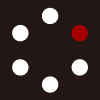
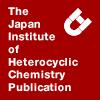
HETEROCYCLES
An International Journal for Reviews and Communications in Heterocyclic ChemistryWeb Edition ISSN: 1881-0942
Published online by The Japan Institute of Heterocyclic Chemistry
e-Journal
Full Text HTML
Received, 5th June, 2014, Accepted, 24th June, 2014, Published online, 7th July, 2014.
DOI: 10.3987/COM-14-S(K)36
■ Synthesis of Photoreactive Diazirinyl Salicin Derivative to Elucidate Functional Analysis of the Bitter Taste Receptor
Munenori Sakurai, Takuma Yoshida, Lei Wang, Yuta Murai, Katsuyoshi Masuda, Yasuko Sakihama, Yasuyuki Hashidoko, Yasumaru Hatanaka, and Makoto Hashimoto*
Division of Applied Science, Graduate School of Agriculture, Hokkaido University, Kita 9 Nishi 9, Kita-ku, Sapporo, Hokkaido 060-8589, Japan
Abstract
Salicin (salicyl alcohol glucoside) is a substance well known for its bitter taste. A photoreactive diazirinyl derivative of salicin will be utilized for the functional analysis of interactions between the bitter taste receptor and salicin. Glucosides of salicyl derivatives are more difficult than phenol derivatives that are unsubstituted at the ortho-position. A diazirinyl salicin derivative was synthesized at moderate yields by glucosidation of 2,3,4,6-tetra-O-acetyl-α-D-glucopyranosyl bromide and 2-hydroxy-4-[3-(trifluoromethyl)-3H-diazirin-3-yl]benzaldehyde in the presence of a phase-transfer catalyst, nBuEt3NBr, followed by reduction and deprotection.Salicin is long known as one of the major components of phenyl glycosides in the family Salicaceae1 and is a bitter anti-inflammatory compound.2 Elucidation of the functions of its gustatory receptor on the basis of structure–activity relationships may reveal the mechanism of homeostatic functions, which is of great interest to scientists. Photoaffinity labeling is one of the most common techniques used in the analysis of chemical biology.3 To the best of our knowledge, there are no reports on the preparation of photoreactive salicin derivatives for the analysis of bitter taste receptors. Two modifications of phenyl glucoside derivatives using diazirine have been reported. The derivatives are not substituted at the o-position of phenol. One modification is glucosidation of p-diazirinyl phenol to synthesize α-glucoside4 and the other is construction of the diazirine moiety in the precursor of the phenyl glucoside derivative.5 However, glucosides of salicyl alcohol derivatives are more complicated than glucosides of the o-position unsubstituted phenol derivatives.6 Optimization of glucosidation of salicyl alcohol or salicylaldehyde derivatives is essential to the synthesis of target diazirinyl derivatives of salicin. In this study, we report the synthesis of diazirinyl derivative and preliminary screening of glucosidations for salicyl alcohol and salicylaldehyde derivatives.
We conducted retrosynthesis of the diazirinyl salicin derivative 1. Salicyl alcohol derivatives (4 and 5) were subjected to glucosidation with a donor derivative (2 or 3), as shown in route a in Scheme 1. Although route a seems to be straightforward construction of the salicin skeleton, few studies have described glucosidations of salicyl alcohol derivatives.7 Another route involves glucosidation with salicyl- aldehyde derivatives (7) followed by reduction of the aldehyde group to primary alcohol (Scheme 1, route b). Although this route is an indirect method for constructing the target skeleton, others investigators used it in the glucosidation of salicylaldehyde due to more effective glucoside formation than using salicyl alcohol derivatives.6 But comprehensive comparison of glucosidation for salicyl derivatives has not been reported yet. Several glucosidation routes for 2 and 3 using salicyl alcohol and salicylaldehyde derivatives were screened as model reactions to synthesize the diazirinyl salicin derivative.
