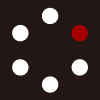
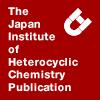
HETEROCYCLES
An International Journal for Reviews and Communications in Heterocyclic ChemistryWeb Edition ISSN: 1881-0942
Published online by The Japan Institute of Heterocyclic Chemistry
e-Journal
Full Text HTML
Received, 7th October, 2014, Accepted, 28th October, 2014, Published online, 5th November, 2014.
DOI: 10.3987/COM-14-13102
■ Synthesis of 2-Aryl- and 6-Heteroaryl-1,3-di(4-pyridyl)azulenes by Katritzky’s Pyridylation of 2-Aryl- and 6-Heteroarylazulenes
Taku Shoji,* Akifumi Maruyama, Shunji Ito, Tetsuo Okujima, Masafumi Yasunami, Junya Higashi, and Noboru Morita
Department of Chemistry, Faculty of Science, Shinshu University, Asahi 3-1-1, Matsumoto, Nagano 390-8621, Japan
Abstract
Preparation of 2-aryl- and 6-heteroarylazulenes 2–6 and 8–10 was established by the palladium-catalyzed cross-coupling reaction of the corresponding haloazulenes with lithium aryl- and heteroarylmagnesium ate complexes, which were readily prepared from the corresponding aryl and heteroaryl halides. The reaction of 2–6 and 8–10, except for 3 and 8, with pyridine in the presence of Tf2O, followed by treatment with KOH in MeOH afforded the corresponding 2-aryl- and 6-heteroaryl-1,3-di(4-pyridyl)azulenes 11–16 in good yields.INTRODUCTION
Azulene (C10H8) has attracted the interest of many research groups owing to its unusual properties as well as its beautiful blue color.1 To construct the electrochromic materials, we have developed the synthetic procedures for several 2- and 6-arylazulene derivatives by utilizing transition-metal-catalyzed cross-coupling reactions (e.g., Suzuki–Miyaura and Stille cross-coupling reactions) of 2- and 6-azulenyl metal reagents.2 Although the azulenyl metal reagents might be useful for the preparation of polyaromatic systems with multiple 2- and 6-azulenyl groups, preparation of the metal reagents for the transition-metal-catalyzed reactions sometimes causes difficulty and the most promising azulenylborane reagents are relatively unstable and undergo easy hydrolysis to afford hydrocarbon derivatives.2,3 As an another subject of the procedures, instability of 1- and/or 3-haloazulenes, the promising precursors for the functionalization at the 1- and/or 3-positions of azulenes by the transition-metal-catalyzed reactions, sometimes causes problems for the synthesis of 1- and/or 3-arylazulenes by such a method. For the reasons described above, development of a general and an efficient method for the preparation of functionalized azulene derivatives with multiple aryl and/or heteroaryl groups from readily available reagents would have great importance because functionalized azulene derivatives should become a promising candidate for the production of advanced materials.2−5
Herein, we report the details of the palladium-catalyzed cross-coupling reaction of 2- and 6-haloazulenes with aryl- and heteroarylmagnesium lithium ate complexes (lithium aryl- and heteroarylmagnesate reagents), which were prepared in situ from the corresponding aryl and heteroaryl halides, to afford 2-aryl- and 6-heteroarylazulenes.6 Furthermore, we also describe the synthesis of 2-aryl- and 6-heteroaryl-1,3-di(4-pyridyl)azulenes by Katritzky’s pyridylation reaction of 2-aryl- and 6-heteroarylazulenes with pyridine in the presence of trifluoromethanesulfonic anhydride (Tf2O), followed by treatment with KOH in MeOH. Characteristic properties of the 2-aryl- and 6-heteroaryl-1,3-di(4-pyridyl)azulenes were also clarified by NMR, absorption spectroscopy, and theoretical calculations.
RESULT AND DISCUSSION
SYNTHESIS: In 2003, Mongin et al. reported a one-pot procedure for the preparation of quinolinyl magnesium lithium ate complexes by a halogen-metal exchange reaction of bromoquinolines, followed by subsequent palladium-catalyzed cross-coupling of the ate complexes with heteroaryl halides to give 2-, 3-, and 4-heteroarylquinolines.7 Previously, we have also reported the preparation of azulenyllithium and -magnesium reagents utilizing halogen–metal exchange reaction and their reactivity toward several electrophiles have been clarified.3,8 Thus, the lithium aryl- and heteroarylmagnesate reagents were prepared in situ under the similar reaction conditions reported by literatures to examine the reactivity of the 2- and 6-haloazulenes with the reagents under the palladium-catalyzed cross-coupling conditions.3,6−8
To a solution of n-butylmagnesium chloride (n-BuMgCl) in diethyl ether was added n-butyllithium (n-BuLi) in hexane at 0 °C to give a lithium tri(n-butyl)magnesate reagent.9 After the mixture was stirred at the same temperature for 30 min, bromobenzene was added dropwise to the mixture at the same temperature. Then, to the cooled mixture a solution of 2-iodoazulene (1) and 5 mol% of PdCl2(PPh3)2 in diethyl ether was added dropwise at 0 °C (Scheme 1).10 The mixture was warmed to room temperature and stirred for 24 h to complete the cross-coupling reaction. The crude product was isolated and purified by the usual workup procedure to afford 2-phenylazulene (2)11 in 89% yield (Table 1, Entry 1).
To examine the generality of the reaction, the cross-coupling reaction of 1 with several aryl and heteroaryl halides was conducted under the similar reaction conditions. The magnesate reagents prepared from 2- and 3-bromopyridines were reacted readily with 1 to afford cross-coupling products 312 (99%) and 412 (72%), respectively (Table 1, Entries 2 and 3). The 2-(3-quinolyl)azulene (5) was also obtained in high yield (80%) by the reaction of 1 with 3-bromoquinoline under the similar reaction conditions as summarized in Table 1 (Entry 4). The magnesate reagent prepared from 1 was also reacted with 1 to give 2,2’-biazulene (6)13 in 71% yield, as less soluble green crystals (Table 1, Entry 5).
The cross-coupling reaction of 6-bromoazulene (7) with heteroarylmagnesate reagents was also conducted under the similar reaction conditions employed for the cross-coupling reaction of 1 (Scheme 2). The cross-coupling product, 6-(2-pyridyl)azulene (8) was obtained by the reaction of 7 with 2-pyridylmagnesate reagent in 93% yield (Table 2, Entry 1). The magnesate reagents prepared from 3-bromopyridine and 3-bromoquinoline were readily reacted with 7 to afford the corresponding cross-coupling products 9 and 10 in 70% and 80% yields, respectively (Table 2, Entries 2 and 3).
