azides from aryl halide and subsequent use in 1,2,3-triazole formation minimises the risk related to handling as most of the azides are explosive in nature. Commercially available CuBr(PPh3)3 is used as a click catalytic system which does not require any additives to afford pure triazoles from terminal alkynes and an azide through in situ azide formation from their corresponding halides.137 Mihovilovic et al.138 reported a tandem catalysis protocol based on decarboxylative coupling of alkynoic acids and 1,3-dipolar cycloaddition of azides prepared in situ via three component reaction for the synthesis of a variety of functionalized 1,2,3-triazoles 97 in excellent yields. In situ preparation of low molecular weight alkynes from decarboxylation of alkanoic acid which are much easier to handle and use in the laboratory conditions makes the protocol more interesting (Scheme 78).
Aryl azides can be prepared from phenyl boronic acid and sodium azide and subsequently used for 1,3-dipolar cycloadition reaction using a recyclable heterogeneous clay supported CuO nanoparticles for one-pot synthesis of 1-aryl-1,2,3-triazoles. Sodium azide apart from its role as an azidonation reagent, also acts as a reducing agent producing in situ click-active Cu(I).139 Cu(I)-catalyzed azide alkyne cycloaddition (CuAAC) reaction for the synthesis of 1,2,3-triazoles is regioselective, and gives only the 1,4-disubstituted product although the heating between an azide and alkyne gives the regioisomer of 1,4- and 1,5-disubstituted 1,2,3-triazoles. Ruthenium based catalyst for azide alkyne cycloaddition reaction (RuAAC) is more selective to give only the 1,5-disubstituted tiazoles under conventional heating or microwave conditions.140 Ruthenium-catalyzed azide alkyne cycloaddition to afford 1,5-disubstituted 1,2,3-triazoles starting from an alkyl halide, an alkyne and sodium azide is reported.141 The organic azides were synthesized from in situ treatment of primary alkyl halide with sodium azide in DMA under microwave heating followed by subsequent addition if RuClCp(PPh3) and alkyne afforded the desired regioselctive 1,5-disubstituted triazoles in good yields. One-pot synthetic procedure directly from alkyl halide, sodium azide and alkyne is unsuccessful. This result may be due to the side reaction of ruthenium catalyst with sodium azide. This problem is easily overcome by using in situ synthesis of azide from alkyl halide and then subsequent addition of alkyne and ruthenium catalyst to afford desired triazoles 98 in very good conversion (Scheme 79).
Azide alkyne cycloaddition reaction using either copper catalyst or ruthenium catalyst afforded the triazoles which mainly limited to N-1 position. Reports on direct functionalization at N-2 position of triazoles are very few and suffers various disadvantages. Chen et al.142 developed a metal and base free three component reaction involving an ynones, sodium azide and an alkyl halide for the regioselective synthesis of 2,4,5-trisubstituted 1,2,3-triazoles 99 via azide chalcone oxidative cycloaddition and post-triazole arylation in one-pot (Scheme 80).
2.4 HETEROCYCLES CONTAINING FOUR HETEROATOMS
2.4.1 SYNTHESIS OF TETRAZOLES
Trimethyl orthoformate, amines and HN3 react to form tetrazoles 100 in good yields in the presence of indium under solvent free condition (Scheme 81).143
The enantioselective synthesis of 5-(1-hydroxy-alkyl)tetrazoles 101 by a [(salen)AlIIIMe]-catalyzed three component Passerini reaction of aldehydes, isocyanides and hydrazoic acid is described (Scheme 82).144
An isocyanide based four component reactions is investigated145 leading to the structurally diverse tetrazole derivative 102 as a major product along with a trace amount of amide as side product using In(III) Lewis acid catalyst from aldehyde, isocyanides, trimethylsilyl azide and aliphatic alcohol in refluxing conditions (Scheme 83).
3. CONSTRUCTION OF SIX-MEMBERED HETEROCYCLES
3.1 HETEROCYCLES CONTAINING ONE HETEROATOM
3.1.1 SYNTHESIS OF PYRIDINE AND DIHYDROPYRIDONE DERIVATIVES
One-pot synthesis of substituted pyridines 103 from aromatic aldehydes with ethyl acetoacetate and ammonium acetate via a domino cyclization-oxidative aromatization (dehydrogenation) approach has been reported. This process is based on the use of a new bifunctional Pd/C/K-10 montmorillonite and microwave irradiation (Scheme 84).146
Multicomponent synthesis of 2,4,6-triarylpyridines from acetophenone, aldehyde and ammonium acetate in the presence of bismuth triflate, heating under solvent free condition has been described.147 It has also been explored the catalytic activity of bismuth triflate towards unexpected acetalization of tetrazoloquinoline-4-carbaldehydes 104 (Scheme 85).
Mixing of methyl acetoacetate, 2 equivalents of aldehyde and 2 equivalents of aniline in the presence of indium trichloride (InCl3) provides highly substituted piperidine derivatives. This pseudo five component reaction is one-pot, atom and step economic and also diastereoselective. In the presence of Lewis acid first imine and enamine is generated which then undergoes Knoevenagel-like condensation with aldehyde to produce iminium-Knoevenagel intermediate which on subsequent elimination of proton and tautomerization forms a diene. This diene undergoes either aza-Diels-Alder or tandem Mannich-Michael type reation to afford piperidine derivative 105 (Scheme 86).148 The group which increases the nucleophilicity on aniline, increases the reaction rate and yield of the products whereas 2-substituted aldehyde, due to the steric effect, provides lower yield and takes longer reaction time. Similar substituted piperidine derivatives 105 is synthesized through multicomponent reaction using same components at room temperature in the presence of Bi(NO3)2.5H2O. This reaction is high yielding, atom economic, high diasteroselective and environmentally benign (Scheme 86).149 One-pot multicomponent reaction of aromatic aldehyde, amines, and acetoacetic esters in the presence of aqua-compatible ZrOCl2.8H2O has been developed for the synthesis of functionalized piperidine derivatives.150 Mechanistically, it is believed that enamine is produced by the reaction between acetoacetic ester and amine. This enamine may then react with aromatic aldehyde to generate a Knoevenagel-type product which undergoes tautomerization to form a non-isolable diene type metal complex. Another equivalent of amine and aldehyde provides imine which subsequently undergoes a [4+2] aza-Diels-Alder reaction with the diene metal complex to afford the functionalized piperidine 105 (Scheme 86).
The multicomponent reaction of alkenyl halides, silylimines and a secondary amine in the presence of a Pd(0) catalyst provides the trisubstituted pyridines 106 (Scheme 87).151 The Lewis acid-catalyzed aza-Diels-Alder cycloaddition reaction of 2-azadiene, formed by cross coupling of a silylimine with an alkenyl halide, and an enamine, formed by cross coupling of an alkenyl halide with an amine, followed by aromatization gave rise the pyridine derivatives.
Compared to base-catalyzed152 synthesis of 2-amino-3,5-dicarbonitrile-6-thiopyridines 107 using DABCO or triethylamine and conventional heating, the ZnCl2-catalyzed one-pot multicomponent reaction153 using conventional or microwave heating produced the desired product in better yields (Scheme 88). Recently a new convenient and eco-friendly multicomponent reaction of aldehydes, malononitrile and thiophenol is developed154 for the synthesis of biologically significant highly substituted pyridine derivatives 107 using efficient and reuseable Zn(II) and Cd(II) metal-organic frameworks (MOFs) as a catalyst (Scheme 88). Ultrasound irradiated multicomponent approaches to 2-amino-6-(arylthio)-4-arylpyridine-3,5-dicarbonitrile derivatives from aryl aldehydes, malononitrile and thiols using ZrOCl2.8H2O/NaNH2 as a catalyst in ionic liquid has been demonstrated. The mechanistic path depicted as initially a Knoevenagel adduct is formed by the reaction between malononitrile and aldehyde. Michael addition of this Knoevenagel adduct to a second molecule of malononitrile takes place, followed by an addition of thiolates to the nitrile groups of Michael addition product. Subsequent cyclization, aromatization and aerial oxidation afforded the corresponding pyridine derivatives 107 (Scheme 88).155
The use of yetterbium perfluorooctanoate for a straightforward and efficient synthesis of 2-amino-3-cyanopyridine derivatives 108 via one-pot multicomponent reaction of aldehydes, ketone, malononitrile and ammonium acetate has been achieved by Wang et al.156 They have investigated the positional effect of substituent of aldehydes and disclosed that the o-substituted aromatic aldehydes failed to produce any product while p- and m-substituted aldehyde smoothly provides the desire products. Electron-deficient aromatic aldehyde and various aromatic ketones are suitable for this transformation (Scheme 89).
Efficient one-pot multicomponent approaches to the synthesis of polysubstituted dihydropyridines from carbonyl compounds, malononitrile and acetonitrile using indium triflate has been described.157 It is also reported that the acetaldehyde did not afford the required product and aromatic aldehyde gave complex mixture which is not further investigated. The reaction seems to proceed via condensation-addition reaction leading to the formation of poly-substituted pyridine derivatives 109 in gram scale level (Scheme 90).
Ammonium acetate (NH4OAc) is widely used as an ammonia source in various organic transformation.158 Recently palladium-catalyzed microwave-assisted one-pot reaction protocol for the synthesis of isoquinilones 110 from 2-bromoarylaldehydes, terminal alkynes, and ammonium acetate has been described (Scheme 91).159
Cerium ammonium nitrate (CAN)-catalyzed convenient approach to 1,4-dihydropyridines 111 from 1-azadiene-based multicomponent reaction is developed.160 The 1-azadiene is generated in situ by the condensation of aromatic amines and α,β-unsaturated aldehydes and, in the same pot, α,β-dicarbonyl compound is added to provide the 1,4-dihydropyridines (Scheme 92). The use of aliphatic amines or α,β-unsaturated aldehydes except cinnamaldehyde derivatives produced complex reaction mixtures with the lower yield of the desired products.
Ytterbium triflate-catalyzed synthesis of dihydropyridines (DHP) through three component reaction is reported.161 The reaction of anilines, benzaldehyde and 3,3-diethoxypropinoate under refluxing condition in the presence of Yb(OTf)3 as catalyst affords the DHPs in good yields. The reaction is involved the followings steps: formation of imine and tautomerization of subsequent imine to enamine which further reacts with aldehyde to form enaminoalcohol. Dehydration of the enaminoalcohol affords α,β-unsaturated imine. Finally Michael type annulations and elimination of amine affords the desire product 112 (Scheme 93).
Microwave-assisted eco-friendly four component reaction of 3-amino-9-ethylcarbazole, malononitrile, aromatic aldehydes and acetylenic esters for the synthesis of N-carbozolyldihydropyridines 113 using indium trichloride as catalyst is developed.162 The transformation is believed to proceed via Knoevenagel condensation, Michael addition, followed by tautomerization leading to the formation of products (Scheme 94).
A simple and facile method for the synthesis of pyrazolo[3,4-b]pyridine and pyrazolo[1,5-a]pyrimidine with more structural diversities in the presence of KF-alumina at refluxing condition has been described (Scheme 95).163
In situ generated 2-azadienes based multicomponent reaction of 3-aminocoumarin, benzaldehyde, and various electron-rich alkenes in the presence of Yb(OTf)3 to afford 1,2,3,4-tetrahydropyrido[2,3-c]-coumarins 115 has been developed.164 Some of these products have been oxidized into the corresponding pyrido[2,3-c]coumarins 116 using various oxidants, such as Br2 and NaIO4 and nitrous gases (Scheme 96).
The reaction of diketene, N-tosylimines and aldehydes using TiCl4 as a Lewis acid catalyst for the synthesis of 2,6-disubstituted piperidin-4-ones 117 is reported (Scheme 97).165 The product is obtained as a mixture of diastereomers, which could be converted into a single diastereomer by treatment with K2CO3.
One-pot, three component reaction of nitroarenes, aldehydes and Danishefsky’s diene using In(0) as a reducing agent as well as a precatalyst for the in situ generation of In3+ for the access of dihydropyridin-4-ones is reported 118 (Scheme 98). 166 Mechanistically, In(0) in the presence of NH4Cl generates InCl3 as the reaction byproduct and catalyzed the aza-Diels-Alder cycloaddition reaction in a one-pot tandem sequence between the imine and then added diene to afford the desire product.
An enol ester (3,4-dihydro-6-methyl-2H-pyran-2-one) as the electron-rich alkene has been used to expand the synthetic versatility of the Povarov reaction for the synthesis of N-aryl lactam 119. Treatment of this enol ester with p-toluidine and ethyl glyoxalate under Sc(OTf)3 catalysis in MeCN at room temperature provided the N-aryl lactam 119 (25%) as the major product, instead of the Povarov adduct 119a (Scheme 99).167
3.1.2 SYNTHESIS OF TETRAHYDROQUINOLINE, QUINOLINE AND ISOQUINOLINE DERIVATIVES
A novel and practical one-pot multicomponent synthesis of isomeric ellipticine derivatives with high diastereoselectivity through intermolecular imino Diels-Alder reaction of 3-aminocarbazoles and benzaldehyde with 3,4-dihydro-2H-pyran in the presence of InCl3 as catalyst in ionic liquid is described (Scheme 100).168
One-pot three component Povarov reaction using inexpensive catalyst for the synthesis of diastereoselective furano[3,2-c]quinolines 122 and 123 have developed. Easily available starting material such as aldehyde, arylamine and dihydropyran or dihydrofuran in the presence of ferric sulphate refluxing in acetonitrile gives quinoline derivatives in good yields via the formation of aldimine followed by Diels-Alder reaction (Scheme 101).169 Recently furan and pyranoquinoline derivatives 122 and 123 were synthesized170 by Povarov multicomponent reaction using niobium pentachloride as an inexpensive and commercially available catalyst in room temperature. This reaction provides good yields and high diastereoselectivity with lower molar concentration of the catalyst (Scheme 101).
Unsaturated lactams with endo- or exo-cyclic double bonds undergo a multicomponent reaction with aldehydes and aniline derivatives, to afford structurally diverse products (Scheme 102) in the presence of Sc(OTf)3 as catalyst. A simple Povarov product 124 is obtained when R4=H, but due to steric effect final electrophilic substitution does not take place when lactam contains methyl group (R4 = Me), produced the Mannich-type product 125, in moderate yields, instead of the desired Povarov product from the reaction with p-toluidine or 4-methoxyaniline and ethyl glyoxalate, under the usual conditions. Interestingly, the adduct 126 in moderate yields is obtained when glyoxylic acid (R1 = CO2H) is used as the carbonyl component, which acts as a nucleophile and captures the final iminium ion intermediate. Under similar reaction conditions the spiro-Povarov adduct 127 is obtained in low yield (20%) when pyrrolidone derivative with an exo double bond is employed, (Scheme 102). Better yield is obtained when the reaction is carried out under microwave irradiation.171
Recently Povarov multicomponent reaction for the synthesis of tetrahydroquinoline and quinoline derivative is developed.172 Anhydrous acetonitrile solution of aldehyde, aniline and reactive electron rich oxa-, thia-, and imidazolones’ olefin component undergoes reaction in the presence of Sc(OTf)3 at room temperature with microwave irradiation to afford the tetrahydroquinoline adduct, which on oxidation with DDQ gave quinoline derivatives in good yields. The silent feature of this reaction is the high regioselectivity affording predominated cis-stereoisomer in all cases (Scheme 103).