Glucosidation of 1,2,3,4,6-penta-O-acetyl-β-D-glucopyranose (2) and 4-acetoxymethylphenol with boron trifluoride etherate and triethylamine8 has been reported to result in good isolation yield. However, all salicyl derivatives (8–10, 1 - 1.5 eq), even though 2-acetoxymethylphenol (9), with boron trifluoride etherate (1.4 - 2.5 eq) and triethylamine (0.3 - 0.5 eq) did not yield a glucosidated product. These results indicated that the reactivity of 2-acetoxymethyl-substituted phenol was different from that of 4-acetoxymethyl-substituted phenol (Scheme 2). The ortho-substitution phenols inhibited the glucosidations of 2 with BF3 etherate.
Glucosidation of 2,3,4,6-tetra-O-acetyl-α-D-glucopyranosyl bromide (3) and phenol derivatives in the presence of phase-transfer catalysts is one of the mild reactions used for the construction of β-glucoside. The phase-transfer catalysts dimethylaminopyridine9 and benzyltriethylammonium bromide10 did not yield any glucosidation products (Scheme 3 entries 1 to 6). However, n-butyltriethylammonium bromide11 improved glucosidation with salicyl derivatives. Although salicyl alcohol derivatives (8 and 9) did not undergo glucosidation, salicylaldehyde (10) produced the glucoside, 11, at moderate yield (Scheme 3 entries 7 to 9). The anomeric proton coupling constant of compound 11 (J = 7.6 Hz) define the β-configuration. Hence, diazirinyl salicin derivatives were synthesized with diazirinyl salicylaldehyde derivative in the presence of the phase-transfer catalyst, n-BuEt3NBr.
Diazirinyl salicylaldehyde derivative 712 was subjected to glucosidation with an equivalent of 2,3,4,6-tetra-O-acetyl-α-D-glucopyranosyl bromide (3) and n-BuEt3NBr at rt to afford 12 at low yield (9%, Scheme 4 entry 1). 2,3,4,6-Tetra-O-acetyl-α-D-glucopyranosyl bromide (3) was completely consumed under these conditions. The isolated yield of 12 improved with 2 equivalents of 2,3,4,6-tetra-O-acetyl-α-D-glucopyranosyl bromide (3) and 2.5 equivalents of n-BuEt3NBr (Scheme 4 entries 2–4). The anomeric proton coupling constant of compound 12 (δ 5.18 ppm, J = 7.6 Hz) define the β-configuration.
The aldehyde in compound 12 was subjected to reduction to primary alcohol with NaBH4 (84%), followed by deacetylation with sodium methoxide (36%) to produce the diazirinyl salicin derivative, 1 (Scheme 5).
Compound 1 was subjected to a photoirradiation experiment with black light (100 W) to ensure its photoreactivity.13 Decay at approximately 360 nm was measured in a time-dependent manner (Scheme 6). Although higher reactivity of generated carbene afforded complex photolysis mixture, main product was identified as methanol adduct of 1 (FD-MS, m/z 398).14 The half-life of diazirinyl moiety on compound 1 was found to be 0.78 min by using a semilogarithmic plot. These results indicated that 1 had sufficient reactivity for photoaffinity labeling. Hence, further functional analysis of the bitter receptor is underway.
EXPERIMENTALS
Genaral methods. NMR spectra were measured by JEOL EX-270 and ECA-500 spectrometers. ESI-TOF-MS data were obtained with a Waters UPLC ESI-TOF mass spectrometer. Optical rotation data
were obtained with a JASCO DIP-370 polarimeter at 23 °C.