In 2001, Katritzky et al. have discovered the reaction of carbonyl compounds with N-[(trifluoromethyl)sulfonyl]pyridinium trifluoromethanesulfonate (TPT), followed by the base induced aromatization, to give the corresponding 4-(2-oxoalkyl)pyridine derivatives.14 Corey and Tian have extended the reaction of the electron-rich aromatic compound with pyridine derivatives in the presence of Tf2O to give the corresponding 4-aryldihydropyridines, which could be convertible to the corresponding 4-arylpyridines.15 We have applied the procedure to the heteroarylation of azulene derivatives at 1-, 2-, 3-, 5-, and 7-positions.16 The methodology using the triflate of nitrogen-containing heterocycles opened a new two-step strategy for the heteroarylation of aliphatic and aromatic compounds. In this study, we have applied the synthetic procedure of 1,3-di(4-pyridyl)azulenes via 1,3-bis(1,4-dihydropyrid-4-yl)azulenes to the synthesis of 2-aryl- and 6-heteroaryl-1,3-di(4-pyridyl)azulenes (Scheme 3). The results on the preparation of 2-aryl- and 6-heteroaryl-1,3-di(4-pyridyl)azulenes 11–16 by utilizing the synthetic procedure are summarized in Table 3. The reaction of 2 with pyridine in the presence of 2.4 equiv. Tf2O, followed by the treatment of KOH in MeOH without an isolation of the intermediately produced product, 1,3-bis(1,4-dihydropyrid-4-yl)-2-phenylazulene, gave 11 in 77% yield (Table 3, Entry 1).
A series of 2-heteroaryl- and 6-heteroarylazulenes 3−6 and 8−10 were subjected to the sequential electrophilic aromatic substitution reaction under the similar reaction conditions to explore the scope of the methodology. The synthesis of 12 and 13 were established by the reaction of 4 and 5 with pyridine in the presence of Tf2O, similar to the procedure described above in 81% and 80% yields, respectively, after the treatment of KOH in MeOH (Table 3, Entries 3 and 4). 1,1’,3,3’-Tetra(4-pyridyl)-2,2’-biazulene (14) was obtained in 72% yield, by the reaction of 6 with pyridine in the presence of 6-fold molar excess Tf2O, followed by the base-induced aromatization (Entry 5). Although the compound 6 is less soluble in the common organic solvent (e.g., CH2Cl2, CHCl3 etc.) as also shown in the literature, tetrapyridyl product 14 possesses relatively high solubility. It should be concluded to the steric effect of substituted pyridine moieties that inhibit the intermolecular π−π stacking, effectively.
The similar reaction of 6-heteroarylazulenes 8−10 with triflate of pyridine was also investigated to introduce the pyridine moieties into the azulene ring. The reaction of 9 and 10 with pyridine in the presence of Tf2O, followed by treatment with KOH in MeOH afforded 15 and 16 in 82% and 75% yields, respectively (Table 3, Entries 7 and 8). However, the sequential electropholic-aromatic substitution reaction of 3 and 8, having a 2-pyridyl moiety, did not afford the presumed 2-(2-pyridyl)- and 6-(2-pyridyl)-1,3-di(4-pyridyl)azulenes owing to the decomposition of the substrates under the reaction conditions (Table 3, Entries 2 and 6).
SPECTROSCOPIC PROPERTIES: All new compounds reported herein were fully characterized by spectroscopic data as shown in the Experimental Section. The high resolution mass spectra of all compounds ionized by FAB or ESI showed the correct molecular ion peaks. These results are consistent with the structures of these products. The 1H NMR spectra of 12 were measured both in acetone-d6 and in 10% CF3CO2D/acetone-d6. The spectra of 12 are represented in Figure 1.17 Most of the proton signals for the pyridine moieties of 12 were observed in downfield regions in 10% CF3CO2D/acetone-d6 relative to those in pure acetone-d6, which should be consistent with the generation of 12a3+ by the protonation of all nitrogen atoms in the pyridine moieties on the azulene ring (Scheme 4). Significant shifts for the ring protons of azulene moiety were also observed on the 1H NMR spectra, when the solvent was changed from acetone-d6 to 10% CF3CO2D/acetone-d6. These results should be attributable to the contribution of the tropylium cationic form 12b3+ in Scheme 4 by the resonance effect between azulene and pyridinium moieties.
The absorption maxima and their coefficients in the UV-Vis spectra for the 2-aryl- and 6-heteroaryl-1,3-di(4-pyridyl)azulenes 11−16 are shown in the Experimental Section. The UV-Vis spectra of 12 in CH2Cl2 and in 10% CF3CO2H/CH2Cl2 are shown in Figure 2. The 2-aryl- and 6-heteroaryl-1,3-di(4-pyridyl)azulenes in CH2Cl2 showed characteristic weak absorption bands in the visible region arising from the azulene system, as well as those of 2-(2- and 3-pyridyl)azulenes 3 and 4 reported by Wakabayashi et al. The UV-Vis spectra of 12 showed weak absorption bands at λmax = 368 nm sh (log ε = 3.86) and λmax = 583 nm (log ε = 2.62) in CH2Cl2. When the solvent was changed to 10% CF3CO2H/CH2Cl2, the longest wavelength absorption band exhibited a hypsochromic shift [λmax = 401 nm (log ε = 4.04) and λmax = 554 nm (log ε = 2.90)] relative to that in CH2Cl2. The blue-shift should be concluded to the formation of tricationic spices 12a3+, that could be drawn as a tropylium cationic form 12b3+ by the protonation of the pyridine nitrogen as illustrated in Scheme 4.
To elucidate the nature of the absorption bands of 12 and protonated species 12a3+, theoretical calculations were carried out on time-dependent density functional theory (TD-DFT) calculations at the B3LYP/6-31G** level.18 The frontier Kohn-Sham orbitals of 12 and 12a3+ are shown in Figures 3 and 4. Judging from a comparison between the experimental and the theoretical UV-Vis spectra, the absorption maxima of 12 at λmax = 583 nm should be considered as the π–π* transition
(HOMO to LUMO) of the substituted azulene-ring itself. The absorption maxima at λmax = 368 nm (sh) is also assigned to the transitions, which originated from the HOMO−1 delocalized on the both azulene and 3-pyridyl moiety at the 2-position to the LUMO located on the azulenyl group (Figure 3). Although the HOMO−1 and HOMO are slightly distributed over two 4-pyridyl groups at the 1,3-positions, contribution to the transitions is relatively small.