Multicomponent reaction of aldehyde, naphthalen-2-amine and but-2-ynedioate in the presence of Yb(OTf)3 forms 3-arylbenzoquinoline-1,2-dicarboxylate derivatives 130 via imino-Diels-Alder reaction. Aliphatic aldehyde is not suitable for this methodology whereas both electron-donating and electron-withdrawing functionality in aromatic aldehydes are compatible for this reaction (Scheme 104).173
A high yielding, solvent free multicomponent synthesis of polyhydroquinoline derivatives 131 using nickel nanoparticles as a heterogeneous catalyst via Hantzsch protocol (Scheme 105) has recently been published.174 The catalytic efficacy of Pd-nanoparticle was investigated through one-pot multicomponent reaction of aromatic aldehydes, cyclic 1,3-dicarbonyl compounds, ethyl acetoacetate or ethyl cyanoacetate and ammonium acetate for the synthesis of polyhydroquinoline derivaties 131. The efficacy of Pd-nanoparticles in different solvents was also investigated and among them THF afforded excellent conversion (Scheme 105).175
An effort towards the synthesis of pyrimidine or pyrazole annulated heterocycles 132 via one-pot three component condensation reaction of aldehyde, aminouracil or aminopyrazole and 1,3-diketone refluxed in water using 20 % InCl3 as catalyst has been reported (Scheme 106).176
Some novel dihydropyridopyrimidine derivatives 133 have been synthesized via three component reaction177 of aryl aldehyde, N,N-dimethyl-6-aminouracil and cyanoacetylindol refluxing in ethanol using InCl3 as catalyst for 6 h. It is also observed that aromatic aldehyde having electron-withdrawing functional group affords high yield (Scheme 107).
Palladium-catalyzed, microwave-assisted, multicomponent reactions starting from o-bromoarylaldehydes, terminal alkynes, and aqueous ammonia for the synthesis of substituted isoquinoline derivatives 134 has been developed (Scheme 108).178
An efficient and high yielding procedure for the synthesis of pyrrolo[3,2-f]quinoline 135 and pyrrolo[2,3-h]quinoline derivatives 136 has been reported179 by one-pot multicomponent reaction of easily available starting material such as benzaldehydes, indoloamines, and phenylacetylenes in the presence of La(OTf)3 as catalyst in good yield by heating in ionic liquid. Among the two different pyrroloquinoline derivatives, pyrrolo[3,2-f]quinoline is formed as major product via normal path of [4+2] cycloaddition whereas pyrrolo[2,3-h]quinoline derivative is formed via an unexpected [3+2] cycloaddition pathway between phenylacetylene and azadiene (Scheme 109).
Straightforward and efficient three component reaction is demonstrated180 for the synthesis of quinolines 137 using environment friendly and reusable yetterbium triflate as catalyst from easily available starting material such as aldehyde, alkynes and amines under microwave irradiation in an ionic liquid as green solvent (Scheme 110).
1,2-Dihydroisoquinolines are of interest because of their frequent occurrence in nature and their wide range of biological and physiological effects.181,182 The search for better hits encouraged to construct structural novel 1,2-dihydroisoquinoline library for the subsequent biological assays. Tandem or cascade reactions and multicomponent reactions offer significant advantages over traditional approaches for the rapid construction of several bonds in a single synthetic procedure. Recently Rh2(OAc)4 and AgOTf catalyzed tandem cyclization/three component reaction of 2-alkynylarylaldimines, diazo compounds, and water or alcohols to afford functionalized 1,2-dihydroisoquinolines 138 is reported (Scheme 111).183
Silver(I)-catalyzed intramolecular cyclization and Cu(II)-catalyzed oxidation of an aliphatic C-H bond of tertiary amine leading to isoquinoline derivatives has been reported by Wu et al.184 Reaction of 2-alkynylbenzaldehyde, sulfohydrazide and a tertiary amine in the presence of Cu(II) and Ag(I) salts afforded an unexpected H-pyrazolo[5,1-a]isoquinolines 139 in good yields (Scheme 112).
C-H Bond activation and annulation is one of the most efficient methodologies for the formation of isoquinoline derivatives. Miura et al.185 has reported an efficient method for the formation of polysubstituted isoquinoline derivatives based on rhodium-based C-H activation of benzophenone imines with alkynes. Recently Chen et al.186 has reported RhCp*Cl2-catalyzed three component reaction of aryl halides, methylamine and alkyne through C-H bond activation for the regioselective formation of isoquinolinium salt 140 (Scheme 113).
The same strategy of C-H bond activation and annulation reaction for the formation of isoquinolinium salts has been described by the same group187 using less expensive ruthenium-based catalyst as compared to the rhodium one. Copper(I)-catalyzed electrophillic tandem cyclization of 2-ethynylbenzaldehyde with o-benzenediamines and iodine for the formation of iodoisoquinoline fused benzamidazoles 141 is reported by Ouyang et al. (Scheme 114).188
Iodoisoqinoline smoothly undergoes the Suzuki (142), Sonogashira (143) and Heck (144) coupling reactions, respectively, in excellent yields (Scheme 115).
Pd, Cu, and Ag salts have been demonstrated effectively for activation of triple bond which provides an efficient route for the synthesis of various heterocyclic systems.189 A parallel diversity-oriented synthesis of 1-(1H-imidazol-1-yl)-1,2-dihydroisoquinoline derivatives 145 via AgOTf-catalyzed three component reaction of 2-alkynylbenzaldehyde, amine, and imidazole has been reported (Scheme 116).190
Pd/C-catalyzed multicomponent reaction of 1,2-dichloroquinoxaline, hydrazine, phenylacetylene and a variety of aldehydes for the construction of pyrrole ring is reported recently. In situ formation Pd(0) and in the presence of Cu(I) catalyst catalyzes the Sonogashira coupling reaction and finally triple bond activation followed by intramolecular cyclization to afford the pyrrolo[2,3-b]quinozalines 146 (Scheme 117).191
Hua et al. has described192 an one-pot three component reaction for the synthesis of isoquinolines and fused pyridine heterocycles from aryl ketones, hydroxylamine, and alkynes catalyzed by rhodium. The reaction involves condensation of aryl ketones and hydroxylamine, C−H bond activation of the in situ generated aryl ketone oximes catalyzed by Rh(III), and cyclization with internal alkynes which finally afford the isoquinolines and fused pyridines 147 in excellent yields (Scheme 118).
Three component reactions of nitroarenes, aldehyde and phenylacetylene for the synthesis of quinoline derivatives 148 by using indium metal in the presence of diluted hydrochloric acid has been demonstrated.193 The reaction procedure involves reduction of the nitroarenes to aniline, coupling with aniline, aldehyde and terminal alkynes, cyclization and dehydrogenation leading to the formation of the product in good yield (Scheme 119).
The Cu(I) iodide-catalyzed multicomponent reaction of 2-ethynylbenzaldehyde, paraformaldehyde, diisopropylamine and t-butylamine affords isoquinoline derivative 149 (Scheme 120).194
A new multicomponent reaction of arynes with isocyanides and terminal alkynes to afford pyridine or isoquinoline derivatives in good to high yields, depending upon the reaction conditions which include the ratio of the reagent and solvents, has been developed.195 It is interesting to note that this one-pot multicomponent regioselective synthesis of polysubstituted pyridines 150 and isoquinolines 151 is difficult to achieve through conventional methods (Scheme 121).
Silver triflate and triphenylphosphine cocatalyzed reaction of 2-alkynylbenzaldehydes, amines and α,β-unsaturated ketones for the synthesis of functionalised 1,2-dihydroisoquinolines 152 in moderate to good yields has been reported (Scheme 122).196 According to the proposed mechanism, the triple bond co-ordinates to the silver salt and, subsequently, the nitrogen atom of the imine attacks the activated triple bond via 6-endo cyclization to afford an isoquinolinium intermediate. In the meantime, triphenylphosphine attacks the α,β-unsaturated ketone as a nucleophilic catalyst, affords enolate, which then undergoes intermolecular attack of the isoquinolinium intermediate to generate the phosphinium species. Finally, elimination of phosphine gives the desired product.
One-pot three component reaction of 2-alkynylaryl aldehyde, primary amines and terminal alkynes for the preparation of dihydroisoquinoline derivative 153 using pybox as a ligand and copper(I) as a catalyst via tandem addition/cyclization mechanism has been reported (Scheme 123).197
An efficient route to the synthesis of 1,2-dihydroisoquinolin-1-ylphosphonate 154 is described. This tandem multicomponent reaction of 2-bromobenzaldehyde, alkyne, amine and diethyl phosphite in the presence of palladium and copper as combine catalyst affords the above mentioned product. This reaction is believed to proceed via Sonogashira-intramolecular cyclization and nucleophilic addition reaction (Scheme 124).198
3.1.3 SYNTHESIS OF QUINOLONE AND ISOQUINOLONE DERIVATIVES
The four component mixture of aldehyde, diethyl malonate, dimedone and ammonium acetate on refluxing in water in the presence of ZnO as catalyst afforded octahydroquinolinediones 155 in good yields. This methodology is eco-friendly, simple, efficient and mild one (Scheme 125).199
The selective synthesis of 2-phenyl-4-quinolone 156 in good yield by the use of heterogeneous catalysts associating the [Pd(PNP)]@SBA-15 catalyst to a grafted amine catalyst as [NH2(CH2)3]@SBA-3 in a mechanical mixture from o-iodoaniline, phenylacetylene and in the presence of carbon monoxide is reported (Scheme 126).200 It is interesting to note that such an approach decreases the palladium contamination in the final products found only 3 to 5 ppm of palladium compared to using homogenous catalytic system, where 40 ppm of palladium is found with final product.
An approach to cyclocarbonylation is realized through the palladium-catalyzed one-pot reaction of diethyl (2-iodophenyl)malonates and imidoyl chlorides using palladium(II) acetate, tri(2,6-dimethoxyphenyl)phosphine (TDMPP) and diisopropylethylamine in tetrahydrofuran under carbon monoxide (400 psi) to provide the highly substituted isoquinolin-1(2H)-ones 157 (Scheme 127).201
A new strategy for the syntheses of skeletal diverse N-fused polycyclic compounds 158 via an Ugi-type MCR (Groebke−Blackburn−Bienayme reaction) followed by a CuI-catalyzed coupling reaction for intramolecular C-N bond formation to form 159 or tandem Pictet-Spengler reaction to afford 160 respectively is described recently (Scheme 128).202
Michael addition and subsequent carbonylation protocol can be used for the synthesis of quinoline derivatives. In this context, palladium-catalyzed intermolecular cyclocarbonylation of 2-iodoanilines with diethyl ethoxycarbonylbutendienoate for the synthesis of 2,3,3-triethoxycarbonyl-2,3-dihydro-4(1H)-quinolinone derivatives 161 has been decribed (Scheme 129).203
Recently 4-ethoxycarbonyl-3,4-dihydroisoquinolinone derivatives 162 are synthesized by palladium-catalyzed three component intermolecular cyclocarbonylation reaction of diethyl (2-iodoaryl)acetates, N-tosylimines and carbon monoxide by the same group.204 This reaction includes the Mannich addition, cyclocarbonylation and decarboxylation (Scheme 130).
3.1.4 SYNTHESIS OF PYRAN AND LACTONE DERIVATIVES
Exploitation of the catalytic efficacy of Mg/Al hydrocalcite has enabled the microwave, solvent free synthesis of 2-aminochromenes 163 by utilizing the components, 1-naphthol, aromatic aldehydes and malononitrile as outlined in Scheme 131.205
GaCl3-catalyzed three component reaction for the synthesis of 1,3-disubstituted benzochromene 164 is reported. This new approach for the preparation of benzochromene from 2-naphthol, alkyne and aldehyde mechanistically proceeds either via the formation of vinyl naphthalen-2-ol from arylation of alkyne to 2-naphthol and subsequent cyclization of aldehyde with vinyl naphthalene providing the product or via formation of propargyl alcohol and propargyl ether from aldehyde and alkyne which then undergo Claisen rearrangement and subsequent cyclization resulting the product (Scheme 132).206
Solvent free three component reaction of kojic acid, aldehyde and 1,3-dione affording dihydropyrano[3,2-b]chromenedione derivative 165 using InCl3 has been achieved. Domino Knoevenagel and hetero Diels-Alder reaction and subsequent dehydration lead to the products (Scheme 133).207
An efficient, mild reaction for the synthesis of highly substituted pyran derivatives 166 is investigated in one-pot multicomponent condensation of an aldehyde, malononitrile, and diketoesters with recyclable heterogeneous strong basic Mg/La catalyst (Scheme 134).208
An efficient and green methodology has been investigated209 for the construction of pyran derivatives 167 through one-pot three component coupling reaction using nanostructure ZnO catalyst of an aldehyde, malononitile and 1,3-diketone. It has also been developed a rearrangement reaction for the conversion of pyran derivatives to the 2-pyridone in aqueous media applying p-TsOH as catalyst (Scheme 135).
Synthesis of nanocrystalline MgO and its catalytic efficacy and recyclability of the catalyst have been examined through the multicomponent synthesis of isatin, malononitrile, 1,3-diketone in equimolar ratio refluxing in water afforded spirooxindoles with fused tetrahydrochromene 168 in good yields (Scheme 136).210
One-pot three component reaction of isatin, malononitrile or cyanoacetic acid ester and phthalhydrazide catalyzed by NiCl2 in an eco-friendly and cost effective solvent PEG 600 afforded pyrazolophthalazinyl spirooxindoles 169 in high to excellent yields has been described (Scheme 137).211
One-pot four component coupling of aromatic amine, aldehyde, β-naphthol, β-oxodithioester and primary alcohol for the synthesis of 4H-[f]chromones 170 in the presence of InCl3 has recently been reported by Singh et al.212 This four component coupling reaction proceeds via Knoevenagel condensation, Michael addition, intramolecular cyclohydration and trans-esterification in a highly chemoselective and regioselctive manner affording the benzo[f]chromones in good yields (Scheme 138).