2-[(2,3,4,6-Tetra-O-acetyl-β-D-glucopyranosyl)oxy]benzaldehyde (11)15
Benzaldehyde 10 (0.1287 g, 1.05 mmol), 2,3,4,6-tetra-O-acetyl-α-D-glucopyranosyl bromide 3 (0.4811 g, 1.17 mmol) and n-BuEt3NBr (0.3888 g, 1.17 mmol) were dissolved in CH2Cl2 (10 mL). A solution of sodium hydroxide (5%, 4 mL) was added to the above solution. The reaction mixture was stirred at rt for 20 h virgously and diluted with CHCl3. The diluted solution was washed with cold 1 M HCl solution and brine, dried over MgSO4, and concentrated. The residue was purified by silica gel column chromatography (hexane / AcOEt = 3 / 1) to afford 11 (0.2185 g, 46%) as colorless solid. 1H-NMR (CDCl3) δ: 10.35 (1H, s), 7.87 (1H, dd, J = 7.7, 1.8 Hz), 7.56 (1H, ddd, J = 8.7, 7.0, 1.4 Hz), 7.19 (1H, t, J = 7.6 Hz), 7.12 (1H, d, J = 8.2 Hz), 5.41 - 5.30 (2H, m), 5.23 - 5.16 (1H, m), 5.19 (1H, d, J = 7.6 Hz), 4.30 (1H, dd, J = 12.5, 5.3 Hz), 4.18 (1H, dd, J = 12.4, 2.5 Hz), 3.90 (1H, ddd, J = 10.1, 5.2, 2.5 Hz), 2.08 (1H, s), 2.07 (1H, s), 2.06 (1H, s), 2.05 (1H, s); 13C-NMR (CDCl3) δ: 189.06, 170.43, 170.12, 169.30, 169.14, 158.74, 135.64, 128.31, 126.28, 123.61, 116.00, 99.07, 72.23, 70.92, 68.18, 61.76, 20.53; [α]D -29.0 (c 1.0, CHCl3).
2-[(2,3,4,6-Tetra-O-acetyl-β-D-glucopyranosyl)oxy]-4-[3-(trifluoromethyl)-3H-diazirine-3-yl]benzaldehyde (12)
Diazirinylbenzaldehyde 7 (0.0553 g, 0.24 mmol), 2,3,4,6-tetra-O-acetyl-α-D-glucopyranosyl bromide 3 (0.2862 g, 0.48 mmol), and n-BuEt3NBr (0.1938 g, 0.60 mmol) were dissolved in CH2Cl2 (4 mL). A solution of sodium hydroxide (5%, 4 mL) was added. The reaction mixture was stirred at rt for 21 h and diluted with CHCl3. The diluted solution was washed with cold 1 M HCl solution and brine, dried over MgSO4, and concentrated. The residue was purified by silica gel column chromatography (hexane / AcOEt = 3 / 1 to hexane / AcOEt = 1 / 1) to afford 12 (0.0875 g, 65%) as colrless solid. 1H-NMR (CDCl3) δ: 10.30 (1H, s), 7.88 (1H, d, J = 8.2 Hz), 6.97 (1H, d, J = 8.9 Hz), 6.94 (1H, s), 5.41-5.30 (2H, m), 5.23-5.13 (1H, m), 5.18 (1H, d, J = 7.6 Hz), 4.27 (1H, dd, J = 5.3, 12.5Hz), 4.20 (1H, dd, J = 2.6, 12.5 Hz), 3.95 (1H, ddd, J = 2.6, 5.3, 9.9 Hz), 2.13 (3H, s), 2.07 (3H, s), 2.06 (3H, s), 2.05 (3H, s). 13C-NMR (CDCl3) δ: 187.99, 170.59, 170.07, 169.33, 169.13, 158.41, 136.54, 128.96, 126.55, 121.68 (q, 1JCF = 274.9 Hz), 121.24, 113.92, 98.88, 72.59, 72.17, 70.68, 67.92, 61.87, 28.45 (q, 2JCF = 39.1 Hz), 20.55. 19F-NMR(CDCl3) δ:-64.57. [α]D +7.3 (c 1.0, MeOH). HRMS-FD (m/z) M+ calcd for C23H23F3N2O11 560.1254, found 560.1238.