Theoretical calculations of 12a3+ showed the absorption band at λmax = 554 nm can be considered as the transition, which originated from the HOMO located on the azulenyl group to the LUMO mainly located on the pyridinium moiety at the 2-position (Figure 4 and Table 4). The broad absorption of 12a3+ at around λmax = 400 nm confirmed that the absorption band arose from the overlapping of HOMO to LUMO+1, LUMO+2, LUMO+3, and LUMO+4. Therefore, the blue-shift of the longest wavelength absorption band in 12a3+ should be concluded to the CT transition from azulene to three pyridinium groups.
Similar color changes were also observed for the other 2-aryl- and 6-heteroaryl-1,3-di(4-pyridyl)azulenes upon changing the solvent from CH2Cl2 to 10% CF3CO2H/CH2Cl2. The longest wavelength absorption band of compound 11 showed a hypsochromic shift in 10% CF3CO2H/CH2Cl2 (λmax = 546 nm) due to the protonation of the two pyridine moiety, compared to that in CH2Cl2 (λmax = 583 nm). When the UV-Vis spectra were measured in CH2Cl2, compound 13 exhibited absorption band at λmax = 585 nm. In contrast, the same compound showed a hypsochromic shift of the absorption band to λmax = 556 nm in 10% CF3CO2H/CH2Cl2, arising from the protonation of both quinoline and pyridine moieties. The longest wavelength absorption band of 2,2'-biazulene derivative 14 in 10% CF3CO2H/CH2Cl2 (λmax = 573 nm) showed a hypsochromic shift compared with that in CH2Cl2 (λmax = 595 nm). Compounds 15 and 16 also showed a hypsochromic shift (15: 616 nm; 16: 606 nm) in 10% CF3CO2H/CH2Cl2 due to the protonation of the pyridine and quinoline moieties, respectively, compared with those in CH2Cl2 (15: 562 nm; 16: 551 nm).
In conclusion, we have described an efficient synthetic method for 2-aryl- and 6-heteroarylazulenes. 2-Iodo- and 6-bromoazulenes 1 and 7 reacted with aryl- and heteroarylmagnesate regents in the presence of a palladium catalyst to afford the corresponding 2-aryl and 6-heteroarylazulenes 2–6 and 8–10 under mild reaction conditions. Several 2-aryl- and 6-heteroaryl-1,3-di(4-pyridyl)azulenes 11–16 were also prepared by utilizing Katritzky’s procedure consisting of the electrophilic substitution reaction of the 2-aryl- and 6-heteroarylazulenes with the triflate of pyridine, followed by the base-induced aromatization of the initially formed dihydropyridine derivatives. The longest wavelength absorption band on UV-Vis spectra of the 2-aryl- and 6-heteroaryl-1,3-di(4-pyridyl)azulenes 11–16 in 10% CF3CO2H/CH2Cl2 exhibited a hyspochromic shift, relative to that in CH2Cl2 due to the protonation of substituted-heterocycles to develop the intramolecular CT absorption bands.
Nitrogen-containing heterocyclics act as a highly versatile functional group in organic synthesis. Thus, there are a large number of reports for the synthesis of functional molecules, starting from nitrogen-containing heterocycles to date. Recently, we have also reported the synthesis of azulene-substituted polymethines,19 bi- and terazulene derivatives,20 which exhibit significant solvatochromism and intramolecular CT, from 1-(4-pyridyl)- and 1,3-di(4-pyridyl)azulenes. Thus, 2-aryl- and 6-heteroaryl-1,3-di(4-pyridyl)azulenes reported in this paper might be convertible to functionalized azulene derivatives.
To evaluate the scope of this class of molecules investigated by this research, molecular transformation of the reported compounds is currently under investigation in our laboratory.
EXPERIMENTAL
Melting points were determined with a Yanagimoto MPS3 micro melting apparatus and are uncorrected. Mass spectra were obtained with a Bruker APEX II instrument. IR and UV-Vis spectra were measured with JASCO FT/IR-4100 and Shimadzu UV-2550 spectrophotometers, respectively. 1H and 13C NMR spectra were recorded with a Bruker AVANCE400 spectrometer (at 400 MHz and 100 MHz, respectively), or a JEOL ECA500 spectrometer (at 500 MHz and 125 MHz, respectively). Elemental analyses were performed at the Research and Analytical Center for Giant Molecules, Graduate School of Science, Tohoku University.
General procedure for the synthesis of 2-aryl- and 6-heteroarylazulenes
To a solution of n-BuMgCl (0.9 M in THF, 1.4 mL) in Et2O (10 mL) was added n-BuLi (1.6 M in hexane, 1.5 mL) at 0 °C. After the mixture was stirred at the same temperature for 30 min, aryl or heteroaryl halides (1.10 mmol) was added dropwise. The resulting mixture was stirred at the same temperature for 1 h. To the mixture was added dropwise a solution of 1 or 7 (1.00 mmol) and PdCl2(PPh3)2 (35 mg, 0.05 mmol) in Et2O (20 mL) at 0 °C, and the mixture was stirred at room temperature for 24 h. The reaction mixture was poured into water and extracted with hexane. The organic layer was washed with brine, dried with Na2SO4, and concentrated under reduced pressure. The residue was purified by column chromatography on alumina with CH2Cl2 to give the corresponding products. Yield of the products is summarized in Tables 1 and 2.
2-Phenylazulene (2)11: mp 228.0−230 °C (lit. 231−232 °C); 1H NMR (500 MHz, CDCl3): δH = 8.29 (d, 2H, J = 10.0 Hz, 4,8-H), 7.96 (d, 2H, J = 7.3 Hz, H of o-Ph), 7.68 (s, 2H, 1,3-H), 7.51 (t, 1H, J = 10.0 Hz, 6-H), 7.46 (dd, 2H, J = 7.3, 7.3 Hz, H of m-Ph), 7.46 (dd, 1H, J = 7.3 Hz, H of p-Ph), 7.16 (t, 2H, J = 10.0 Hz, 5,7-H) ppm.
2-(2-Pyridyl)azulene (3)12: mp 114.5−115.0 °C (CH2Cl2/MeOH, lit. 116−118 °C); 1H NMR (400 MHz, CDCl3): δH = 8.74 (d, 1H, J = 4.8 Hz, 6’-H of Py), 8.34 (d, 2H, J = 10.0 Hz, 4,8-H), 7.99 (dd, 1H, J = 4.8, 1.2 Hz, 3’-H of Py), 7.97 (s, 2H, 1,3-H), 7.48 (dd, 1H, J = 4.8, 1.2 Hz, 5’-H of Py), 7.41 (t, 1H, J = 10.0 Hz, 6-H), 7.22 (dd, 1H, J = 4.8, 1.2 Hz, 4’-H of Py), 7.16 (t, 2H, J = 10.0 Hz, 5,7-H) ppm.