The synthesis of 2-aminochromene 171 and indolyl chromene 172 by indium trichloride via three component reaction is developed. Salicyldehyde, malononitrile and Hantzsch dihydropyridine ester in the presence of InCl3 produced 2-aminochromene. Hantzsch dihydropyridine ester here acts as hydride donor in the formation of 2-aminochromene. Interestingly under the same condition replacing Hantzsch dihydropyridine ester with indole provides indolyl chromene derivatives (Scheme 139).213
An efficient three component coupling reaction involving Knoevenagel condensation, Micheal addition and then intramolecular cyclization in the presence of nano ZnO affording dihydropyranochromene 173 from aromatic aldehyde, malononitrile and 3-hydroxycoumarin has been demonstrated (Scheme 140).214
An efficient, solvent free, eco-friendly, one-pot multicomponent reaction methodology for the synthesis of xanthene and anthracene derivatives 174 and 175 respectively in excellent yields by the reaction of aldehydes, β-naphthol and cyclic 1,3-dicarbonyl compounds in the presence of catalytic amount of InCl3 or P2O5 is reported by Singh et al. (Scheme 141).215 Other research groups also have synthesized benzoxanthene derivatives 174 in excellent yields by an one-pot three component condensation216 reaction of dimedone, aldehyde and β-naphthol using metal nanoparticle supported acidic ionic liquid. Combination of F3O4-nano-support feature and soft imidazolium linkers makes the catalyst more free and soluble to the reaction system which facilitates effective condensation (Scheme 141).
Singh et al.217 developed an efficient route for the synthesis of chromeno[2,3-d]pyrimidinone 176 and diazabenzo[b]fluorenone 177 using indium trichloride under solvent free condition. Mixture of aromatic aldehyde, cyclic-1,3-diketone and 1,3-dimethylbarbutaric acid in the presence of indium catalyst under neat condition resulting the formation of product. Aliphatic aldehydes, due to its tendency to undergo aldol condensation reaction as a side reaction affords mixtures of products lowering the yields of major product (Scheme 142).
Coupling of aldehyde, homoallylic alcohols and ammonium thiocyanates under three component reaction in the presence of In(OTf)3 produced 4-thiocyanotetrahydropyranes 178 via Prins-cyclization and thiocyanation sequence (Scheme 143).218
An efficient three component strategy involves the generation of allyltin(IV) from allyl bromide in the presence of Pd(0)/SnX2 (X = Cl, Br) in anhydrous DCM, then homoallyloxytin(IV) intermediate is formed from allyltin(IV) and an aldehyde, and finally coupled with an aldehyde, an aryl epoxide or an arene to afford tetrahydropyrans 179, benzyl tetrahydropyrans 180 or 4,4-diarylbut-1-enes 181, respectively (Scheme 144).219
The three component reaction between 1,3-diketone with terminal alkyne in the presence of carbon monoxide (200 psi) is effected in the presence of Pd(PPh3)2Cl2 as catalyst and dppp as ligand in ionic liquid [bmim][Tf2N] to give the highly substituted endocyclic enol lactone 182 in moderate to good yield (Scheme 145).220
Chromenes are the structural motifs frequently found in a number of natural products and biologically active molecules. An enantioselective one-pot three component reaction of salicylaldehyde, malononitrile, and indole for the synthesis of 2-amino-4-(indol-3-yl)-4H-chromenes 183 through a Knoevenagel/Pinner/Friedel-Crafts reaction sequence is catalyzed by Zn(II) complex (Scheme 146).221
Multicomponent reaction of 3,5-dimethoxyphenol, diethyl malonate and various aromatic aldehyde under room temperature in the presence of niobium pentachloride as catalyst providing coumarin derivatives 184 in good yields has been demonstrated.222 Knoevenagal condensation, hydroarylation and intramolecular lactonization is responsible for the formation the product (Scheme 147).
Copper-catalyzed multicomponent reaction223 of sugar-derived alkyne, tosyl azide and salicylaldehyde for the synthesis of glycosyl iminocoumarins 185 framework has been explored. This reaction undergoes initially copper-catalyzed click reaction between sugar-derived terminal alkyne and tosyl azide. Elimination of nitrogen (N2) of the intermediate forms ketemine, which on addition with phenolate of salicylaldehyde and subsequent intramolecular cyclization leads to the formation of products (Scheme 148).
Recently, a consecutive one-pot, three component coupling-addition-SNAr (CASNAR) sequence starting from aroyl chlorides, alkynes, and sodium sulfide nonahydrate has been developed for the synthesis of 4H-thiochromen-4-one 186 in good yields (Scheme 149).224
3.2 HETEROCYCLES CONTAINING TWO HETEROATOMS
3.2.1 SYNTHESIS OF PYRIMIDINE AND DIHYDROPYRIMIDONE DERIVATIVES
A copper-catalyzed multicomponent reaction of sulfonyl azides, terminal alkynes, and α,β-unsaturated imines has been developed to give a new class of pyrimidine derivative, N-sulfonyl-2-alkylidene-1,2,3,4-tetrahydropyrimidines 187 (Scheme 150).225 In most of the cases, pyrimidines is isolated as the major product and in several cases, azetidin-2-imines 188 is found as minor product with high trans selectivity from this three component reaction.
ZnCl2-catalyzed three component reaction from structurally diverse enamines, triethyl orthoformate, and ammonium acetate leads to 4,5-disubstituted pyrimidine derivatives 189 in good yields through a single operation (Scheme 151).226
AgI-catalyzed synthesis of polysubstituted pyrimidines 190 with high regioselectivity in good to excellent yields is achieved from electron-deficient alkynes, anilines, and formaldehyde (Scheme 152).227
Indium triflate-catalyzed228 three component condensation reactions for the synthesis of spiro hexahydropyrimidine derivatives 191 from easily available starting materials like cyclohexanone, formaldehyde and aniline stirring in dichloromethane in room temperature has been described (Scheme 153).
Palladium-catalyzed iminoacylation by isocyanide insertion is an efficient but relatively unexplored method that offers significant advantages as compared to the well-known carbon monoxide insertions. By employing iminoacylation in palladium-catalyzed cascade reactions,229 Orru et al. recently describes230 a multicomponent reaction approach toward the synthesis of 4-aminophthalazin-1(2H)-ones 192 starting from o-bromobenzoates, isocyanides, and hydrazines in excellent yields (Scheme 154).
The multicomponent reaction of aniline, formaldehyde and 1,3-diketone provides hexahydropyrimidines 194 using ferric chloride as catalyst in dichloromethane has been described.231 The same methodology provides spiro analogous 193 when indane is used as 1,3-diketone. Similarly spiro substituted piperidine 195 were obtained instead of hexahydropyrimidines when dimedone is used as 1,3-dicarbonyl compound, due to its high reactivity (Scheme 155).
Highly diverse multicomponent tandem reaction of acid chloride, terminal alkyne and ethyl-2-amino-1H-indole-3-carboxylate for the synthesis of pyrimidoindoles 196 in good to excellent yields has been reported.232 The reaction involves sequential Sonograshira and [3+3] cyclocondensation reaction leading to the formation of product (Scheme 156).
Recently Biginelli multicomponent reaction for the synthesis of dihydropyrimidones 197 in excellent yield from ethyl acetoacetate, aldehyde and urea as component in the presence of copper triflate,233 bismuth nitrate234 and LaCl3235 as catalyst under microwave irradiation has been reported by different research groups. An efforts towards the synthesis of the same Biginelli dihydropyrimidine 197 from the same components using Zn(II) and Cd(II) metal-organic frameworks (MOFs) as a heterogeneous catalyst under solvent free condition has also been reported.236 The metal-organic framework (MOFs) is useful, reusable and green catalyst making shorter reaction times for this synthesis (Scheme 157).
Recently SnCl2-catalyzed cyclocondensation of β-oxodithioesters with a variety of readily accessible aldehydes and urea (Scheme 158) has been reported for the synthesis of new dihydropyrimidinones 198 through237 three-component Biginelli reaction.
Synthesis of polyfunctional 2,3-dihydropyrimidin-4-ones 199 utilizing ZnCl2 as catalyst has been described.238 The Lewis acid ZnCl2 plays a dual role: promotes the isomerizaton of hydroaminated-intermediate and also facilitates further amidation of the isomerized product (Scheme 159).
The synthesis of 5:6 and 6:6 fused pyrimidine derivatives catalyzed by KF/alumina through a three component reaction has been reported in good yields (Scheme 160).239
A diastereoselective three component reaction using antimony trichloride for the synthesis of arylhexahydrofuropyrimidin-2-one 203 has been developed. Easily available starting material aldehyde, urea and dihydropyrane or dihydrofuran in the presence of mild and superior antimony trichloride as catalyst leads to the pyrimidine scaffolds in excellent yields (Scheme 161).240
3.2.2 SYNTHESIS OF QUINAZOLINONE, QUINOXALINE AND PROMAZINE DERIVATIVES
4(3H)-Quinazolinones have emerged as an important class of heterocycles that have attracted significant synthetic interest because of their pharmacological and therapeutic properties such as antibacterial, antifungal, antimalarial antihypertensive, anticonvulsant, analgesic, anti-inflammatory antiviral and anticancer activities.241-242 3-Aryl-6,8-dichloro-2H-1,3-benzoxaine-2,4(3H)-diones and 3-arylquinazoline-2,4(1H,3H)-diones have been used as antimycobacterial agents.243 Several methods have been developed for their synthesis.244
Palladium-catalyzed three component intermolecular cyclocarbonylation of 2-iodoanilines and N-toluenesulfonyl-aldimines in the presence of carbon monoxide providing dihydroquinazolinenone derivatives 204 has been investigated by Alper et al.245 They also examined the effect of different ligands as well as solvent effects for this reaction and found that dppf as ligand and THF or toluene as solvent are superior in this regards (Scheme 162).
Another palladium-catalyzed cyclocarbonylation of o-iodoanilines with imidoyl chlorides and carbon monoxide for the synthesis of substituted quinazolin-4(3H)-ones 205 has been developed in good to excellent yields by the same group. When equimolar amounts of o-iodoanilines and imidoyl chlorides are treated with palladium(II) acetate, triphenylphosphine and triethylamine in tetrahydrofuran under carbon monoxide (500 psi) at 140 °C, the corresponding quinazolinone derivatives are formed (Scheme 163).246 The reaction is believed to proceed via in situ formation of an amidine, followed by oxidative addition, carbon monoxide insertion, and intramolecular cyclization to give the substituted quinazolin-4(3H)-ones.
The selective synthesis of 2,3-dihydroquinazolin-4(1H)-ones 206 and quinazolin-4(3H)-ones 207 using Ga(OTf)3 as a reusable catalyst in ethanol involving isatoic anhydride, ammonium acetate or amines and aldehydes in one-pot have been described (Scheme 164).247
Palladium acetate and cuprous iodide assisted sequential multicomponent cascade synthesis of quinazolinones 208 in moderate to good yields from tosyl azide, 2-iodoaniline, phenylacetylene and carbon monoxide is reported.248 This sequential process includes the copper-catalyzed three component reaction and palladium-catalyzed carbonylation (Scheme 165).
An efficient and convenient synthesis for the preparation of 1′H-spiro[indoline-3,2ˊ-quinazoline]-2,4′(3′H)-diones 209 through a three component cyclocondensation employing isatoic anhydride, isatins and amines using the inexpensive, non-toxic and easily available KAl(SO4)2·12H2O (alum) as catalyst has been described (Scheme 166).249
The synthesis of N-cyclohexyl-3-arylquinoxaline-2-amines 210 through the reaction of o-phenylenediamine, cyclohexyl isocyanide and an aromatic aldehyde utilizing ferric perchlorate is reported (Scheme 167).250
Three component reaction using 2-(methylamino)phenyl-4-methylbenzenesulfonate, paraformaldehyde and phenylacetylene in the presence of CuCl and KOH provides 1,4-benzoxazine derivatives 211. The process is completely regioselective and stereoselective resulting (Z)-isomer (Scheme 168).251
Palladium-catalyzed under microwave irradiation three component approach to promazine 212 with the formation of C-S bond from thiophenols and aryl iodides and C-N bond from amines and aryl bromides in an one-pot reaction has been reported.252 The transformation may also be carried out by conventional heating with comparable yields (Scheme 169).
Smiles rearrangement under microwave irradiation has been investigated253 for one-pot synthesis of benzo[b][1,4]thiazin-3(4H)-one derivatives 213 involving 2-chlorobenzothiols, chloroacetyl chloride and primary amines as components (Scheme 170).
3.3 HETEROCYCLES CONTAINING THREE HETEROATOMS
One-pot multicomponent synthesis of triazinane diones 214 from phosphonates, nitriles, aldehydes, and isocyanates have been reported (Scheme 171). Aldehydes with (hetero) aromatic and aliphatic substituents and isocyanate components with benzylic and aromatic substituents is suitable for the reaction.254
Synthesis of 2-aryl-1,3,2-aryldioxaborins 215 from phenols, aldehydes, and phenylboronic acid using ZrCl4 as catalyst is investigated (Scheme 172).255 This reaction is assumed to proceed via [3,3]-sigmatropic rearrangement pathway which is well documented in the literature.256
4. CONSTRUCTION OF SEVEN-MEMBERED HETEROCYCLES
Synthesis of various furoazepine derivatives from imidazopyridine caebenes, different heterocyclic o-dialdehydes and activated alkynes is developed.257 The formation of products undergoes the followings steps: first imidazopyridine carbenes and aromatic dialdehyde form dipolar intermediates via nucleophilic addition which undergo [3+2] cycloaddition with electron-deficient alkynes followed by aromatization and further intramolecular nucleophilic addition to aldehyde give the products in good yields (Scheme 173).
Diazepines258 have been the object of intense investigation in medicinal chemistry, because of their remarkable central nervous system depressant activity and more recently, the area of biological interest of 1,5-benzodiazepines have been extended to antibiotics and various diseases.259 Recently one-pot multicomponent synthesis of diazepine derivatives 218 from a 1,2-diamine, a linear or cyclic ketone, and an isocyanide in the presence of a catalytic amount of silica supported iron oxide (Fe3O4/SiO2) nanoparticles at ambient temperature has been reported.260 Metal nanoparticles (MNPs), especially supported magnetic metal nanoparticles (S-MMNPs), have emerged as a new class of nanocatalyst (Scheme 174).
o-Phenylenediamines, with in situ generated alkynones from acyl chloride and alkynes produced pharmacologically interesting seven-membered heterocycles 1,5-benzodiazepines 219 via coupling addition-cyclocondensation sequence in the presence of palladium and copper as combined catalyst (Scheme 175).261
One-pot synthesis of benzodiazepinone derivatives 220 by Er(III) triflate is reported.262 Condensation reaction of 1,2-phenylenediamine and dimedone followed by cyclization with aliphatic or aromatic acyl chloride in the same reaction pot stirring at room temperature afforded the benzodiazepinone derivative in good yields (Scheme 176).
A novel multicomponent tandem imino-pinacol coupling-aza-Michael reaction have developed263 for the sysnthesis of polysubstituted monocyclic 1,4-diazepine derivatives 221 from α,β-unsaturated ketone, aromatic aldehyde and aniline in the presence of Zn/InCl3 as catalyst under aqueous medium. The method is eco-compatible, efficient, high yielding and atom economical (Scheme 177).
An isocyanide-based multicomponent reaction for the synthesis of polysubstituted 1,4-benzodiazepin-3-ones 222 and 223 (Scheme 178)264 using a reducing agent, Fe0/NH4Cl, in protic solvents, under controlled microwave irradiation, proceeds through an one-pot, two-step process. Interestingly, o-nitrobenzaldehyde and o-nitrobenzylamine not only act as bifunctional substrates, but also selectively direct a substitution pattern for benzodiazepin-3-ones of type 222 and 223.