2-[(2,3,4,6-Tetra-O-acetyl-β-D-glucopyranosyl)oxy]-4-[3-(trifluoromethyl)-3H-diazirine-3-yl]benzyl alcohol (13)
The diazirinyl tetraacetylphenylglucoside derivative 12 (0.0673 g, 0.12 mmol) was dissolved in MeOH and cooled to 0 ℃ and NaBH4 (0.0054 g, 0.14 mmol) was added to the reaction mixture. The reaction mixture was stirred at rt for 4 h. After acidification of the solution with 1 M HCl at 0 ℃ the reaction mixture was extracted with CH2Cl2. The organic solution was dried over MgSO4, filtrated, and concentrated. The residue was purified by silica gel column chromatography (hexane / AcOEt = 1 / 1) to afford diazirinyl tetraacetylsalicin derivative 13 (0.0567 g, 84%) as colorless solid. 1H-NMR (CDCl3) δ: 7.40 (1H, d, J = 7.9 Hz), 6.92 (1H, d, J = 7.9 Hz), 6.84 (1H, s), 5.34 (1H, t, J = 5.9 Hz), 5.33 (1H, dd, J = 13.4, 17.3 Hz), 5.13 (2H, dd, J = 8.2, 17.8 Hz), 4.65 (1H, d, J = 13.5 Hz), 4.56 (1H, d, J = 13.2 Hz), 4.23 (1H, d, J = 5.6 Hz), 4.21 (1H, d, J = 2.6 Hz), 3.91 (1H, dq, J = 2.6, 9.7 Hz), 2,37 (1H, br s), 2.11 (3H, s), 2.10 (3H, s), 2.07 (3H, s), 2.05 (3H, s). 13C-NMR (CDCl3) δ: 170.66, 170.06, 169.68, 169.39, 154.58, 132.94, 129.88, 129.72, 121.99(q, 1JCF = 274.3 Hz), 121.71, 113.26, 99.16, 72.41, 72.21, 70.97, 68.10, 61.90, 60.36, 28.30 (q, 2JCF = 40.2 Hz), 20.63, 20.55. 19F-NMR(CDCl3) δ: -65.06. [α]D -2.4 (c 1.0, MeOH). HRMS-FD (m/z) M+ calcd for C23H25F3N2O11 562.1410, found 562.1410.
2-Hydroxymethyl-5-[3-(trifluoromethyl)-3H-diazirin-3-yl]phenyl-β-D-glucopyranoside (1)
To a solution of diazirinyl pentaacetylsalicin derivative 13 (47.5 mg, 0.084 mmol) in MeOH (2 mL), a solution of NaOMe in MeOH (28%, 25 μL) was added at 0 ℃, and the reaction mixture was stirred at rt for 1 h. After the reaction, the mixture was concentrated in vacuo. The residue was purified by silica gel column chromatography (CH2Cl2 / MeOH = 4 / 1) to afford diazirinyl salicin 1 (0.0120 g, 36%) as colorless solid. 1H-NMR (CD3OD) δ: 7.38 (1H, d, J = 8.2 Hz), 6.95 (1H, s), 6.84 (1H, d, J = 7.9 Hz), 4.76-4.73 (1H, d), 4.67 (1H, d, J = 14.2 Hz), 4.52 (1H, d, J = 13.8 Hz), 3.79 (1H, d, J = 11.9 Hz), 3.62 (1H, dd, J = 3.1, 12.4 Hz), 3.43-3.33 (4H, m), 1.19 (1 H, br s). 13C-NMR (CD3OD), δ: 156.86, 134.82, 130.25, 129.92, 123.59 (q, 1JCF = 274.9 Hz), 121.77, 115.01, 103.26, 78.32, 77.96, 74.86, 71.19, 62.30, 60.10, 29.45 (q, 2JCF = 40.2 Hz). 19F-NMR(CD3OD) δ: -66.89. [α]D -23.1 (c 1.0, MeOH). HRMS-FD (m/z) M+ calcd for C15H17F3N2O7 394.0988, found 394.1002.