2-(3-Pyridyl)azulene (4)12: mp 180.0−182.0 °C, decomp. (lit. 182−183 °C); 1H NMR (400 MHz, CDCl3): δH = 9.21 (d, 1H, J = 1.6 Hz, 2’-H of Py), 8.57 (dd, 1H, J = 4.8, 1.6 Hz, 6’-H of Py), 8.32 (d, 2H, J = 10.0 Hz, 4,8-H), 8.18 (dd, 1H, J = 4.8, 1.6 Hz, 5’-H of Py), 7.67 (s, 2H, 1,3-H), 7.56 (t, 1H, J = 10.0 Hz, 6-H), 7.37 (dd, 1H, J = 4.8, 1.6 Hz, 4’-H of Py), 7.19 (t, 2H, J = 10.0 Hz, 5,7-H) ppm.
2-(3-Quinolyl)azulene (5): mp 184.0−185.0 °C (CH2Cl2/MeOH); IR (KBr): νmax = 3006 (m), 1574 (m), 1531 (m), 1504 (m), 1495 (m), 1469 (m), 1406 (m), 1350 (m), 1207 (w), 1020 (w), 951 (m), 906 (m), 812 (s), 729 (m), 669 (w), 578 (w), 474 (w) cm−1; UV-Vis (CH2Cl2): λmax (log ε) = 286 (4.68), 308 sh (4.79), 316 (4.83), 380 (4.32), 397 (4.32), 575 (2.66), 618 (2.64), 676 sh (2.29) nm; 1H NMR (400 MHz, CDCl3): δH = 9.53 (d, 1H, J = 1.6 Hz, 2’-H of Quinoline), 8.62 (d, 1H, J = 1.6 Hz, 4’-H of Quinoline), 8.34 (d, 2H, J = 10.0 Hz, 4,8-H), 8.12 (d, 1H, J = 8.4 Hz, 8’-H of Quinoline), 7.90 (d, 1H, J = 8.4 Hz, 5’-H of Quinoline), 7.80 (s, 2H, 1,3-H), 7.69 (dd, 1H, J = 8.4, 1.2 Hz, 6’-H of Quinoline), 7.59–7.53 (m, 2H, 6-H and 7’-H of Quinoline), 7.25 (t, 2H, J = 10.0 Hz, 5,7-H) ppm; 13C NMR (100 MHz, CDCl3): δC = 150.24, 147.64, 146.10, 141.40, 137.28, 136.70, 133.50, 129.60, 129.45, 129.26, 128.31, 128.21, 127.06, 124.21, 114.46 ppm; HRMS (ESI, positive): calcd for C19H13N + Na+ [M + Na]+ 278.0941, found: 278.0941. Anal. Calcd for C19H13N: C, 89.38; H, 5.13; N, 5.49. Found: C, 89.08; H, 5.25; N, 5.44.
2,2'-Biazulene (6)13: mp >300 °C (lit. >300 °C); MS (EI, 70 eV): m/z (relative intensity, %) = 254 (100). Although low solubility of compound 6 hampered the 1H NMR measurement, effective formation of compound 14 from the product should provide the criterion of the structure of compound 6.
6-(2-Pyridyl)azulene (8): mp 113.5−114.0 °C (CH2Cl2/MeOH); IR (KBr): νmax = 3088 (w), 3078 (w), 3024 (w), 1576 (s), 1552 (w), 1475 (s), 1444 (m), 1419 (m), 1400 (m), 1365 (m), 1327 (w), 1304 (m), 1304 (w), 1226 (m), 1199 (m), 1107 (m), 1053 (m), 1024 (s), 972 (s), 844 (s), 802 (s), 754 (w), 750 (w), 713 (s), 671 (m), 621 (m), 572 (m), 555 (w), 501 (w), 488 (w), 418 (m), 403 (m) cm−1; UV-Vis (CH2Cl2): λmax (log ε) = 290 (4.74), 298 (4.75), 340 sh (3.78), 352 (3.84), 370 (3.85), 600 (2.56), 650 sh (2.48), 730 sh (1.96) nm; 1H NMR (400 MHz, CDCl3): δH = 8.75 (dd, 1H, J = 4.8, 1.2 Hz, 6’-H of Py), 8.45 (d, 2H, J = 10.8 Hz, 4,8-H), 7.93 (t, 1H, J = 4.0 Hz, 2-H), 7.80–7.74 (m, 4H, 5,7-H and 3’,5’-H of Py), 7.41 (d, 2H, J = 4.0 Hz, 1,3-H), 7.28 (t, 1H, J = 4.8, 1.2 Hz, 4’-H of Py) ppm; 13C NMR (100 MHz, CDCl3): δC = 161.07, 150.08, 148.08, 138.18, 137.29, 136.23, 123.19, 123.03, 118.80, 107.86 ppm; HRMS (ESI, positive): calcd for C15H11N + Na+ [M + Na]+ 228.0784, found: 228.0784. Anal. Calcd for C15H11N∙1/5H2O: C, 86.27; H, 5.50; N, 6.71. Found: C, 86.57; H, 5.51; N, 6.49.
6-(3-Pyridyl)azulene (9): mp 119.0−120.0 °C (CH2Cl2/MeOH); IR (KBr): νmax = 3024 (w), 3005 (w), 1576 (s), 1552 (w), 1475 (s), 1444 (m), 1419 (m), 1400 (m), 1365 (m), 1327 (w), 1304 (m), 1226 (m), 1199 (m), 1107 (m), 1053 (m), 1024 (s), 972 (s), 844 (s), 802 (s), 754 (w), 750 (w), 713 (s), 671 (m), 621 (m), 572 (m), 555 (m), 501 (m), 488 (w), 418 (m), 403 (m) cm−1; UV-Vis (CH2Cl2): λmax (log ε) = 298 (4.82), 336 sh (3.72), 352 (3.81), 368 (3.71), 588 (2.56), 634 sh (2.48), 710 sh (2.00) nm; 1H NMR (400 MHz, CDCl3): δH = 8.90 (d, 1H, J = 1.6 Hz, 2’-H of Py), 8.65 (d, 1H, J = 4.8, 1.6 Hz, 6’-H of Py), 8.41 (d, 2H, J = 10.0 Hz, 4,8-H), 7.95–7.91 (m, 2H, 2-H and 5’-H of Py), 7.45 (d, 2H, J = 4.0 Hz, 1,3-H), 7.39 (dd, 1H, J = 4.8, 1.6 Hz, 4’-H of Py), 7.32 (d, 2H, J = 10.0 Hz, 5,7-H) ppm; 13C NMR (100 MHz, CDCl3): δC = 149.30, 148.99, 147.07, 140.78, 139.12, 137.72, 135.85, 135.61, 123.36, 123.01, 118.93 ppm; HRMS (ESI, positive): calcd for C15H11N + Na+ [M + Na]+ 228.0784, found: 228.0784. Anal. Calcd for C15H11N: C, 87.77; H, 5.40; N, 6.82. Found: C, 87.72; H, 5.48; N, 6.76.