Microwave-assisted approach for the formation of pyrimido-oxazepine analoges 224 is developed. Mixture of dichloropyrimidine, secondary or primary aliphatic amine and salicylaldehyde in the presence of cesium carbonate (Cs2CO3) under microwave irradiation followed by reduction of the subsequent product with NaBH4 at room temperature in the same reaction pot provides the expected product (Scheme 179). It is interesting that arylamine produces lower yield under this study.265
5. MISCELLANEOUS
Solvent free multicomponent reaction catalyzed by copper salt towards the synthesis of N-sulfonylazetidine-2-imine 225 is described.266 Multicomponent reaction of sulfonyl azides, terminal alkyne and carbodiimides in the presence of copper oxide leads to the formation of the product 225 (Scheme 180).
A new and simple synthetic protocol for the construction of spirocyclic quinoline derivatives 226 through one-pot three component coupling reaction of an alkynol derivative, an aldehyde, and an aromatic amine has been developed (Scheme 181).267 In this Povarov synthesis, both reactive species, enol ether and the N-arylaldimine, are catalytically preformed in situ prior to the reaction.
A multicomponent reaction is carried out by the treatment of pent-4-yn-1-ols, salicylaldehydes and alkoxymethanes by [Pd(MeCN)4](BF4)2 in acetonitrile at room temperature to provide the oxygen-substituted chroman spiroacetal 227 in high yields (Scheme 182).268
Copper-catalyzed domino three component coupling reaction under microwave irradiation for the synthesis of indole-fused benzo-1,4-diazepines 228 from N-mesyl-2-ethynylaniline and o-bromobenzylamines has been reported by Ohta et al. (Scheme 183).269
Copper-catalyzed270 domino three component coupling-cyclization is developed by Ohta group involving ethynylaniline, paraformaldehyde, an amino ester in dioxane under microwave irradiation to produce the indole intermediate which on treatment with MsOH at 80 °C for 30 min furnished 4-oxotetrahydro-β-carbolines 229 (Scheme 184).
A new copper-catalyzed synthesis of 3-(aminomethyl)isoquinoline-fused polycyclic compounds 230 through four component coupling, cyclization, and oxidation, is described by the same group (Scheme 185).271 The Mannich-type reaction of 2-ethynylbenzaldehyde with paraformaldehyde and a secondary amine followed by the treatment with a diamine component in the presence of CuCl afforded tricyclic isoquinolines. In this reaction, CuCl showed effective catalytic activity under an oxygen atmosphere to afford the corresponding products in moderate to good yields.
Palladium-catalyzed couplings of N,N-di(o-iodophenyl)carbodiimides with different alkyl or aryl amines under carbon monoxide providing quinazolino[3,2-a]quinazolinones 231, in a single operation has been reported by Alper et al.272 The reaction is not dependent on the electronic nature of the substituents of the aryl moiety. Increasing the bulkiness of the R3 group decreased the yield of the tetracyclic product (Scheme 186).
An easy route to benzoindolizine derivatives 232 involving the cycloaddition of azadienes with a silyl enol ether followed by a palladium(0)-catalyzed Heck coupling reaction has been developed. The in situ generated azadienes (X = H, Me, Cl) undergoes cycloaddition reactions with ethyl 3-trimethylsiloxy-2-butenoate followed by intramolecular Heck coupling reaction using a catalytic amount of palladium(II) acetate in anhydrous toluene in the presence of a slight excess of triethylamine as a base (Scheme 187).273
An efficient, simple and novel one-pot four component reaction for the synthesis274 of pyranopyrazol-6-one derivatives 233 refluxing in aqueous media in the presence of Ba(OH)2 as readily available, inexpensive and effective catalyst from hydrazine hydrate, ethyl acetoacetate, aromatic aldehyde and meldrums acid has been reported (Scheme 188).
Multicomponent reaction of ethyl acetoacetate, hydrazine hydrate, malononitrile and aldehyde to produce 6-amino-4-alkyl/aryl-3-methyl-2,4-dihydropyrano[2,3-c]pyrazole-5-carbonitrile 234 using expedient and recyclable γ-alumina as catalyst is described recently.275 It is also reported the comparative catalytic efficacy of γ-alumina, basic alumina and KF-alumina. Among these three catalysts, γ-alumina proved to be the most effective for this multicomponent reaction (Scheme 189).
Silver(I) triflate and nickel perchlorate hexahydrate-catalyzed three component reactions for the synthesis of tetrahydropyridazinoisoquinoline derivative 235 of 2-alkynylbenzaldehydes, sulfonohydrazide and dimethyl cyclopropane has been reported where 6-endo cyclization and [3+3] cycloaddition is responsible for the formation of product (Scheme 190).276
A novel four component reactions for the construction of tetracyclic aza-analogs of nuevamine 236 from aldehyde, amine, isonitrile compound and maleic anhydride by free radical cyclization with tributyltin hydride and scandium triflate under microwave irradiation is reported (Scheme 191).277
An array of naphthoxazinone derivatives 237 is synthesized with copper nanoparticle from the mixture of aldehyde, urea and 2-naphthol in PEG-400 at room temperature.278 In case of N-methylurea, the yield of the product reduces whereas in case of N,N-dimethylurea, both α-naphthol and naphthalen-2-thiol are failed to produce any product. In mechanistic rationalization, at initial event aldehyde and urea condensed to form reactive acylimine. Subsequently, the acylimine undergoes a cyclization with 2-naphthol to afford naphthoxazinones (Scheme 192).
Environmentally benign one-pot four component condensation reaction of phthalic anhydride, hydrazinium hydroxide, aromatic aldehyde and demidone in the presence of Ce(SO4)2.4H2O as eco-friendly catalyst for the formation of indazolophthalazine trione 238 under solvent free condition has been reported. The mechanistic rationalization of this multicomponent reaction is responsible for initially formation of phthalhydrazide from nucleophilic addition of hydrazinium hydroxide to phthalic anhydride followed by dehydration and subsequent Michael type addition reaction and cyclization of phthalhydrazide and heterodiene which is formed by Knoevenagel condensation between dimedone and aldehyde affording the product (Scheme 193).279
One-pot four component reaction280 of phthalimide, hydrazine hydrate, aromatic aldehyde and malononitrile or ethyl cyanoacetate in refluxing ethanol has been described using NiCl2.6H2O as effective and inexpensive catalyst for the synthesis pyrazolophthalazinedione 239 in good yield (Scheme 194).
Three component reaction of δ-hydroxy-α,β-unsaturated aldehyde (perlin aldehyde), 1,3-diketone and aniline in dichloromethane using indium(III) chloride as catalyst at -10 °C is described.281 This provides the sugar annulated N-aryl-tetrahydropyridine derivatives 240 in good yield (Scheme 195).
Transition metal-catalyzed reactions of functionalized allenes have become one of the most important tools for the synthesis of some biologically important systems especially the medium-sized heterocyclic system. Palladium-catalyzed carbopalladation of allenes may afford the π-allyllic palladium species which would react with a nucleophile to form the allylation product. A three component Pd(0)-catalyzed highly chemo- and regioselective cyclization reaction for the synthesis of ten-memebered heterocycles 241 with two stereodefined C-C bonds from 1,5-bisallenes, organic halides and primary amines has been reported (Scheme 196).282
One-pot three component reaction combining a set of acyclic substrates/reagents of 1,2-diaza 1,3-dienes, primary amines, and isothiocyanates in a different order of addition under the same reaction conditions, a robust chemo-/regioselective approach to heterocyclic skeletal diversity is achieved. The protocols afford selectively substituted 1,3,5-trisubstituted 2-thiohydantoins or 2-iminothiazolidin-4-ones 242 and 243 bearing a diversity at the 5-position of the heterorings (Scheme 197).283
Salicylaldehyde, malononitrile and amine in the presence of catalytic amount of LiClO4 afford benzopyranopyrimidines 244 via pseudo four component reactions. It is assumed that the reaction involved the Knoevenagel condensation between salicylaldehyde and malononitrile followed by subsequent Pinner reaction to afford an intermediate. Cyano group of that intermediate is then attacked by the amine, followed by cyclization with another mole of salicylaldehyde producing the final product (Scheme 198).284
6. CONCLUSION
Heterocycles are found in many natural products, pharmaceuticals, organic materials, and in numerous functional molecules. Therefore, the ongoing interest for developing new versatile and efficient syntheses of heterocycles has always been a challenge in the synthetic community. Heterocycles are especially important in chemical and pharmaceutical industries. The traditional and conventional methodologies mostly used for the synthesis of these heterocycles, are reliable and robust and proceed generally at low cost, but these methodologies suffered with waste byproducts and harsh reaction conditions. In this regards, multicomponent reactions (MCRs) minimize such waste and are in general environmentally benign. Heterocycles having a complicated structure with many labile functional groups can be synthesized often from rather simple easy accessible starting materials through sequential catalytic processes in one-step.
In the past decade developments in the multicomponent reactions (MCRs) have greatly increased. More development in the area of multicomponent reactions (MCRs) in near upcoming research is required for the synthesis of a variety of heterocyclic compounds. The ability to perform tandem reactions to construct complex heterocyclic scaffolds makes the multicomponent reactions (MCRs) a potentially powerful synthetic tool. We hope that this review will be useful not only for synthetic organic chemists but also for heterocyclic and natural product synthetic chemists.
7. ACKNOWLEDGEMENTS
P. K. Maji and S. K. Bera gratefully acknowledge UGC, India for financial support under Minor Research Programme. R. U. Islam gratefully acknowledges Department of Applied Chemistry, Birla Institute of Technology Mesra, Ranchi, India for financial support. The authors are also grateful to all the co-workers whose work is described in this review for their contribution to the development of metal-catalyzed multicomponent heterocycles syntheses.
References
1. The Chemistry of Heterocycles: Structure, Reactions, Synthesis, and Applications, ed. by T. Eicher and S. Hauptmann; Wiley-VCH: Weinheim, 2003.
2. R. Kumar, K. S. Nagabhushana, and N. W. Mal, Chem. Indus. Digest Ann., 2009, 21, 135.
3. For a recent monograph, see Multicomponent Reactions, ed. by J. Zhu and H. Bienaymé, Wiley-VCH: Weinheim, 2005.
4. For reviews, see a) A. Dömling, Chem. Rev., 2006, 106, 17; CrossRef b) R. V. A. Orru and M. de Greef, Synthesis, 2003, 1471; CrossRef c) H. Bienaymé, C. Hulme, G. Oddon, and P. Schmitt, Chem. Eur. J., 2000, 6, 3321; CrossRef d) A. Dömling and I. Ugi, Angew. Chem. Int. Ed., 2000, 39, 3168; CrossRef e) I. Ugi, A. Dömling, and B. Werner, J. Heterocycl. Chem., 2000, 37, 647; CrossRef f) L. Weber, K. Illgen, and M. Almstetter, Synlett, 1999, 366; CrossRef g) R. W. Armstrong, A. P Combs, P. A. Tempest, S. D. Brown, and T. A. Keating, Acc. Chem. Res., 1996, 29, 123; CrossRef h) I. Ugi, A. Dömling, and W. Hörl, Endeavour, 1994, 18, 115; CrossRef i) G. H. Posner, Chem. Rev., 1986, 86, 831. CrossRef
