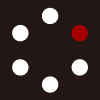
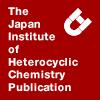
HETEROCYCLES
An International Journal for Reviews and Communications in Heterocyclic ChemistryWeb Edition ISSN: 1881-0942
Published online by The Japan Institute of Heterocyclic Chemistry
e-Journal
Full Text HTML
Received, 4th November, 2015, Accepted, 16th December, 2015, Published online, 18th January, 2016.
■ An Efficient Conversion of Lysine to 2-Aminocaprolactam
Hiroshi Matsumoto, Kouji Kaiso, and Akio Kamimura*
Department of Applied Molecular Bioscience, Graduate School of Medicine, Yamaguchi University, , Japan
Abstract
Treatment of lysine in wet MeOH at high-temperatures provided a smooth and efficient conversion to 2-aminocaprolactam that is recognized as a potential intermediate for the preparation of bioactive compounds. The obtained 2-aminocaprolactam underwent very rapid racemization under these conditions. A kinetic study of the reaction was performed.2-Aminocaprolactam is recognized as an important compound because it is included in a component of bioactive products. For example, this structure is contained in families of natural products such as bengamides1 and caprolactins,2 whose syntheses have been reported.3 Parker and co-workers found that aminocaprolactam derivatives are effective inhibitors of γ-secretase and potential drugs for preventing Alzheimer’s disease.4 This structure is also included in factor Xa inhibitors such as BMS-3445775 and the capuramycin-related antibiotics A500359 and A503083.6 Because of these interesting aspects, the development of a method for the efficient preparation of 2-aminocaprolactam has been a topic of research interest.
Previous syntheses of 2-aminocaprolactam have included 2-amination of caprolactam,7 acidic ring closure of lysine,8 and Beckman rearrangement of α-aminocyclohexanone oxime. 9 Goto and co-workers recently reported a single-step preparation of 2-aminocaprolactam in which lysine smoothly undergoes dehydration in subcritical water at 573K for 20 min to afford the lactam in 51% yield.10 Although this single-step protocol is attractive, the decomposition of 2-aminocaprolactam is unavoidable at long reaction time and is the main reason for the moderate yield. In addition, this protocol employs high-temperature water, which may cause undesirable side reactions because of its strong reactivity. The authors reported some kinetic data for the reaction, but they did not provide a detailed reaction mechanism.
Recently, we reported that supercritical i-PrOH is a good reaction solvent for the selective conversion of polyamide to the corresponding monomer (Scheme 1).11 Use of supercritical MeOH, however, resulted in further conversion of caprolactam to give hydroxycapronic ester and alkenyl ester.12 The report prompted us to examine the dehydration reaction of lysine in high-temperature alcohol to improve the conversion because supercritical alcohol provides a mild reaction conditions. Here we report the efficient conversion of lysine to racemic 2-aminocaprolactam under high-temperature wet MeOH conditions. Kinetic and mechanistic studies of the racemization of 2-aminocaprolactam are also described.
The conversion of lysine 1 to 2-aminocaprolactam 2 was examined under various conditions. The reactions were conducted in sealed stainless steel tubes with Swagelok caps at both ends, which were heated in an oven adjusted to a certain temperature. The amounts of compounds 1 and 2 were quantified by HPLC analyses with a curve-fitting method using L-valine as an internal standard. The results are summarized in Table 1.
Treatment of lysine 1 with sub-critical water at 250 °C for 30 min resulted in the formation of compound 2 in 10% yield (entry 1). Because the reaction is regarded to proceed via dehydration, anhydrous conditions were expected to improve the yield of 2. MeOH was thus used as the reaction solvent and 2 was obtained in 55% yield (entry 2). To our surprise, the use of a mixed MeOH-water solvent (80:20 v/v) enhanced the yield of 2 to 72% yield (entry 3). This result is attributed to the fact that high-temperature MeOH is less reactive than high-temperature water and prevents side reactions from compound 2. The use of other alcohols under sealed tube conditions also gave 2 in slightly lower yields (entries 4 to 6). In contrast, toluene and toluene-water were less efficient solvent systems for this conversion (entries 7 and 8). These results may be due to the less solubility of the amino acid. Compound 2 was not formed in refluxing BuOH (entry 9), and only a moderate yield of the desired lactam was obtained after refluxing 1 in DMSO for 30 min (entry 10). Thus, we concluded that MeOH-water was the most effective solvent for the reaction.