6-(3-Quinolyl)azulene (10): mp 162.0−164.0 °C (CH2Cl2/MeOH); IR (KBr): νmax = 3080 (w), 3055 (w), 1618 (w), 1578 (s), 1562 (w), 1549 (w), 1491 (m), 1471 (m), 1442 (m), 1400 (s), 1352 (w), 1338 (w), 1304 (w), 1228 (w), 1126 (w), 1053 (w), 1024 (w), 978 (w), 970 (w), 956 (w), 945 (w), 908 (m), 856 (m), 837 (s), 785 (m), 746 (m), 638 (m), 574 (w), 480 (m), 463 (m), 451 (m), 420 (m) cm−1; UV-Vis (CH2Cl2): λmax (log ε) = 284 (4.65), 300 sh (4.60), 308 sh (4.60), 352 (3.91), 370 (3.95), 590 (2.48), 638 sh (2.40), 710 sh (1.91) nm; 1H NMR (400 MHz, CDCl3): δH = 9.22 (d, 1H, J = 1.6 Hz, 2’-H of Quinoline), 8.45 (d, 2H, J = 10.0 Hz, 4,8-H), 8.36 (d, 1H, J = 1.6 Hz, 4’-H of Quinoline), 8.18 (d, 1H, J = 8.0 Hz, 8’-H of Quinoline), 7.96 (t, 1H, J = 4.0 Hz, 2-H), 7.90 (d, 1H, J = 8.0 Hz, 5’-H of Quinoline), 7.76 (t, 1H, J = 8.0 Hz, 7’-H of Quinoline), 7.56 (t, 1H, J = 8.0 Hz, 6’-H of Quinoline), 7.47–7.44 (m, 4H, 1,3,5,7-H) ppm; 13C NMR (100 MHz, CDCl3): δC = 150.88, 147.86, 147.54, 139.54, 138.37, 138.19, 136.34, 135.44, 130.38, 129.67, 128.61, 128.12, 127.71, 123.73, 119.45 ppm; HRMS (ESI, positive): calcd for C19H13N + Na+ [M + Na]+ 278.0941, found: 278.0941. Anal. Calcd for C19H13N∙1/10H2O: C, 88.76; H, 5.17; N, 5.45. Found: C, 88.71; H, 5.39; N, 5.46.
General procedure for the synthesis of 2-aryl- and 6-heteroaryl-1,3-di(4-pyridyl)azulenes
Tf2O (2.4 equiv.) and pyridine (20 equiv.) in CH2Cl2 were added at room temperature to a solution of 2–5 and 8–10 in CH2Cl2. In the case of the reaction of compound 6, 6.0 equiv. Tf2O and 40 equiv. pyridine were used as reagents. The resulting mixture was stirred at the same temperature for 30 min. The solvent was removed under reduced pressure. 5 equiv. KOH was added to a solution of the crude product in MeOH at room temperature, and the resulting mixture was stirred at the same temperature for 3 h. The reaction mixture was poured into water, extracted with CH2Cl2, and dried with Na2SO4. The solvent was removed under reduced pressure and the residue was purified by column chromatography on alumina with CH2Cl2 to give the corresponding 2-aryl- and 6-heteroaryl-1,3-di(4-pyridyl)azulenes. Yield of the products is summarized in Table 3.
2-Phenyl-1,3-di(4-pyridyl)azulene (11): mp 266.0−268.0 °C (sublimation); IR (KBr): νmax = 3027 (w), 1589 (s), 1572 (m), 1536 (w), 1499 (w), 1428 (m), 1403 (m), 1295 (w), 1258 (w), 1214 (w), 1069 (w), 1029 (w), 992 (m), 916 (w), 866 (w), 854 (m), 836 (m), 822 (w), 807 (w), 791 (m), 764 (w), 743 (s), 721 (m), 709 (m), 698 (s), 676 (w), 659 (w) cm-1; UV-Vis (CH2Cl2): λmax (log ε) = 258 (4.42), 318 (4.69), 325 sh (4.68), 370 sh (3.95), 583 (2.69), 620 (2.67), 691 sh (2.37) nm; UV-Vis (10% CF3CO2H/CH2Cl2): λmax (log ε) = 260 (4.40), 300 sh (4.35), 334 (4.51), 407 sh (4.14), 546 (3.20) nm; 1H NMR (500 MHz, CDCl3): δH = 8.55 (d, 4H, J = 6.3 Hz, 2’,6’-H of Py), 8.39 (d, 2H, J = 9.8 Hz, 4,8-H), 7.67 (t, 1H, J = 9.8 Hz, 6-H), 7.27 (t, 2H, J = 9.8 Hz, 5,7-H), 7.23–7.15 (m, 7H, 3’,5’-H of Py, m-Ph, and p-Ph), 6.98 (d, 2H, J = 8.6 Hz, o-Ph) ppm; 13C NMR (125 MHz, CDCl3): δC = 149.72 (C-2’,6’ of Py), 148.33 (ipso-Ph), 144.14 (C-3a,8a), 139.12 (C-6), 137.98 (C-4’ of Py), 135.88 (C-4,8), 135.16 (C-2), 131.13 (o-Ph), 128.28 (C-1,3), 127.58 (m-Ph), 126.35 (C-3’,5’ of Py), 126.24 (p-Ph), 125.72 (C-5,7) ppm; HRMS (EI, positive): calcd for C26H18N2+ [M]+ 358.1465, found: 358.1470. Anal. Calcd for C26H18N2: C, 87.12; H, 5.06; N, 7.82. Found: C, 87.03; H, 5.18; N, 7.79.