5. For reviews on diversity oriented syntheses, see a) T. J. J. Müller and D. M. D’Souza, Pure Appl. Chem., 2008, 80, 609; CrossRef b) T. J. J. Müller, Functional Organic Materials. Syntheses, Strategies, and Applications, ed. by T. J. J. Müller and U. H. F. Bunz, Wiley-VCH Verlag GmbH & Co. KGaA; Weinheim, 2007, p179; c) S. L. Schreiber and M. D. Burke, Angew. Chem. Int. Ed., 2004, 43, 46; CrossRef d) M. D. Burke, E. M. Berger, and S. L. Schreiber, Science, 2003, 302, 613; CrossRef e) P. Arya, D. T. H. Chou, and M. G. Baek, Angew. Chem. Int. Ed. Engl., 2001, 40, 339; CrossRef f) B. Cox, J. C. Denyer, A. Binnie, M. C. Donnelly, B. Evans, D. V. S. Green, J. A. Lewis, T. H. Mander, A. T. Merritt, M. J. Valler, and S. P. Watson, Progr. Med. Chem., 2000, 37, 83; CrossRef g) S. L. Schreiber, Science, 2000, 287, 1964. CrossRef
6. D. Y. Murzin and R. Leino, Chem. Eng. Res. Design, 2008, 86, 1002. CrossRef
7. a) Pyrroles, Part II, ed. by R. A. Jones, Wiley: New York, 1992; b) B. A. Trofimov, L. N. Sobenina, A. P. Demenev, and A. I. Mikhaleva, Chem. Rev., 2004, 104, 2481; CrossRef c) F. Bellina and R. Rossi, Tetrahedron, 2006, 62, 7213; CrossRef d) C. F. Lee, L. M. Yang, T. Y. Hwu, and T. Y. Luh, J. Am. Chem. Soc., 2000, 122, 4992; CrossRef e) C. W. Boyce, M. A. Labrili, C. A. Sehon, and Q. Jin, J. Am. Chem. Soc., 1999, 121, 54; CrossRef f) V. M. Domingo, C. Aleman, E. Brillas, and L. Julia, J. Org. Chem., 2001, 66, 4058. CrossRef
8. a) A. Hantzsch, Ber., 1890, 23, 1474; CrossRef b) F. Palacios, D. Aparico, J. M. Santos, and J. Vicario, Tetrahedron, 2001, 57, 1961; CrossRef c) A. W. Trautwein, R. D. Sussmuth, and G. Jung, Bioorg. Med. Chem. Lett., 1998, 8, 2381. CrossRef
9. a) L. Knorr, Ber. Dtsch. Chem. Ges., 1884, 17, 1635; CrossRef b) C. Paal, Ber. Dtsch. Chem. Ges., 1885, 18, 367; CrossRef c) B. M. Trost and G. A. Doherty, J. Am. Chem. Soc., 2000, 122, 3801. CrossRef
10. a) J. L. Bullington, R. R. Wolff, and P. F. Jackson, J. Org. Chem., 2002, 67, 9439; CrossRef b) A. R. Katritzky, S. Zhang, M. Wang, H. C. Kolb, and P. J. Steel, J. Heterocycl. Chem., 2002, 39, 759; CrossRef c) K.-I. Washizuka, S. Minakata, I. Ryu, and M. Komatsu, Tetrahedron, 1999, 55, 12969. CrossRef
11. For selected examples, see: a) E. Benedetti, G. Lemiere, L.-L. Chapellet, A. Penoni, G. Palmisano, M. Malacria, J.-P. Goddard, and L. Fensterband, Org. Lett., 2010, 12, 4396; CrossRef b) S. Maiti, S. Biswas, and U. Jana, J. Org. Chem., 2010, 75, 1674; CrossRef c) A. Saito, O. Konishi, and Y. Hanzawa, Org. Lett., 2010, 12, 372; CrossRef d) J. T. Binder and S. F. Kirsch, Org. Lett., 2006, 8, 2151; CrossRef e) S. Kramer, J. L. H. Madsen, M. Rottlander, and T. Skrydstrup, Org. Lett., 2010, 12, 2758; CrossRef f) S. Chiba, Y.-F. Wang, G. Lapointe, and K. Narasaka, Org. Lett., 2008, 10, 313. CrossRef
12. E. Merkul, C. Boersch, W. Frank, and T. J. J. Müller, Org. Lett., 2009, 11, 2269. CrossRef
13. X. Chen, L. Hou, and X. Li, Synlett, 2009, 828. CrossRef
14. S. Dey, C. Pal, D. Nandi, V. S. Giri, M. Zaidlewicz, M. Krzeminski, L. Smentek, B. A. Hess, J. Gawronski, M. Kwit, N. J. Babu, A. Nangia, and P. Jaisankar, Org. Lett., 2008, 10, 1373. CrossRef
15. V. Cadierno, J. Gimeno, and N. Nebra, Chem. Eur. J., 2007, 13, 9973. CrossRef
16. B. Gabriele, L. Veltri, R. Mancuso, G. Salerno, S. Maggi, and B. M. Aresta, J. Org. Chem., 2012, 77, 4005. CrossRef
17. T. Murata, M. Murai, Y. Ikeda, K. K. Miki, and K. Ohe, Org. Lett., 2012, 14, 2296. CrossRef
18. E. Ghabraie, S. Balalaie, M. Bararjanian, H. R. Bijanzadeh, and F. Rominger, Tetrahedron, 2011, 67, 5415. CrossRef
19. D. Hong, Y. Zhu, Y. Li, X. Lin, P. Lu, and Y. Wang, Org. Lett., 2011, 13, 4668. CrossRef
20. S. Zhang, J. Zhao, W.-X. Zhang, and Z. Xi, Org. Lett., 2011, 13, 1626. CrossRef
21. C. V. Galliford and K. A. Scheidt, J. Org. Chem., 2007, 72, 1811. CrossRef
22. G. Dou, C. Shi, and D. Shi, J. Comb. Chem., 2008, 10, 810. CrossRef
23. G. Shanthi and P. T. Perumal, Tetrahedron Lett., 2009, 50, 3959. CrossRef
24. A. T. Khan, M. Lal, P. R. Bagdi, R. S. Basha, P. Saravanan, and S. Patra, Tetrahedron Lett., 2012, 53, 4145. CrossRef
25. a) J. E. Saxton, Nat. Prod. Rep., 1997, 14, 559; CrossRef b) M. Toyota and N. Ihara, Nat. Prod. Rep., 1998, 15, 327; CrossRef c) J. A. Joule, Product Class 13: Indole and Its Derivatives; Thieme: Stuttgart, 2001; d) T. Kawasaki and K. Higuchi, Nat. Prod. Rep., 2005, 22, 761; CrossRef e) G. R. Humphrey and J. T. Kuethe, Chem. Rev., 2006, 106, 2875. CrossRef
26. For reviews on indole chemistry, see: a) R. J. Sundberg, Indoles; Academic: London, 1996; b) A. R. Katritzky and A. F. Pozharskii, Handbook of Heterocyclic Chemistry; Pergamon: Oxford, 2000; Chapter 4; c) J. J. Li and G. W. Gribble, In Palladium in Heterocyclic Chemistry; Pergamon: Oxford, 2000; Chapter 3; d) K. Kruger, A. Tillack, and M. Beller, Adv. Synth. Catal., 2008, 350, 2139. CrossRef
27. a) E. Fischer and F. Jourdan, Ber. Dtsch. Chem. Ges., 1883, 16, 2241; CrossRef b) E. Fischer and O. Hess, Ber. Dtsch. Chem. Ges., 1884, 17, 559; CrossRef c) B. Robinson, The Fischer Indole Synthesis; John Wiley and Sons: Chichester, 1982; d) D. L. Hughes, Org. Prep. Proced. Int., 1993, 25, 607; CrossRef e) L. E. Kaim, L. Grimaud, and C. Ronsseray, Synlett, 2010, 2296. CrossRef
28. a) S. Cacchi and G. Fabrizi, Chem. Rev., 2005, 105, 2873; CrossRef b) C. V. Stevens, E. Van Meenen, Y. Eeckhout, B. Vanderhoydonck, and W. Hooghe, Chem. Commun., 2005, 38, 4827; CrossRef c) N. Batail, A. Bendjeriou, L. Djakovitch, and V. Dufaud, Appl. Catal., A, 2010, 388, 179; d) S. V. Jeught, N. D. Vos, K. Masschelein, I. Ghiviriga, and C. V. Stevens, Eur. J. Org. Chem., 2010, 5444. CrossRef
29. a) R. C. Larock and S. Babu, Tetrahedron Lett., 1987, 28, 1037; b) R. C. Larock and E. K. Yum, J. Am. Chem. Soc., 1991, 113, 6689; CrossRef c) R. C. Larock, E. K. Yum, and M. D. Refvik, J. Org. Chem., 1998, 63, 7652; CrossRef d) G. Huang and R. C. Larock, J. Org. Chem., 2003, 68, 7342; CrossRef e) Y. Jia and J. Zhu, J. Org. Chem., 2006, 71, 7826; CrossRef f) J. Zhao and R. C. Larock, J. Org. Chem., 2006, 71, 5340; CrossRef g) G. Zeni and R. C. Larock, Chem. Rev., 2006, 106, 4644; CrossRef h) S. Patil and J. K. Buolamwini, Curr. Org. Synth., 2006, 3, 477. CrossRef
30. J. Barluenga, A. Jiménez-Aquino, C. Valdés, and F. Aznar, Angew. Chem. Int. Ed., 2007, 46, 1529. CrossRef
31. Z. Chen, D. Zheng, and J. Wu, Org. Lett., 2011, 13, 848. CrossRef
32. Y. Liu and J.-W. Sun, J. Org. Chem., 2012, 77, 1191. CrossRef
33. D. Marosvolgyi-Hasko, A. Petz, A. Takacs, and L. Kollar, Tetrahedron, 2011, 67, 9122. CrossRef
34. a) N. L. Chavan, S. K. Nayak, and R. S. Kusurkar, Tetrahedron, 2010, 66, 1827; CrossRef b) F. Liger, F. Popowycz, T. Besson, L. Picot, C. M. Galmarini, and B. Joseph, Bioorg. Med. Chem., 2007, 15, 5615; CrossRef c) J. R. Dormoy and A. Heymes, Tetrahedron, 1993, 49, 2885; CrossRef d) M.Alvarez, D. Fernandez, and J. A. Joule, Synthesis, 1999, 615; CrossRef e) N. R. Curtius, J. J. Kulagowski, P. D. Leeson, M. P. Redgill, F. Emms, S. B. Freedman, and S. Patel, Bioorg. Med. Chem. Lett., 1999, 9, 585. CrossRef
35. R. Suresh, S. Muthusubramanian, R. Senthilkumaran, and G. Manickam, J. Org. Chem., 2012, 77, 1468. CrossRef
36. Y. Liang, T. Meng, H.-J. Zhang, and Z. Xi, Synlett, 2011, 911. CrossRef
37. The first report for ketenimine, see: H. Staudinger and E. Hauser, Helv. Chim. Acta, 1921, 4, 887. CrossRef
38. For reviews on formation and synthetic applications of ketenimines, see: a) P. Lu and Y. G. Wang, Synlett, 2010, 165; CrossRef b) E. J. Yoo and S. Chang, Curr. Org. Chem., 2009, 13, 1766; CrossRef c) J. E. Hein and V. V. Fokin, Chem. Soc. Rev., 2010, 39, 1302; CrossRef d) G. R. Krow, Angew. Chem., Int. Ed. Engl., 1971, 10, 435. CrossRef
39. a) V. V. Rostovtsev, L. G. Green, V. V. Fokin, and K. B. Sharpless, Angew. Chem. Int. Ed., 2002, 41, 2596; CrossRef b) C. W. Tornøe, C. Christensen, and M. Meldal, J. Org. Chem., 2002, 67, 3057; CrossRef c) E. J. Yoo, M. Ahlquist, I. Bae, K. B. Sharpless, V. V. Fokin and S. Chang, J. Org. Chem., 2008, 73, 5520. CrossRef
40. a) I. Bae, H. Han, and S. Chang, J. Am. Chem. Soc., 2005, 127, 2038; CrossRef b) S. H. Cho, E. J. Yoo, I. Bae, and S. Chang, J. Am. Chem. Soc., 2005, 127, 16046; CrossRef c) S. H. Cho and S. Chang, Angew. Chem. Int. Ed., 2007, 46, 1897; CrossRef d) E. J. Yoo and S. Chang, Org. Lett., 2008, 10, 1163; CrossRef e) J. Kim, Y. Lee, J. Lee, Y. Do, and S. Chang, J. Org. Chem., 2008, 73, 9454; CrossRef f) E. J. Yoo, S. H. Park, S. H. Lee, and S. Chang, Org. Lett., 2009, 11, 1155; CrossRef g) S. L. Cui, X. F Lin, and Y. G. Wang, Org. Lett., 2006, 8, 4517; CrossRef h) S. L. Cui, J. Wang, and Y. G. Wang, Org. Lett., 2007, 9, 5023; CrossRef i) J. She, Z. Jiang, and Y. G. Wang, Synlett, 2009, 2023; CrossRef j) W. Lu, S. W. Song, D. Hong, P. Lu, and Y. G. Wang, Adv. Synth. Catal., 2009, 351, 1768; CrossRef k) H. W. Jin, X. L. Xu, J. R. Gao, J. H. Zhong, and Y. G. Wang, Adv. Synth. Catal., 2010, 352, 347; CrossRef l) M. P. Cassidy, J. Raushel, and V. V. Fokin, Angew. Chem., Int. Ed., 2006, 45, 3154; CrossRef m) M. Whiting and V. V. Fokin, Angew. Chem. Int. Ed., 2006, 45, 3157; CrossRef n) X. Xu, D. Cheng, J. Li, H. Guo, and J. Yan, Org. Lett., 2007, 9, 1585. CrossRef
41. H. Jin, B. Zhou, Z. Wu, Y. Shen, and Y. Wang, Tetrahedron, 2011, 67, 1178. CrossRef
42. Y. Suzuki, Y. Ohta, S. Oishi, N. Fujii, and H. Ohno, J. Org. Chem., 2009, 74, 4246. CrossRef
43. B. Yan and Y. Liu, Org. Lett., 2007, 9, 4323. CrossRef
44. S. Mishra, B. Naskar, and R. Ghosh, Tetrahedron Lett., 2012, 53, 5483. CrossRef
45. a) M. H. Chen, P. Pollard, A. Patchett, K. Cheng, L. Wei, W. S. Chan, B. Buttler, T. M. Jacks, and R. G. Smith, Bioorg. Med. Chem. Lett., 1999, 9, 261; CrossRef b) R. K. Behara, A. K. Behara, R. Pradhan, A. Pati, and M. Patra, Synth. Commun., 2006, 36, 3729. CrossRef
46. a) C. Marti and E. M. Carreira, Eur. J. Org. Chem., 2003, 2209; CrossRef b) A. Millemaggi and R. J. K. Taylor, Eur. J. Org. Chem., 2010, 4527. CrossRef
47. A. Pinto, L. Neuville, and Z. Zhu, Tetrahedron Lett., 2009, 50, 3602. CrossRef
48. M. Genelot, A. Bendjeriou, V. Dufaud, and L. Djakovitch, Appl. Catal. A: Gen., 2009, 369, 125. CrossRef
49. C. S. Cho and W. S. Ren, Tetrahedron Lett., 2009, 50, 2097. CrossRef
50. D. Marosvolgyi-Hasko, A. Takacs, Z. Riedl, and L. Kollar, Tetrahedron, 2011, 67, 1036. CrossRef
51. D. Marosvolgyi-Hasko, A. Petz, A. Takacs, and L. Kollar, Tetrahedron, 2011, 67, 9122. CrossRef
52. C. L. Floch, C. Bughin, E. L. Gall, E. Léonel, and T. Martens, Tetrahedron Lett., 2009, 50, 5456. CrossRef
53. C. Qi, H. Jiang, L. Huang, G. Yuan, and Y. Ren, Org. Lett., 2011, 13, 5520. CrossRef
54. S. Li and S. Ma, Org. Lett., 2011, 13, 6046. CrossRef
55. R.-V. Nguyen and C.-J. Li, Synlett, 2008, 1897. CrossRef
56. H. Li, J. Liu, B. Yan, and Y. Li, Tetrahedron Lett., 2009, 50, 2353. CrossRef
57. N. Sakai, N. Uchida, and T. Konakahara, Tetrahedron Lett., 2008, 49, 3437. CrossRef
58. L. Zhao, G. Cheng, and Y. Hu, Tetrahedron Lett., 2008, 49, 7364. CrossRef
59. For general reviews, see a) A. N. Kost and I. I. Grandberg, Adv. Heterocycl. Chem., 1966, 6, 347; CrossRef b) J. Elguero, In Comprehensive Heterocyclic Chemistry, ed. by A. R. Katritzky and C. W. Rees, Pergamon: Oxford, 1984; Vol. 5, p 167; CrossRef c) J. Elguero, In Comprehensive Heterocyclic Chemistry II, ed. by A. R. Katritzky, C. W. Rees, and E. F. V. Scriven, Elsevier: Oxford, 1996; Vol. 3, p 1. CrossRef
60. D. J. Wustrow, T. Capiris, R. Rubin, J. A. Knobelsdorf, H. Akunne, M. D. Davis, R. MacKenzie, T. A. Pugsley, K. T. Zoski, T. G. Heffner, and L. D. Wise, Bioorg. Med. Chem. Lett., 1998, 8, 2067. CrossRef