Photolysis of compound 1 in MeOH
A 1 mM degassed methanolic solution of the diazirinyl salicin 1 was placed in a quartz cuvette. Photolysis was carried out with 100 W black-light at a distance of 5cm from surface of light source. Spectra were measured after each minute, and then the half-life was caluculated from the decrements of the absorbance around 360 nm.
ACKNOWLEDGEMENTS
MH thanks the Fugaku Foundation for financial support. Part of this work was performed under the Cooperative Research Program of “Network Joint Research Center for Materials and Devices”.
References
1. G. A. Boeckler, J. Gershenzon, and S. B. Unsicker, Phytochemistry, 2011, 72, 1497. CrossRef
2. B. Bufe, T. Hofmann, D. Krautwurst, J.-D. Raguse, and W. Meyerhof, Nat. Genet., 2002, 32, 397. CrossRef
3. a) J. Brunner, Annu. Rev. Biochem., 1993, 62, 483; CrossRef b) Y. Hatanaka, H. Nakayama, and Y. Kanaoka, Rev. Heteroatom Chem., 1996, 14, 213; c) G. Dormán and G. D. Prestwich, Trends Biotechnol., 2000, 18, 64; CrossRef d) T. Tomohiro, M. Hashimoto, and Y. Hatanaka, Chem. Records, 2005, 5, 385; CrossRef e) M. Hashimoto and Y. Hatanaka, Eur. J. Org. Chem., 2008, 2513; CrossRef f) L. Dubinsky, B. P. Krom, and M. M. Meijler, Bioorg. Med. Chem., 2012, 20, 554. CrossRef
4. Y. Ambroise, C. Mioskowski, G. Leblanc, and B. Rousseau, Bioorg. Med. Chem. Lett., 2000, 10, 1125. CrossRef
5. N. K. Tyagi and R. K. H. Kinne, Anal. Biochem., 2003, 323, 74. CrossRef
6. M. L. Belyanin, E. V. Stepanova, and V. D. Ogorodnikov, Carbohydr. Res., 2012, 363, 66. CrossRef
7. a) K. Kinomura and T. Sakakibara, Jpn. Kokai Tokkyo Koho (1989), JP 01249796 A; b) J. Huang, Org. Chem. Ind. J., 2011, 7, 30.
8. Y. S. Lee, E. S. Rho, Y. K. Min, B. T. Kim, and K. H. Kim, J. Carbohydr. Chem., 2001, 20, 503. CrossRef
9. J.-N. Xiang, L.-H. Jiang, C.-Y. Chen, Z.-y. Fu, and J.-F. Duan, J. Carbohydr. Chem., 2006, 25, 595. CrossRef
10. H. P. Kleine, D. V. Weinberg, R. J. Kaufman, and R. S. Sidhu, Carbohydr. Res., 1985, 142, 333. CrossRef
11. C.-Y. Chen, J.-G. Sun, F.-Y. Liu, K.-P. Fung, P. Wu, and Z.-Z. Huang, Tetrahedron, 2012, 68, 2598. CrossRef
12. a) M. Hashimoto, Y. Hatanaka, and K. Nabeta, Bioorg. Med. Chem. Lett., 2000, 10, 2481; CrossRef b) M. Hashimoto, Y. Hatanaka, J. Yang, J. Dhesi, and G. D. Holman, Carbohydr. Res., 2001, 331, 119; CrossRef c) M. Hashimoto, K. Nabeta, and K. Murakami, Bioorg. Med. Chem. Lett., 2003, 13, 1531. CrossRef
13. M. Hashimoto and Y. Hatanaka, Anal. Biochem., 2006, 348, 154. CrossRef
14. Y. Murai, L. Wang, Y. Muto, Y. Sakihama, Y. Hashidoko, Y. Hatanaka, and M. Hashimoto, Heterocycles, 2013, 87, 2119. CrossRef
15. T. Storr, L. E. Scott, M. L. Bowen, D. E. Green, K. H. Thompson, H. J. Schugar, and C. Orvig, Dalton Trans., 2009, 3034. CrossRef