2-(3-Pyridyl)-1,3-di(4-pyridyl)azulene (12): mp 242.0−244.0 °C (CH2Cl2/MeOH); IR (KBr): νmax = 3032 (w), 1590 (s), 1572 (w), 1539 (w), 1505 (w), 1429 (m), 1402 (m), 1390 (w), 1310 (w), 1259 (w), 1213 (w), 1196 (w), 1114 (w), 1040 (w), 1024 (m), 991 (m), 961 (w), 883 (w), 856 (m), 838 (m), 824 (m), 793 (m), 771 (w), 757 (s), 730 (w), 709 (s), 676 (w), 666 (w), 658 (w) cm−1; UV-Vis (CH2Cl2): λmax (log ε) = 252 (4.30), 315 (4.64), 323 sh (4.62), 368 sh (3.86), 583 (2.62), 623 (2.59), 694 sh (2.19) nm; UV-Vis (10% CF3CO2H/CH2Cl2): λmax (log ε) = 258 (4.24), 295 sh (4.40), 317 (4.45), 401 sh (4.04), 554 (2.90) nm; 1H NMR (500 MHz, CDCl3): δH = 8.59 (d, 4H, J = 4.6 Hz, 2’,6’-H of Py), 8.46 (d, 1H, J = 4.9 Hz, 6”-H of Py), 8.41 (d, 2H, J = 9.9 Hz, 4,8-H), 8.27 (s, 1H, 2’-H of Py), 7.73 (t, 1H, J = 9.9 Hz, 6-H), 7.33 (dd, 1H, J = 4.9, 1.8 Hz, 4’-H of Py), 7.32 (t, 2H, J = 9.9 Hz, 5,7-H), 7.17 (d, 4H, J = 4.- Hz, 3’,5’-H of Py), 7.13 (dd, 1H, J = 4.9, 1.8 Hz, 5’’-H of Py) ppm; 13C NMR (125 MHz, CDCl3): δC = 151.39 (C-2” of Py), 149.97 (C-2’,6’ of Py), 148.60 (C-6” of Py), 143.95 (C-3” of Py), 143.56 (C-3a,8a), 139.87 (C-6), 138.11 (C-4” of Py), 138.00 (C-4’ of Py), 136.62 (C-4,8), 131.28 (C-2), 126.44 (C-1,3), 126.30 (C-3’,5’ of Py), 126.00 (C-5,7), 123.08 (C-5” of Py) ppm; HRMS (EI, positive): calcd for C25H17N3+ [M]+ 359.1417, found: 359.1416. Anal. Calcd for C25H17N3: C, 83.54; H, 4.77; N, 11.69. Found: C, 83.50; H, 4.82; N, 11.68.
1,3-Di(4-pyridyl)-2-(3-quinolyl)azulene (13): mp > 300 °C (CH2Cl2/MeOH); IR (KBr): νmax = 3028 (w), 2965 (w), 1592 (s), 1573 (w), 1540 (w), 1504 (w), 1434 (m), 1410 (m), 1376 (w), 1273 (w), 1217 (m), 1132 (w), 1067 (w), 1008 (w), 990 (w), 977 (w), 949 (w), 916 (m), 872 (w), 855 (w), 826 (m), 797 (s), 763 (s), 756 (s), 732 (w), 726 (w), 711 (s), 678 (w), 668 (w), 658 (w) cm−1; UV-Vis (CH2Cl2): λmax (log ε) = 269 (4.60), 330 (4.92), 373 sh (4.17), 396 sh (3.77), 585 (2.85), 623 (2.83), 694 sh (2.44) nm; UV-Vis (10% CF3CO2H/CH2Cl2): λmax (log ε) = 257 (4.54), 285 sh (4.60), 333 (4.77), 410 sh (4.17), 556 (3.09) nm; 1H NMR (500 MHz, CDCl3): δH = 8.57 (d, 4H, J = 5.3 Hz, 2’,6’-H of Py), 8.57 (s, 1H, 2”-H of Quinoline), 8.44 (d, 2H, J = 9.8 Hz, 4,8-H), 8.03 (d, 1H, J = 8.4 Hz, 8”-H of Quinoline), 7.76 (s, 1H, 4”-H of Quinoline), 7.70 (t, 1H, J = 9.8 Hz, 6-H), 7.70 (dd, 1H, J = 8.6, 8.6 Hz, 7”-H of Quinoline), 7.56 (d, 1H, J = 8.6 Hz, 5”-H of Quinoline), 7.49 (dd, 1H, J = 8.6, 8.6 Hz, 6”-H of Quinoline), 7.33 (t, 2H, J = 9.8 Hz, 5,7-H), 7.20 (d, 4H, J = 4.6 Hz, 3’,5’-H of Py) ppm; 13C NMR (125 MHz, CDCl3): δC = 152.02 (C-2” of Quinoline), 150.06 (C-2’,6’ of Py), 146.90 (C-4a” or C-8a” of Quinoline), 143.81 (C-4” of Quinoline), 143.64 (C-4’ of Py), 139.90 (C-6), 138.11 (C-4a” or C-8a” of Quinoline), 137.81 (C-3a,8a), 136.60 (C-4,8), 130.12 (C-7” of Quinoline), 129.38 (C-8” of Quinoline), 128.51 (C-2), 128.05 (C-5” of Quinoline), 127.41 (C-3” of Quinoline), 127.13 (C-6” of Quinoline), 126.61 (C-1,3), 126.31 (C-3’,5’ of Py), 126.07 (C-5,7) ppm; HRMS (EI, positive): calcd for C29H19N3+ [M]+ 409.1574, found: 409.1585. Anal. Calcd for C29H19N3: C, 85.06; H, 4.68; N, 10.26. Found: C, 84.98; H, 4.78; N, 10.24.
1,1’,3,3’-Tetra(4-pyridyl)-2,2’-biazulene (14): mp >300 °C (CH2Cl2/MeOH); IR (KBr): νmax = 3061 (w), 1594 (s), 1573 (m), 1541(w), 1504 (w), 1430 (m), 1370 (m), 1312 (w), 1303 (w), 1221 (w), 1125 (w), 1067 (w), 993 (w), 912 (w), 878 (w), 859 (w), 825 (m), 782 (m), 746 (m), 728 (s), 686 (s), 670 (w), 659 (w) cm−1; UV-Vis (CH2Cl2): λmax (log ε) = 311 sh (4.59), 342 (4.88), 414 (3.99), 595 (2.91), 635 (2.89), 706 sh (1.71) nm; UV-Vis (10% CF3CO2H/CH2Cl2): λmax (log ε) = 257 (4.45), 350 (4.72), 362 sh (4.71), 403 sh (4.37), 573 (3.22) nm; 1H NMR (500 MHz, CDCl3): δH = 8.34 (d, 4H, J = 9.7 Hz, 4,8-H), 8.26 (dd, 8H, J = 1.6, 4.5 Hz, 2’,6’-H of Py), 7.70 (t, 2H, J = 9.7 Hz, 6-H), 7.28 (t, 4H, J = 9.7 Hz, 5,7-H), 6.58 (d, 8H, J = 1.6, 4.5 Hz, 3’,5’-H of Py) ppm; 13C NMR (125 MHz, CDCl3): δC = 149.44 (C-2’,6’ of Py), 143.27 (C-3a,8a), 142.19 (C-2), 140.07 (C-6), 137.77 (C-4’ of Py), 136.14 (C-4,8), 127.24 (C-1,3), 126.17 (C-5,7), 125.23 (C-3’,5’ of Py) ppm; HRMS (FAB, positive): calcd for C40H26N4 + H+ [M + H]+ 563.2231, found: 563.2257. Anal. Calcd for C40H26N4: C, 85.38; H, 4.66; N, 9.96. Found: C, 85.35; H, 4.70; N, 9.95.