61. T. D. Penning, J. J. Talley, S. R. Bertenshaw, J. S. Carter, P. W. Collins, S. Docter, M. J. Graneto, L. F. Lee, J. W. Malecha, J. M. Miyashiro, R. S. Rogers, D. J. Rogier, S. S. Yu, G. D. Anderson, E. G. Burton, J. N. Cogburn, S. A. Gregory, C. M. Koboldt, W. E. Perkins, K. Seibert, A. W. Veenhuizen, Y. Y. Zhang, and P. C. Isakson, J. Med. Chem., 1997, 40, 1347. CrossRef
62. H.-L. Liu, H.-F. Jiang, M. Zhang, W.-J. Yao, Q.-H. Zhu, and Z. Tang, Tetrahedron Lett., 2008, 49, 3805. CrossRef
63. B. Willy and T. J. J. Müller, Eur. J. Org. Chem., 2008, 4157. CrossRef
64. B. Willy and T. J. J. Müller, Org. Lett., 2011, 13, 2082. CrossRef
65. L. Shen, S. Cao, N. Liu, J. Wu, L. Zhu, and X. Quin, Synlett, 2008, 1341. CrossRef
66. S. Majumder, K. R. Gipson, R. J. Staples, and A. L. Odom, Adv. Synth. Catal., 2009, 351, 2013. CrossRef
67. K. Kumari, D. S. Raghuvanshi, V. Jouikov, and K. N. Singh, Tetrahedron Lett., 2012, 53, 1130. CrossRef
68. M. R. Kumar, A. Park, N. Park, and S. Lee, Org. Lett., 2011, 13, 3542. CrossRef
69. X.-M. Yan, Z.-M. Chen, F. Yang, and Z.-Z. Huang, Synlett, 2011, 569. CrossRef
70. Y. Suzuki, S. Naoe, S. Oishi, N. Fujii, and H. Ohno, Org. Lett., 2012, 14, 326. CrossRef
71. J. P. Stonehouse, D. S. Chekmarev, N. V. Ivanova, S. Lang, G. Pairaudeau, N. Smith, M. J. Stocks, S. I. Sviridov, and L. M. Utkina, Synlett, 2008, 100. CrossRef
72. S. Safaei, I. Mohammadpoor-Baltork, A. R. Khosropour, M. Moghadam, S. Tangestaninejad, and V. Mirkhani, Synlett, 2011, 2214. CrossRef
73. Y. Li, D. Hong, Y. Zhu, P. Lu, and Y. Wang, Tetrahedron, 2011, 67, 8086. CrossRef
74. S. Li, Y. Luo, and J. Wu, Org. Lett., 2011, 13, 4312. CrossRef
75. a) R. M. Chabin, E. McCauley, J. R. Calaycay, T. M. Kelly, K. L. MacNaul, G. C. Wolfe, N. I. Hutchinson, S. Madhusudanaraju, J. A. Schmidt, J. W. Kozarich, and K. K. Wong, Biochemistry, 1996, 35, 9567; CrossRef b) N. P. Jensen, S. M. Schmitt, T. B. Windholz, and T. Y. Shen, J. Med. Chem., 1972, 15, 341; CrossRef c) L. R. Swett and T. O. Yellin, J. Med. Chem., 1970, 13, 968; CrossRef d) M. M. Herrador, J. S. Buruaga, and M. D. Suarez, J. Med. Chem., 1985, 28, 146; CrossRef e) J. Gising, M. T. Nilsson, L. R. Odell, S. Yahiaoui, M. Lindh, H. Iyer, A. M. Sinha, B. R. Srinivasa, M. Larhed, S. L. Mowbray, and A. Karlen, J. Med. Chem., 2012, 55, 2894; CrossRef f) J. G. Lombardino and E. H. Wiseman, J. Med. Chem., 1974, 17, 1182; CrossRef g) S. D. Zaric, D. M. Popovic, and E. W. Knapp, Biochemistry, 2001, 40, 7914. CrossRef
76. a) A. Bhatnagar, P. K. Sharma, and N. Kumar, Int. J. PharmTech. Res., 2011, 3, 268; b) L. De Luca, Curr. Med. Chem., 2006, 13, 1; c) M. Boiani and M. Gonzalez, Mini-Rev. Med. Chem., 2005, 5, 68. CrossRef
77. L. Bromberg, L. Chen, E. P. Chang, S. Wang, and T. A. Hatton, Chem. Mater., 2010, 22, 538. CrossRef
78. a) H. Debus, Ann., 1858, 107, 204; b) B. Radziszewski, Ber. Dtsch. Chem. Ges., 1882, 15, 2706. CrossRef
79. C. F. Huebner, J. Am. Chem. Soc., 1951, 73, 4667. CrossRef
80. a) V. Gracias, A. F. Gasiecki, and S. W. Djuric, Tetrahedron Lett., 2005, 46, 9049; CrossRef b) V. Gracias, D. Darczak, A. F. Gasiecki, and S. W. Djuric, Tetrahedron Lett., 2005, 46, 9053; CrossRef c) V. Gracias, A. F. Gasiecki, T. G. Pagano, and S. W. Djuric, Tetrahedron Lett., 2006, 47, 8873; CrossRef d) X. Beebe, V. Gracias, and S. W. Djuric, Tetrahedron Lett., 2006, 47, 3225; CrossRef e) L. Wang, K. W. Woods, Q. Li, K. J. Barr, R. W. McCroskey, S. M. Hannick, L. Gherke, R. B. Credo, Y. H. Hui, K. Marsh, R. Warner, J. Y. Lee, N. Zielinski-Mozng, D. Frost, S. H. Rosenberg, and H. L. Sham, J. Med. Chem., 2002, 45, 1697. CrossRef
81. a) F. De Moliner and C. Hulme, Org. Lett., 2012, 14, 1354; CrossRef b) S. Li, Z. K. Li, Y. F. Yuan, D. J. Peng, Y. J. Li, L. S. Zhang, and Y. M. Wu, Org. Lett., 2012, 14, 1130; CrossRef c) Y. J. Xiao and L. M. Zhang, Org. Lett., 2012, 14, 4662; CrossRef d) B. Hu, Z. Wang, N. Ai, J. Zheng, X. H. Liu, S. Shan, and Z. W. Wang, Org. Lett., 2011, 13, 6362; CrossRef e) S. Petit, C. Fruit, and L. Bischoff, Org. Lett., 2010, 12, 4928; CrossRef f) B. Jiang, X. Wang, F. Shi, S. J. Tu, T. Ai, A. Ballew, and G. Li, J. Org. Chem., 2009, 74, 9486. CrossRef
82. S. Das Sharma, P. Hazarika, and D. Konwar, Tetrahedron Lett., 2008, 49, 2216. CrossRef
83. J. Han, T. Li, Y. Pan, A. Kattuboina, and G. Li, Chem. Biol. Drug Des., 2008, 71, 71. CrossRef
84. K. Worrall, B. Xu, S. Bontemps, and B. A. Arndtsen, J. Org. Chem., 2011, 76, 170. CrossRef
85. a) S. Bontemps, J. S. Quesnel, K. Worrall, and B. A. Arndtsen, Angew. Chem. Int. Ed., 2011, 50, 8949; CrossRef b) R. Dhawan, R. D. Dghaym, D. J. St. Cyr, and B. A. Arndtsen, Org. Lett., 2006, 8, 3927. CrossRef
86. B. Xu, K. Worrall, and B. A. Arndtsen, Molecules, 2012, 17, 13759. CrossRef
87. Z. Jiang, P. Lu, and Y. Wang, Org. Lett., 2012, 14, 6266. CrossRef
88. E. F. DiMauro and J. M. Kennedy, J. Org. Chem., 2007, 72, 1013. CrossRef
89. S. Rostamnia, K. Lamei, M. Mohammadquli, M. Sheykhan, and A. Heydari, Tetrahedron Lett., 2012, 53, 5257. CrossRef
90. B. V. Subha Reddy, P. Shivaramkrishna Reddy, Y. Jayasudhan Reddy, and J. S. Yadav, Tetrahedron Lett., 2011, 52, 5789. CrossRef
91. P. Liu, L.-S. Fang, X. Lei, and G.-Q. Lin, Tetrahedron Lett., 2010, 51, 4605. CrossRef
92. S. Mishra and R. Ghosh, Synthesis, 2011, 3463. CrossRef
93. T. Guntreddi, B. K. Allam, and K. N. Singh, Synlett, 2012, 2635. CrossRef
94. S. K. Guchhait, A. L. Chandgude, and G. Priyadarshani, J. Org. Chem., 2012, 77, 4438. CrossRef
95. Y. Kim, M. R. Kumar, N. Park, Y. Heo, and S. Lee, J. Org. Chem., 2011, 76, 9577. CrossRef
96. B. Willy, F. Rominger, and T. J. J. Müller, Synthesis, 2008, 293. CrossRef
97. P. J. Boissarie, Z. E. Hamilton, S. Lang, J. A. Murphy, and C. J. Suckling, Org Lett., 2011, 13, 6256. CrossRef
98. B. Gabriele, P. Plastina, G. Salerno, R. Mancuso, and M. Costa, Org. Lett., 2007, 9, 3319. CrossRef
99. W.-J. Yoo and C.-J. Li, Adv. Synth. Catal., 2008, 350, 1503. CrossRef
100. G. Satish, K. H. V. Reddy, K. Ramesh, K. Karnakar, and Y. V. D. Nageswar, Tetrahedron Lett., 2012, 53, 2518. CrossRef
101. L. Shi, X. Liu, H. Zhang, Y. Jiang, and D. Ma, J. Org. Chem., 2011, 76, 4200. CrossRef
102. K. C. Majumdar, N. De, D. Ghosh, S. Ponra, and B. Roy, Synthesis, 2012, 87. CrossRef
103. S. Muthusamy, R. Ramkumar, and A. K. Mishra, Tetrahedron Lett., 2011, 52, 148. CrossRef
104. R. D. Baxter and J. Montgomery, J. Am. Chem. Soc., 2008, 130, 9662. CrossRef
105. a) R. Huisgen, G. Szeimies, and L. Moebius, Chem. Ber., 1965, 98, 4014; CrossRef b) R. Huisgen, Pure Appl. Chem., 1989, 61, 613. CrossRef
106. C. W. Tornøe, C. Christensen, and M. Meldal, J. Org. Chem., 2002, 67, 3057. CrossRef
107. V. V. Rostovtsev, L. G. Green, V. V. Fokin, and K. B. Sharpless, Angew. Chem. Int. Ed., 2002, 41, 2596. CrossRef
108. a) V. D. Bock, H. Hiemstra, and J. H. van Maarseveen, Eur. J. Org. Chem., 2006, 51; CrossRef b) M. Meldal and C. W. Tornøe, Chem. Rev., 2008, 108, 2952; CrossRef c) P. Appukkuttan and E. Van der Eycken, Eur. J. Org. Chem., 2008, 1133; CrossRef d) C. O. Kappe and E. Van der Eycken, Chem. Soc. Rev., 2010, 39, 1280. CrossRef
109. a) J. A. Anderson and M. F. García, ed., Catalytic Science Series; Imperial College Press: London, 2005; b) K. Kaneda, K. Ebitani, T. Mizugaki, and K. Mori, Bull. Chem. Soc. Jpn., 2006, 79, 981. CrossRef
110. For recent reviews, see a) J. Sun and X. Bao, Chem. Eur. J., 2008, 14, 7478; CrossRef b) R. J. White, R. Luque, V. L. Budarin, J. H. Clark, and D. J. Macquarrie, Chem. Soc. Rev., 2009, 38, 481; CrossRef c) J. M. Campelo, D. Luna, F. Luque, J. M. Marinas, and A. A. Romero, ChemSusChem., 2009, 2, 18; CrossRef d) L. De Rogatis, M. Cargnello, V. Gombac, B. Lorenzut, T. Montini, and P. Fornasiero, ChemSusChem., 2010, 3, 24; CrossRef e) L. D. Pachon, J. H. van Maarseveen, and G. Rothenberg, Adv. Synth. Catal., 2005, 347, 811. CrossRef
111. For recent reviews, see a) A. Chanda and V. V. Fokin, Chem. Rev., 2009, 109, 725; CrossRef b) R. N. Butler and A. G. Coyne, Chem. Rev., 2010, 110, 6302. CrossRef
112. For recent reviews, see a) K. Kumaravel and G. Vasuki, Curr. Org. Chem., 2009, 13, 1820; CrossRef b) E. J. Yoo and S. Chang, Curr. Org. Chem., 2009, 13, 1766; CrossRef c) B. Jiang, T. Rajale, W. Wever, S.-J. Tu, and G. Li, Chem. Asian J., 2010, 5, 2318; CrossRef d) D. J. Ramon and M. Yus, Angew. Chem. Int. Ed., 2005, 44, 1602. CrossRef
113. a) M. Laksmi Kantam, V. S. Jaya, B. Sreedhar, M. Mohan Rao, and B. M. Choudary, J. Mol. Catal. A, 2006, 256, 273; CrossRef b) H. Sharghi, R. Khalifeh, and M. M. Doroodmand, Adv. Synth. Catal., 2009, 351, 207; CrossRef c) V. Beneteau, A. Olmos, T. Boningari, J. Sommer, and P. Pale, Tetrahedron Lett., 2010, 51, 3673. CrossRef
114. L. Ackermann, H. K. Potukuchi, D. Landsberg, and R. Vicente, Org. Lett., 2008, 10, 3081. CrossRef
115. J. Kalisiak, K. B. Sharpless, and V. V. Fokin, Org. Lett., 2008, 10, 3171. CrossRef
116. S.-I. Fukuzawa, E. Shimizu and S. Kikuchi, Synlett, 2007, 2436. CrossRef
117. H. S. G. Beckmann and V. Wittmann, Org. Lett., 2007, 9, 1. CrossRef
118. K. Barral, A. D. Moorhouse, and J. E. Moses, Org. Lett., 2007, 9, 1809. CrossRef
119. A. D. Moorhouse and J. E. Moses, Synlett, 2008, 2089. CrossRef
120. J. S. Jadav, B. V. Subba Reddy, G. Madhusudhan Reddy, and D. N. Chary, Tetrahedron Lett., 2007, 48, 8773. CrossRef
121. a) F. Alonso, Y. Moglie, G. Radivoy, and M. Yus, Tetrahedron Lett., 2009, 50, 2358; CrossRef b) A. Sarkar, T. Mukherjee, and S. Kapoor, J. Phys. Chem. C, 2008, 112, 3334; CrossRef c) H. Elayadi, M. A. Ali, A. Mehdi, and H. B. Lazrek, Catal. Commun., 2012, 26, 155; CrossRef d) R. B. N. Baig and R. S. Varma, Green Chem., 2012, 14, 625; CrossRef e) R. Hudson, C.-J. Li, and A. Moores, Green Chem., 2012, 14, 622. CrossRef
122. F. Alonso, Y. Moglie, G. Radivoy and M. Yus, J. Org. Chem., 2011, 76, 8394. CrossRef
123. a) S. Grasso, G. Desarro, N. Micale, M. Zappala, G. Puia, M. Baraldi, and C. Demicheli, J. Med. Chem., 2000, 43, 2851; CrossRef b) N. Watanabe, Y. Kabasawa, Y. Takase, M. Matsukura, K. Miyazaki, H. Ishihara, K. Kodama, and H. Adachi, J. Med. Chem., 1998, 41, 3367; CrossRef c) P. Salehi, D. I. MaGee, M. Dabiri, L. Torkian, and J. Donahue, Mol. Divers., 2012, 16, 231. CrossRef
124. B. Sreedhar, P. S. Seddy, and V. Rama Krishna, Tetrahedron Lett., 2007, 48, 5831. CrossRef
125. W. Qian, D. Winternheimer, and J. Allen, Org. Lett., 2011, 13, 1682. CrossRef
126. J. S. Yadav, B. V. Subba Reddy, G. Madhusudhan Reddy, and S. Rehana Anjum, Tetrahedron Lett., 2009, 50, 6029. CrossRef
127. J. S. Yadav, B. V. S. Reddy, D. N. Chary, and C. S. Reddy, Tetrahedron Lett., 2008, 49, 2649. CrossRef
128. H. Ankati, Tetrahedron Lett., 2009, 50, 4677. CrossRef
129. B. S. P. A. Kumar, K. H. V. Reddy, B. Madhav, K. Ramesh, and Y. V. D. Nageswar, Tetrahedron Lett., 2012, 53, 4595. CrossRef
130. F. Alonso, Y. Moglie, G. Radivoy, and M. Yus, Synlett, 2012, 2179. CrossRef
131. S. Ladouceur, A. M. Soliman, and E. Zysman-Colman, Synthesis, 2011, 3604. CrossRef
132. S. Sarkar, S. Dutta, and A. K. Sen, Synthesis, 2012, 1079. CrossRef
133. N. Li, D. Wang, J. Li, W. Shi, C. Li and B. Chen, Tetrahedron Lett., 2011, 52, 980. CrossRef
134. L. Li, G. Zhang, A. Zhu, and L. Zhang, J. Org. Chem., 2008, 73, 3630. CrossRef
135. J. T. Fletcher and J. E. Reilly, Tetrahedron Lett., 2011, 52, 5512. CrossRef
136. a) F. X. Felpin and E. Fouquet, ChemSusChem, 2008, 1, 718; CrossRef b) M. G. Finn and V. V. Fokin, Chem. Soc. Rev., 2010, 39, 1231; CrossRef c) P. Wu and V. V. Fokin, Aldrichimica. Acta, 2007, 40, 7.