6-(3-Pyridyl)-1,3-di(4-pyridyl)azulene (15): mp 218.0−220.0 °C (CH2Cl2/MeOH); IR (KBr): νmax = 3015 (m), 2969 (m), 1591 (m), 1507(w), 1463 (w), 1433 (m), 1370 (s), 1275 (w), 1267 (w), 1228 (s), 1216 (s), 1095 (w), 990 (w), 814 (m), 767 (s), 749 (s), 699 (w), 670 (w), 658 (m) cm−1; UV-Vis (CH2Cl2): λmax (log ε) = 242 sh (4.32), 310 sh (4.54), 337 (4.69), 371 sh (4.13), 398 sh (3.79), 567 sh (2.49), 616 (2.58), 670 sh (2.48), 758 sh (1.95) nm; UV-Vis (10% CF3CO2H/CH2Cl2): λmax (log ε) = 256 (4.25), 287 sh (4.03), 327 sh (4.49), 348 (4.54), 400 (4.33), 419 sh (4.27), 562 (2.83) nm; 1H NMR (500 MHz, CDCl3): δH = 8.72 (d, 1H, J = 4.6 Hz, 6”-H of Py), 8.68−8.66 (m, 6H, 4,8-H and 2’,6’-H of Py), 8.13 (s, 1H, 2-H), 7.91 (d, 2H, J = 10.8 Hz, 5,7-H), 7.80−7.79 (m, 2H, 2”,4”-H of Py), 7.51 (d, 4H, J = 5.8 Hz, 3’,5’-H of Py), 7.15 (dd, 1H, J = 4.6, 4.6 Hz, 5”-H of Py) ppm; 13C NMR (125 MHz, CDCl3): δC = 159.38 (C-6), 150.59 (C-3” of Py), 150.22 (C-2’,6’ of Py), 150.07 (C-6” of Py), 144.28 (C-3a,8a), 137.41 (C-2), 137.23 (C-2” or C-4” of Py), 135.68 (C-4,8), 128.19 (C-1,3), 125.60 (C-5,7), 124.31 (C-3’,5’ of Py), 123.39 (C-5” of Py), 122.76 (C-2” or C-4” of Py) ppm. The signal for C-4’ of pyridine could not be observed probably due to the overlapping with the other signal. HRMS (EI, positive): calcd for C25H17N3+ [M]+ 359.1417, found: 359.1416. Anal. Calcd for C25H17N3∙1/5H2O: C, 82.71; H, 4.83; N, 11.57. Found: C, 82.65; H, 4.85; N, 11.60.
1,3-Di(4-pyridyl)-6-(3-quinolyl)azulene (16): mp >300 °C (CH2Cl2/MeOH); IR (KBr): νmax = 3030 (w), 1594(s), 1577(m), 1550 (w), 1504 (w), 1491 (w), 1431 (m), 1370 (m), 1334 (w), 1312 (w), 1251 (w), 1220 (w), 1131 (w), 1033 (w), 992 (m), 949 (w), 916 (w), 854 (m), 815 (s), 783 (m), 742 (m), 720 (w), 700 (m), 685 (w), 679 (w), 658 (m) cm−1; UV-Vis (CH2Cl2): λmax (log ε) = 239 sh (4.47), 311 (4.56), 343 (4.75), 377 sh (4.33), 442 sh (2.92), 560 sh (2.55), 606 (2.65), 658 sh (2.55), 740 sh (2.04) nm; UV-Vis (10% CF3CO2H/CH2Cl2): λmax (log ε) = 254 (4.37), 286 (4.20), 327 sh (4.53), 360 (4.67), 418 sh (4.38), 551 (3.03) nm; 1H NMR (500 MHz, CDCl3): δH = 9.24 (d, 1H, J = 2.3 Hz, 2”-H of Quinoline), 8.75 (d, 4H, J = 6.3 Hz, 2’,6’-H of Py), 8.72 (d, 2H, J = 11.0 Hz, 4,8-H), 8.41 (d, 1H, J = 2.3 Hz, 4”-H of Quinoline), 8.21 (s, 1H, 2-H), 8.20 (d, 1H, J = 8.6 Hz, 8”-H of Quinoline), 7.93 (d, 1H, J = 8.6 Hz, 5”-H of Quinoline), 7.79 (dd, 1H, J = 8.6, 8.6 Hz, 7”-H of Quinoline), 7.64 (dd, 1H, J = 8.6, 8.6 Hz, 6”-H of Quinoline), 7.61 (d, 2H, J = 11.0 Hz, 5,7-H), 7.59 (d, 4H, J = 6.3 Hz, 3’,5’-H of Py) ppm; 13C NMR (125 MHz, CDCl3): δC = 150.29 (C-2’,6’ of Py), 150.19 (C-6), 149.92 (C-2” of Quinoline), 148.01 (C-4a” or C-8a” of Quinoline), 144.11 (C-3a,8a), 137.31 (C-2), 136.88 (C-8” of Quinoline), 135.76 (C-4,8), 135.08 (C-4” of Quinoline), 130.40 (C-7” of Quinoline), 129.52 (C-4' of Py), 128.89 (C-6” of Quinoline), 128.27 (C-5” of Quinoline), 127.68 (C-4a” or C-8a” of Quinoline), 127.56 (C-1,3), 125.88 (C-5,7), 124.24 (C-3’,5’ of Py) ppm. The signal for C-3” of quinoline could not be observed probably due to the overlapping with the other signal. HRMS (EI, positive): calcd for C29H19N3+ [M]+ 409.1574, found: 409.1585. Anal. Calcd for C29H19N3∙1/3H2O: C, 83.83; H, 4.77; N, 10.11. Found: C, 83.85; H, 4.73; N, 10.12.
ACKNOWLEDGEMENTS
This work was partially supported by a Grant-in-Aid for Research (Grant No. 25810019 to T.S.) from the Ministry of Education, Culture, Sports, Science, and Technology, Japan.
References
1. (a) K.-P. Zeller, in Azulene in Methoden der Organischen Chemie (Houben-Weyl), 4th ed. (ed. by H. Kropf), Thieme, Stuttgart, 1985, Vol. V, Part 2c, pp. 127–418; (b) S. Ito and N. Morita, Eur. J. Org. Chem., 2009, 4567; CrossRef (c) S. Ito, T. Shoji, and N. Morita, Synlett, 2011, 2279. CrossRef
2. (a) S. Ito, T. Okujima, and N. Morita, Tetrahedron Lett., 2002, 43, 1261; CrossRef (b) S. Ito, T. Okujima, and N. Morita, J. Chem. Soc., Perkin Trans. 1, 2002, 1896; (c) S. Ito, T. Terazono, T. Kubo, T. Okujima, N. Morita, T. Murafuji, Y. Sugihara, K. Fujimori, J. Kawakami, and A. Tajiri, Tetrahedron, 2004, 60, 5357. CrossRef
3. S. Ito, T. Kubo, N. Morita, Y. Matsui, T. Watanabe, A. Ohta, K. Fujimori, T. Murafuji, Y. Sugihara, and A. Tajiri, Tetrahedron Lett., 2004, 45, 2891. CrossRef
4. (a) S. Ito, H. Inabe, T. Okujima, N. Morita, M. Watanabe, N. Harada, and K. Imafuku, J. Org. Chem., 2001, 66, 7090; CrossRef (b) S. Ito, A. Nomura, N. Morita, C. Kabuto, H. Kobayashi, S. Maejima, K. Fujimori, and M. Yasunami, J. Org. Chem., 2002, 67, 7295; CrossRef (c) S. Ito, H. Inabe, N. Morita, K. Ohta, T. Kitamura, and K. Imafuku, J. Am. Chem. Soc., 2003, 125, 1669; CrossRef (d) T. Shoji, S. Ito, K. Toyota, M. Yasunami, and N. Morita, Chem. Eur. J., 2008, 14, 8398; CrossRef (e) T. Shoji, E. Shimomura, M. Maruyama, S. Ito, T. Okujima, and N. Morita, Eur. J. Org. Chem., 2013, 957; CrossRef (f) T. Shoji, E. Shimomura, M. Maruyama, A. Maruyama, S. Ito, T. Okujima, K. Toyota, and N. Morita, Eur. J. Org. Chem., 2013, 7785. CrossRef
5. C. Lambert, G. Nöll, M. Zabel, F. Hampel, E. Schmälzlin, C. Bräuchle, and K. Meerholz, Chem. Eur. J., 2003, 9, 4232. CrossRef
6. A part of this study was reported previously in preliminary form: T. Shoji, S. Kikuchi, S. Ito, and N. Morita, Heterocycles, 2005, 66, 91. CrossRef
7. (a) S. Dumouchel, F. Mongin, F. Trécourt, and G. Quéguiner, Tetrahedron Lett., 2003, 44, 2033; CrossRef (b) S. Dumouchel, F. Mongin, F. Trécourt, and G. Quéguiner, Tetrahedron Lett., 2003, 44, 3877; CrossRef (c) S. Dumouchel, F. Mongin, F. Trécourt, and G. Quéguiner, Tetrahedron, 2003, 59, 8629. CrossRef
8. T. Shoji, S. Ito, K. Toyota, T. Iwamoto, M. Yasunami, and N. Morita, Eur. J. Org. Chem., 2009, 4307. CrossRef
9. (a) A. Inoue, K. Kitagawa, H. Shinokubo, and K. Oshima, J. Org. Chem., 2001, 66, 4333; CrossRef (b) T. Iida, T. Wada, K. Tomimoto, and T. Mase, Tetrahedron Lett., 2001, 42, 4841. CrossRef
10. To obtain the product in high yield, 5 mol% of palladium catalyst was required at least (see reference 6).
11. M. Yasunami, A. Chen, P.-W. Yang, and K. Takase, Chem. Lett., 1980, 9, 579. CrossRef
12. S. Wakabayashi, Y. Kato, K. Mochizuki, R. Suzuki, M. Matsumoto, Y. Sugihara, and M. Shimizu, J. Org. Chem., 2007, 72, 744. CrossRef
13. (a) T. Morita and K. Takase, Bull. Chem. Soc. Jpn., 1982, 55, 1144; CrossRef (b) T. Shibasaki, T. Ooishi, N. Yamanouchi, T. Murafuji, K. Kurotobi, and Y. Sugihara, J. Org. Chem., 2008, 73, 7971. CrossRef
14. A. R. Katritzky, S. Zhang, T. Kurz, M. Wang, and P. J. Steel, Org. Lett., 2001, 3, 2807. CrossRef
15. E. J. Corey and Y. Tian, Org. Lett., 2005, 7, 5535. CrossRef
16. (a) T. Shoji, R. Yokoyama, S. Ito, M. Watanabe, K. Toyota, M. Yasunami, and N. Morita, Tetrahedron Lett., 2007, 48, 1099; CrossRef (b) T. Shoji, S. Ito, M. Watanabe, K. Toyota, M. Yasunami, and N. Morita, Tetrahedron Lett., 2007, 48, 3009; CrossRef (c) T. Shoji, S. Ito, K. Toyota, M. Yasunami, and N. Morita, Tetrahedron Lett., 2007, 48, 4999; CrossRef (d) J. Higashi, T. Shoji, S. Ito, K. Toyota, M. Yasunami, and N. Morita, Eur. J. Org. Chem., 2008, 5823; CrossRef (e) T. Shoji, S. Ito, T. Okujima, J. Higashi, R. Yokoyama, K. Toyota, M. Yasunami, and N. Morita, Eur. J. Org. Chem., 2009, 1554; CrossRef (f) T. Shoji, S. Ito, K. Toyota, and N. Morita, Eur. J. Org. Chem., 2010, 1059; CrossRef (g) T. Shoji, S. Ito, J. Higashi, and N. Morita, Eur. J. Org. Chem., 2011, 5311; CrossRef (h) T. Shoji, Y. Inoue, S. Ito, T. Okujima, and N. Morita, Heterocycles, 2012, 85, 35; CrossRef (i) T. Shoji, A. Maruyama, M. Maruyama, S. Ito, T. Okujima, J. Higashi, K. Toyota, and N. Morita, Bull. Chem. Soc. Jpn., 2014, 87, 141. CrossRef
17. 1H NMR measurement was performed in acetone-d6, owing to the insolubility of cationic species of 12.
18. The B3LYP/6-31G** time-dependence density functional calculations were performed with Spartan’10, Wavefunction, Irvine, CA.
19. T. Shoji, A. Yamamoto, Y. Inoue, E. Shimomura, S. Ito, J. Higashi, and N. Morita, Chem. Lett., 2012, 41, 1644. CrossRef
20. T. Shoji, A. Yamamoto, E. Shimomura, M. Maruyama, S. Ito, T. Okujima, K. Toyota, and N. Morita, Chem. Lett., 2013, 42, 638. CrossRef