137. S. Lal and S. Díez-Gonzalez, J. Org. Chem., 2011, 76, 2367. CrossRef
138. A. Kolarovic, M. Schnurch, and M. D. Mihovilovic, J. Org. Chem., 2011, 76, 2613. CrossRef
139. S. Mohammed, A. K. Padala, B. A. Dar, B. Singh, B. Sreedhar, R. A. Vishwakarma, and S. B. Bharate, Tetrahedron, 2012, 68, 8156. CrossRef
140. L. K. Rasmussen, B. C. Boren and V. V. Fokin, Org. Lett., 2007, 9, 5337. CrossRef
141. J. R. Johansson, P. Lincoln, B. Norden, and N. Kann, J. Org. Chem., 2011, 76, 2355. CrossRef
142. Y. Zhang, X. Li, J. Li, J. Chen, X. Meng, M. Zhao, and B. Chen, Org. Lett., 2012, 14, 26. CrossRef
143. D. Kundu, A. Majee, and A. Hajra, Tetrahedron Lett., 2009, 50, 2668. CrossRef
144. T. Yue, M.-X. Wang, D.-X. Wang, and J. Zhu, Angew. Chem. Int. Ed., 2008, 47, 9454. CrossRef
145. H. Yanai, T. Sakiyama, T. Oguchi and T. Taguchi, Tetrahedron Lett., 2012, 53, 3161. CrossRef
146. O. De Paolis, J. Baffoe, S. M. Landge, and B. Torok, Synthesis, 2008, 3423. CrossRef
147. P. V. Shinde, V. B. Labade, J. B. Gujar, B. B. Shingate, and M. S. Shingare, Tetrahedron Lett., 2012, 53, 1523. CrossRef
148. P. A. Clarke, A. V. Zaytzev, and A. C. Whitewood, Tetrahedron Lett., 2007, 48, 5209. CrossRef
149. G. Brahmachari and S. Das, Tetrahedron Lett., 2012, 53, 1479. CrossRef
150. S. Mishra and R. Ghosh, Tetrahedron Lett., 2011, 52, 2857. CrossRef
151. J. Barluenga, A. Jiménez-Aquino, M. A. Fernández, F. Aznar, and C. Valdés, Tetrahedron, 2008, 64, 778. CrossRef
152. N. M. Evdokimov, A. S. Kireev, A. A. Yakovenko, M. Y. Antipin, I. V. Magedov, and A. Kornienko, J. Org. Chem., 2007, 72, 3443. CrossRef
153. M. Sridhar, B. C. Ramanaiah, C. Narsaiah, B. Mahesh, M. Kumaraswamy, K. K. R. Mallu, V. M. Ankathi, and P. S. Rao, Tetrahedron Lett., 2009, 50, 3897. CrossRef
154. M. Thimmaiah, P. Li, S. Regati, B. Chen, and J. C.-G. Zhao, Tetrahedron Lett., 2012, 53, 4870. CrossRef
155. M. R. P. Heravi and F. Fakhr, Tetrahedron Lett., 2011, 52, 6779. CrossRef
156. J. Tang, L. Wang, Y. Yao, L. Zhang, and W. Wang, Tetrahedron Lett., 2011, 52, 509. CrossRef
157. N. Mahajan, A. Sambyal, A. P. S. Pannu, and T. K. Razdan, Tetrahedron Lett., 2011, 52, 1265. CrossRef
158. a) L. Zhao, F. Liang, X. Bi, S. Sun, and Q. Liu, J. Org. Chem., 2006, 71, 1094; CrossRef b) L. Dong, S. Aleem, and C. A. Fink, Tetrahedron Lett., 2010, 51, 5210. CrossRef
159. D. Yang, S. Burugupalli, D. Daniel, and Y. Chen, J. Org. Chem., 2012, 77, 4466. CrossRef
160. V. Sridharan, P. T. Perumal, C. Avendańo, and J. C. Menéndez, Tetrahedron, 2007, 63, 4407. CrossRef
161. S. Sueki, R. Takei, J. Abe, and I. Shimizu, Tetrahedron Lett., 2011, 52, 4473. CrossRef
162. E. Yamuna, M. Zeller, and K. J. R. Prasad, Tetrahedron Lett., 2011, 52, 6805. CrossRef
163. P. Mizar and B. Myrboh, Tetrahedron Lett., 2009, 50, 3088. CrossRef
164. A. A. Kudale, J. Kendall, D. O. Miller, J. L. Collins, and G. J. Bodwell, J. Org. Chem., 2008, 73, 8437. CrossRef
165. P. A. Clarke, A. V. Zaytsev, T. W. Morgan, A. C. Whitwood, and C. Wilson, Org. Lett., 2008, 10, 2877. CrossRef
166. P. J. Alaimo, R. O’Brien, III, A. W. Johnson, S. R. Slauson, J. M. O’Brien, E. L. Tyson, A.-L. Marshall, C. E. Ottinger, J. C. Chacon, L. Wallace, C. Y. Paulino, and S. Connell, Org. Lett., 2008, 10, 5111. CrossRef
167. N. Isambert, M. Cruz, M. J. Arévalo, E. Gómez, and R. Lavilla, Org. Lett., 2007, 9, 4199. CrossRef
168. V. Gaddam and R. Nagarajan, Tetrahedron Lett., 2009, 50, 1243. CrossRef
169. A. T. Khan, D. K. Das, and M. M. Khan, Tetrahedron Lett., 2011, 51, 4539. CrossRef
170. B. H. S. T. da Silva, L. M. Martins, and L. C. da silva-Filho, Synlett, 2012, 1973. CrossRef
171. E. Vicente-Garca, F. Catti, R. Ramón, and R. Lavilla, Org. Lett., 2010, 12, 860. CrossRef
172. E. Vicente-García, R. Ramón, and R. Lavilla, Synthesis, 2011, 2237. CrossRef
173. X. S. Wang, J. Zhou, K. Yang, and C. S. Yao, Tetrahedron Lett., 2010, 51, 5721. CrossRef
174. S. B. Sakal, K. F. Shelke, B. B. Shingate, and M. S. Shingare, Tetrahedron Lett., 2009, 50, 1754. CrossRef
175. M. Saha and A. K. Pal, Tetrahedron Lett., 2011, 52, 4872. CrossRef
176. J. M. Khurana, A. Chaudhary, B. Nand, and A. Lumb, Tetrahedron Lett., 2012, 53, 3018. CrossRef
177. P. S. Naidu, P. Borah, and P. J. Bhuyan, Tetrahedron Lett., 2012, 53, 4015. CrossRef
178. M. Dell’Acqua, G. Abbiati, and E. Rossi, Synlett, 2010, 2672. CrossRef
179. S. Ramesh and R. Nagarajan, Synlett, 2012, 717. CrossRef
180. A. Kumar and V. K. Rao, Synlett, 2011, 2157. CrossRef
181. For selected examples, see a) K. W. Bentley, The Isoquinoline Alkaloids; Harwood Academic: Australia, 1998; Vol. 1; b) J. D. Phillipson, M. F. Roberts, and M. H. Zenk, The Chemistry and Biology of Isoquinoline Alkaloids; Springer: New York, NY, 1985.
182. For selected examples, see a) B. W.Trotter, K. K. Nanda, N. R. Kett, C. P. Regan, J. J. Lynch, G. L. Stump, L. Kiss, J. Wang, R. H. Spencer, S. A. Kane, R. B. White, R. Zhang, K. D. Anderson, N. J. Liverton, C. J. McIntyre, D. C. Beshore, G. D. Hartman, and C. J. Dinsmore, J. Med. Chem., 2006, 49, 6954; CrossRef b) P. Ramesh, N. S. Reddy and Y. Venkateswarlu, J. Nat. Prod., 1999, 62, 780; CrossRef c) T. Kaneda, Y. Takeuchi, H. Matsui, K. Shimizu, N. Urakawa, and S. Nakajyo, J. Pharm. Sci., 2005, 98, 275; CrossRef d) C. Marchand, S. Antony, K. W. Kohn, M. Cushman, A. Ioanoviciu, B. L. Staker, A. B. Burgin, L. Stewart, and Y. Pommier, Mol. Cancer Ther., 2006, 5, 287. CrossRef
183. Z. Guo, M. Cai, J. Jiang, L. Yang, and W. Hu, Org. Lett., 2010, 12, 652. CrossRef
184. S. Li and J. Wu, Org. Lett., 2011, 13, 712. CrossRef
185. T. Fukutani, N. Umeda, K. Hirano, T. Satoh, and M. Miura, Chem. Commun., 2009, 5141. CrossRef
186. J. Jayakumar, K. Parthasarathy, and C.-H. Cheng, Angew. Chem. Int. Ed., 2012, 51, 197. CrossRef
187. J. Parthasarathy, N. Senthilkumar, J. Jayakumar, and C.-H. Cheng, Org. Lett., 2012, 14, 3478. CrossRef
188. H.-C. Ouyang, R.-Y. Tang, P. Zhong, X.-G. Zhang, and J.-H. Li, J. Org. Chem., 2011, 76, 223. CrossRef
189. a) Z. Chen, X. Yu, and J. Wu, Chem. Commun., 2010, 46, 6356; CrossRef b) X. Yu and J. Wu, J. Comb. Chem., 2010, 12, 238; CrossRef c) S. Ye and J. Wu, Tetrahedron Lett., 2009, 50, 6273; CrossRef d) H. Zhou, H. Jin, S. Ye, X. He, and J. Wu, Tetrahedron Lett., 2009, 50, 4616; CrossRef e) X. Yu, Q. Ding, Z. Chen, and J. Wu, Tetrahedron Lett., 2009, 50, 4279; CrossRef f) Q. Ding, Z. Wang, and J. Wu, Tetrahedron Lett., 2009, 50, 198; CrossRef g) S. Ye, H. Zhou, and J. Wu, Tetrahedron, 2009, 65, 1294; CrossRef h) Y. Ye, Q. Ding, and J. Wu, Tetrahedron, 2008, 64, 1378; CrossRef i) Q. Ding and J. Wu, Org. Lett., 2007, 9, 4959; CrossRef j) K. Gao and J. Wu, J. Org. Chem., 2007, 72, 8611; CrossRef k) Q. Ding, Y. Ye, R. Fan, and J. Wu, J. Org. Chem., 2007, 72, 5439; CrossRef l) W. Sun, Q. Ding, X. Sun, R. Fan, and J. Wu, J. Comb. Chem., 2007, 9, 690; CrossRef m) Q. Ding, B. Wang, and J. Wu, Tetrahedron, 2007, 63, 12166. CrossRef
190. H. Lou, S. Ye, J. Zhang, and J. Wu, Tetrahedron, 2011, 67, 2060. CrossRef
191. M. Bakherad, A. Keivanloo, and S. Jajarmi, Tetrahedron, 2012, 68, 2107. CrossRef
192. L. Zheng, J. Ju, Y. Bin, and R. Hua, J. Org. Chem., 2012, 77, 5794. CrossRef
193. B. Das, P. Jangili, J. Kashanna, and R. A. Kumar, Synthesis, 2011, 3267. CrossRef
194. Y. Ohta, S. Oishi, N. Fujii, and H. Ohno, Chem. Commun., 2008, 835. CrossRef
195. F. Sha and X. Huang, Angew. Chem., 2009, 121, 3510; CrossRef Angew. Chem. Int. Ed., 2009, 48, 3458. CrossRef
196. S. Ye and J. Wu, Tetrahedron Lett., 2009, 50, 6273. CrossRef
197. M. Yu, Y. Wang, C.-J. Li, and X. Yao, Tetrahedron Lett., 2009, 50, 6791. CrossRef
198. H. Zhou, H. Jin, S. Ye, X. He, and J. Wu, Tetrahedron Lett., 2009, 50, 4616. CrossRef
199. S. H. S. Azzam, A. Siddekh, and M. A. Pasha, Tetrahedron Lett., 2012, 53, 6306. CrossRef
200. M. Genelot, V. Dufaud, and L. Djakovitch, Tetrahedron, 2011, 67, 976. CrossRef
201. Z. Zheng and H. Alper, Org. Lett., 2008, 10, 4903. CrossRef
202. V. Tyagi, S. Khan, V. Bajpai, H. M. Gauniyal, B. Kumar, and P. M. S. Chauhan, J. Org. Chem., 2012, 77, 1414. CrossRef
203. K. Okuro and H. Alper, J. Org. Chem., 2012, 77, 4420. CrossRef
204. K. Okuro and H. Alper, Tetrahedron Lett., 2012, 53, 4816. CrossRef
205. M. P. Surpur, S. Kshirsagar, and S. D. Samant, Tetrahedron Lett., 2009, 50, 719. CrossRef
206. J. S. Yadav, B. V. Subha Reddy, S. K. Biswas, and S. Sengupta, Tetrahedron Lett., 2009, 50, 5798. CrossRef
207. B. V. Subba Reddy, M. Ramana Reddy, G. Narasimhulu, and J. S. Jadav, Tetrahedron Lett., 2010, 51, 5677. CrossRef
208. N. Seshu Babu, N. Pasha, K. T. V. Rao, P. S. S. Prasad, and N. Lingaiah, Tetrahedron Lett., 2008, 49, 2730. CrossRef
209. P. Bhattacharyya, K. Pradhan, S. Paul, and A. R. Das, Tetrahedron Lett., 2012, 53, 4687. CrossRef
210. B. Karmakar, A. Nayak, and J. Banerji, Tetrahedron Lett., 2012, 53, 5004. CrossRef
211. X.-N. Zhang, Y.-X. Li, and Z.-H. Zhang, Tetrahedron, 2011, 67, 7426. CrossRef
212. S. Samai, G. C. Nandi, and M. S. Singh, Tetrahedron, 2012, 68, 1247. CrossRef
213. G. Shanthi and P. T. Perumal, Tetrahedron Lett., 2007, 48, 6785. CrossRef
214. S. Paul, P. Bhattacharyya, and A. R. Das, Tetrahedron Lett., 2011, 52, 4636. CrossRef
215. G. C. Nandi, S. Samai, R. Kumar, and M. S. Singh, Tetrahedron, 2009, 65, 7129. CrossRef
216. Q. Zhang, H. Su, J. Luo, and Y. Wei, Green Chem., 2012, 14, 201. CrossRef
217. R. Kumar, K. Raghuvanshi, R. K. Verma, and M. S. Singh, Tetrahedron Lett., 2010, 51, 5933. CrossRef
218. J. S. Yadav, B. V. Subha Reddy, T. Maity, and G. G. K. S. N. Kumar, Tetrahedron Lett., 2007, 48, 8874. CrossRef
219. U. K. Roy, P. K. Jana, and S. Roy, Tetrahedron Lett., 2007, 48, 1183. CrossRef
220. Y. Li, Z. Yu, and H. Alper, Org. Lett., 2007, 9, 1647. CrossRef
221. W. Chen, Y. Cai, X. Fu, X. Liu, L. Lin, and X. Feng, Org. Lett., 2011, 13, 4910. CrossRef
222. W. H. dos Santos and L. C. da Silva-Filho, Synthesis, 2012, 3361. CrossRef
223. K. Palanichamy, S. R. Suravarapu, and K. P. Kaliappan, Synthesis, 2012, 1841. CrossRef
224. B. Willy and T. J. J. Muller, Synlett, 2009, 1255. CrossRef
225. W. Lu, W. Song, D. Hong, P. Lu, and Y. Wang, Adv. Synth. Catal., 2009, 351, 1768. CrossRef
226. T. Sasada, F. Kobayashi, N. Sakai, and T. Konakahara, Org. Lett., 2009, 11, 2161. CrossRef
227. H. Cao, X. Wang, H. Jiang, Q. Zhu, M. Zhang, and H. Liu, Chem. Eur. J., 2008, 14, 11623. CrossRef
228. A. Dandia, A. K. Jain, and S. Sharma, Tetrahedron Lett., 2012, 53, 5270. CrossRef
229. a) T. Vlaar, E. Ruijter, and R. V. A. Orru, Adv. Synth. Catal., 2011, 353, 809; CrossRef b) G. Van Baelen, S. Kuijer, L. Rycek, S. Sergeyev, E. Janssen, F. J. J. de Kanter, B. U. W. Maes, E. Ruijter, and R. V. A. Orru, Chem. Eur. J., 2011, 17, 15039. CrossRef
230. T. Vlaar, E. Ruijter, A. Znabet, E. Janssen, F. J. J. de Kanter, B. U. W. Maes, and R. V. A. Orru, Org. Lett., 2011, 13, 6496. CrossRef
231. C. Mukhopadhyay, S. Rana, and R. J. Butcher, Tetrahedron Lett., 2011, 52, 4153. CrossRef
232. S. Gupta, S. K. Sharma, A. K. Mandadapu, H. M. Gauniyal, and B. Kundu, Tetrahedron Lett., 2011, 52, 4288. CrossRef
233. K. K. Pasunooti, H. Chai, C. N. Jensen, B. K. Goritala, S. Wang, and X. W. Liu, Tetrahedron Lett., 2011, 52, 80. CrossRef
234. B. K. Banik, A. T. Reddy, A. Datta, and C. Mukhopadhyay, Tetrahedron Lett., 2007, 48, 7392. CrossRef
235. T. N. Glasnov, H. Tye, and C. O. Kappe, Tetrahedron, 2008, 64, 2035. CrossRef
236. P. Li, S. Regati, R. J. Butcher, H. D. Arman, Z. Chen, S. Xiang, B. Chen, and C.-G. Zhao, Tetrahedron Lett., 2011, 52, 6220. CrossRef
237. O. M. Singh and N. S. Devi, J. Org. Chem., 2009, 74, 3141. CrossRef
238. M. Zhang and H.-F. Jiang, Eur. J. Org. Chem., 2008, 3519. CrossRef
239. P. Mizar and B. Myrboh, Tetrahedron Lett., 2008, 49, 5283. CrossRef
240. R. N. Bhattacharya, P. Kundu, and G. Maiti, Tetrahedron Lett., 2011, 52, 26. CrossRef
241. a) C. S. Jang, F. Y. Fu, C. Y. Wang, K. C. Haung, G. Lu, and T. C. Thou, Science, 1946, 103, 59; CrossRef b) R. O. Dempcy and E. B. Skibo, Bioorg. Med. Chem. Lett., 1993, 1, 39; c) K. Tereshima, H. Shimamura, A. Kawase, Y. Tanaka, T. Tanimura, T. Kamisaki, Y. Ishizuka, and M. Sato, Chem. Pharm. Bull., 1995, 43, 2021; CrossRef d) N. J. Liverton, D. J. Armstrong, D. A. Claremon, D. C. Remy, J. J. Baldwin, R. J. Lynch, G. Zhang, and R. Gould, Bioorg. Med. Chem. Lett., 1998, 8, 484. CrossRef
242. a) S. Kobayashi, M. Ueno, R. Suzuki, and H. Ishitani, Tetrahedron Lett., 1999, 40, 2175; CrossRef b) S.-L. Cao, Y.-P. Feng, Y.-Y. Jiang, S.-Y. Liu, G.-Y. Ding, and R.-T. Li, Bioorg. Med. Chem. Lett., 2005, 15, 1915; CrossRef c) D. Gueyrard, V. Durnel, O. Leoni, S. Palmieri, and P. Rollin, Heterocycles, 2000, 52, 827; CrossRef d) B. Ana and S. Boteanu, Farmacia, 1971, 19, 683; e) T. A. Martin, A. G. Wheller, R. F. Majewski, and J. R. Corrigan, J. Med. Chem., 1964, 7, 812. CrossRef
243. a) K. Waisser, J. Gregor, H. Dostal, J. Kunes, L. Kubicova, V. Klimesova, and J. Kaustova, Farmaco, 2001, 56, 803; CrossRef b) J. Kunes, J. Bazant, M. Pour, K. Waisser, M. Slosarek, and J. Janota, Farmaco, 2000, 55, 725. CrossRef
244. a) G. Rabillound and B. Sillion, J. Heterocycl. Chem., 1980, 17, 1065; CrossRef b) M. Okabe and R.-C. Sun, Tetrahedron, 1995, 51, 1861; CrossRef c) R. J. Abdel-Jalil, W. Voelter, and M. Saeed, Tetrahedron Lett., 2004, 45, 3475; CrossRef d) S. Xue, J. McKenna, W.-C. Shineh, and O. Repic, J. Org. Chem., 2004, 69, 6474; CrossRef e) B. Das and J. Benerjee, Chem. Lett., 2004, 33, 960; CrossRef f) J.-F. Liu, J. Lee, A. M. Dalton, G. Bi, L. Yu, C. M. Baldino, E. McElory, and M. Brown, Tetrahedron Lett., 2005, 46, 1241. CrossRef
245. K. Okuro and H. Alper, Tetrahedron Lett., 2012, 53, 2540. CrossRef
246. H. Alper and Z. Zheng, Org. Lett., 2008, 10, 829. CrossRef
247. J. C. Chen, D. Wu, F. He, M. Liu, H. Wu, J. Ding, and W. Su, Tetrahedron Lett., 2008, 49, 3814. CrossRef
248. Y. Shen, C. Han, S. Cai, P. Lu, and Y. Wang, Tetrahedron Lett., 2012, 53, 5671. CrossRef
249. A. A. Mohammadi, M. Dabiri, and H. Qaraat, Tetrahedron, 2009, 65, 3804. CrossRef
250. M. M. Heravi, B. Baghernejad, and H. A. Oskooie, Tetrahedron Lett., 2009, 50, 767. CrossRef
251. X. Xu, L. Liang, J. Liu, J. Yang, L. Mai, and Y. Li, Tetrahedron Lett., 2009, 50, 57. CrossRef
252. T. Dahl, C. W. Tornoe, B. B. Andersen, P. Nielsen, and M. Jorgensen, Angew. Chem. Int. Ed., 2008, 47, 1726. CrossRef
253. H. Zuo, Z.-B. Li, F.-K. Ren, J. R. Falck, M. Lijuan, C. Ahn, and D.-S. Shin, Tetrahedron, 2008, 64, 9669. CrossRef
254. B. Groenendaal, D. J. Vugts, R. F. Schmitz, F. J. J. de Kanter, E. Ruijter, M. B. Groen, and R. V. A. Orru, J. Org. Chem., 2008, 73, 719. CrossRef
255. H. Zheng and D. G. Hall, Tetrahedron Lett., 2010, 51, 4256. CrossRef
256. a) U. Nubbemeyer, Synthesis, 2003, 961; CrossRef b) A. M. M. Castro, Chem. Rev., 2004, 104, 2939. CrossRef
257. C.-X. Yan, Z.-X. Sun, and Y. Cheng, Synthesis, 2012, 865. CrossRef
258. a) J. L. R. Martin, M. Sainz-Pardo, T. A. Furukawa, E. Martín-Sanchez, T. Seoane, and C. Galan, J. Psychopharmacol., 2007, 21, 774; CrossRef b) S. Chakraborty, N. H. Shah, J. C. Fishbein, and R. S. Hosmane, Bioorg. Med. Chem. Lett., 2011, 21, 756; CrossRef c) D. Antonow and D. E. Thurston, Chem. Rev., 2011, 111, 2815. CrossRef
259. a) S. Michelini, G. B. Cassano, F. Frare, and G. Perugi, Pharmacopsychiatry, 1996, 29, 127; CrossRef b) J. Knabe, H. P. Buech, and S. Bender, Arch. Pharm. (Weinhein), 1995, 328, 59; CrossRef c) R. N. Brogden, R. C. Heel, T. M. Speight, and G. S. Avery, Drugs, 1980, 20, 161; CrossRef d) K. S. Atwal, J. L. Bergey, A. Hedberg, and S. Moreland, J. Med. Chem., 1987, 30, 635; CrossRef e) V. Merluzzi, K. D. Hargrave, M. Labadia, K. Grozinger, M. Skoog, J. C. Wu, C.-K. Shih, K. Eckner, S. Hattox, J. Adams, A. S. Rosenthal, R. Faanes, R. J. Eckner, R. A. Koup, and J. L. Sullivan, Science, 1990, 250, 1411; CrossRef f) M. D. Braccio, G. Grossi, G. Roma, L. Vargiu, M. Mura, and M. E. Marongiu, Eur. J. Med. Chem., 2001, 36, 935; CrossRef g) K. A. Parker and C. A. Coburn, J. Org. Chem., 1992, 57, 97. CrossRef
260. A. Maleki, Tetrahedron, 2012, 68, 7827. CrossRef
261. a) B. Willy, T. Dallos, F. Rominger, J. Schönhaber, and T. J. J. Müller, Eur. J. Org. Chem., 2008, 4796; CrossRef b) S. S. Palimkar, R. J. Lahoti, and K. V. Srinivasan, Green Chem., 2007, 9, 146. CrossRef
262. M. Nardi, A. Cozza, A. De Nino, M. Oliverio, and A. Procopio, Synthesis, 2012, 800. CrossRef
263. I. R. Siddiqui, S. Shamim, D. K. Shireen, and M. A. Waseem, New J. Chem., 2012, 36, 2209. CrossRef
264. R. A. De Silva, S. Santra, and P. R. Andreana, Org. Lett., 2008, 10, 4541. CrossRef
265. C. Hudson, V. S. Murthy, K. G. Esetep, and G. Gustafson, Tetrahedron Lett., 2007, 48, 1489. CrossRef
266. X. Yi, B.-D. Barry, P. Liao, and X. Bi, Synthesis, 2012, 1323. CrossRef
267. J. Barluenga, A. Mendoza, F. Rodriguez, and F. J. Fananas, Angew. Chem., 2008, 120, 7152; CrossRef Angew. Chem. Int. Ed., 2008, 47, 7044. CrossRef
268. J. Barluenga, A. Mendoza, F. Rodríguez, and F. J. Fañanás, Angew. Chem., 2009, 121, 1672; CrossRef Angew. Chem. Int. Ed., 2009, 48, 1644. CrossRef
269. Y. Ohta, H. Chiba, S. Oishi, N. Fujii, and H. Ohno, Org. Lett., 2008, 10, 3535. CrossRef
270. Y. Ohta, S. Oishi, N. Fujii, and H. Ohno, Org. Lett., 2009, 11, 1979. CrossRef
271. Y. Ohta, Y. Kubota, T. Watabe, H. Chiba, S. Oishi, N. Fujii, and H. Ohno, J. Org. Chem., 2009, 74, 6299. CrossRef
272. F. Zeng and H. Alper, Org. Lett., 2010, 12, 3642. CrossRef
273. A. Kapur, K. Kumar, L. Singh, P. Singh, M. Elango, V. Subramanian, V. Gupta, P. Kanwal, and M. P. S. Ishar, Tetrahedron, 2009, 65, 4593. CrossRef
274. S. H. S. Azzam and M. A. Pasha, Tetrahedron Lett., 2012, 53, 6834. CrossRef
275. H. Mecadon, M. R. Rohman, M. Rajbangshi, and B. Myrboh, Tetrahedron Lett., 2011, 52, 2523. CrossRef
276. X. Yu, G. Qiu, J. Liu, and J. Wu, Synthesis, 2011, 2268. CrossRef
277. A. Zamudio-Medina, M. C. García-González, J. Padilla, and E. González-Zamora, Tetrahedron Lett., 2010, 51, 4837. CrossRef
278. A. Kumar, A. Saxena, M. Dewan, A. De, and S. Mozumdar, Tetrahedron Lett., 2011 52, 4835. CrossRef
279. E. Mosaddegh and A. Hassankhani, Tetrahedron Lett., 2011, 52, 488. CrossRef
280. S.-H. Song, J. Zhong, Y.-H. He, and Z. Guan, Tetrahedron Lett., 2012, 53, 7075. CrossRef
281. B. V. S. Reddy, C. Divyavani, Z. Begum, J. S. Yadav, and T. P. Rao, Synthesis, 2011, 168. CrossRef
282. J. Cheng, X. Jiang, and S. Ma, Org. Lett., 2011, 13, 5200. CrossRef
283. O. A. Attanasi, S. Bartoccini, G. Favi, G. Giorgi, F. R. Perrulli, and S. Santeusanio, J. Org. Chem., 2012, 77, 1161. CrossRef
284. R. Ghahremanzadeh, T. Amanpour, and A. Bazgir, Tetrahedron Lett., 2010, 51, 4202. CrossRef