We next examined the reaction temperature. The reaction progressed smoothly at 220 °C, and compound 2 was obtained in 72% yield (entry 11). The reaction at 180 °C achieved an efficient conversion although the reaction required 6 h (entry 12). The reaction carried out at 130 °C failed to form 2 (entry 13). Thus, 250 °C was concluded as the optimum reaction temperature for efficient progress of the reaction.
The kinetics of the reaction and the chirality of 2 were investigated. The time–course of the reaction was examined at 250 °C, 180 °C, and 130 °C. The results are depicted in Figure 1.
The reaction progressed smoothly when compound 1 was heated at 250 °C. Short induction periods of approximately 10 and 20 min were observed at 250 and 180 °C, respectively, and were attributed to the initial heating period, during which the reaction vessel reached the reaction temperature. Following the induction period, the yield of 2 increased rapidly and exceeded 70% after 30 min (solid red line). The reaction at 180 °C progressed slowly and reached a maximum yield after 120 min (solid green line). A temperature of 130 °C was insufficient to drive the reaction, and lysine 1 was nearly quantitatively recovered after 120 min (black solid and dotted lines). Thus, a temperature of 180 °C or above is necessary for the reaction to proceed.
The time–course data were used to estimate the kinetic parameters of the reaction. The values for log[1] were plotted as a function of the reaction time, and good correlations were observed (Figure 2). The slopes of the lines for each reaction temperature provided the Arrhenius plot, from which the activation energy of the reaction was calculated to be approximately 15 kcal/mol. Thus, it is thought that the cyclization readily occurs under the reaction conditions used in the present study, as long as the temperature is sufficient.
The optical purity of compound 2 was examined. The enantiomeric excess values for compounds 1 and 2 were analyzed via HPLC using Cadenza CD-C18 and SUMICHIRAL OA-5000. Although it was expected that the optical purity of compound 2 would be maintained during the conversion, the isolated lactam was racemic. Such fast racemization has not been reported previously. To verify the degree of racemization, the enantiomeric excesses of compounds 1 and 2 were determined under various reaction conditions. The results are summarized in Figure 3.
Unfortunately, both L-lysine 1 and 2-aminocaprolactam 2 were readily racemized as the reaction progressed. For example, 1 lost its optical activity within 40 min when heated at 250 °C (red dotted line). The rate of racemization was lower, however, when the reaction was conducted at 180 °C (green dotted line). In contrast, no racemization of 1 was observed in the reaction performed at 130 °C, although the formation of 2 was very slow (Figure 1, black solid line). In all cases, the ee values for 2-aminocaprolactam 2 changed as the reaction progressed. For example, the initial ee values of 70% decreased to 0% in 30 min of the reaction at 250 °C. The racemization rate of 2 decreased as the reaction was carried out at lower temperature, dropping to 20% after 120 min at 180 °C (dotted green line). Note that the racemization of 2 was always faster than that of 1. These results clearly suggest that racemization mainly occurs after the formation of compound 2, and that the racemization of remaining 1 occurs via the equilibrium of compounds 1 and 2 (vide infra). It is known that 2-aminocaprolactam 2 readily undergoes enzyme-catalyzed racemization.13
Racemization of compound 2 was very fast in MeOH-water (4:1) and complete in 20 min at 250 °C (Scheme 2). The racemization rate decreased significantly at 130 °C for 60 min, and enantiomeric excess of 2 was maintained. Use of anhydrous toluene reduced the rate of racemization at 250 °C: the ee of 2 was 75% ee after 20 min. Dimethylaminolactam 3 also underwent complete racemization when heated in MeOH-water or toluene-water solvent at 250 °C for 20 min. In contrast, the racemization was almost completely prevented when compound 3 was heated in absolute toluene. Under these conditions, an external proton was not available in the reaction mixture. These results suggest that racemization was facilitated by the presence of protons that can promote enol formation.
We assume that this racemization of aminocaprolactam 2 occurs via enol formation in the presence of a proton that is likely chelated to the carbonyl oxygen and the amino group at the C2 position (Scheme 3). This proton can also be supplied by a protic solvent. Hydrogen bonding of the proton at the C2 amino group induces tautomerization to give the enol form of 2. Tautomerism then produces racemic lactam 2. The equilibrium of compounds 1 and 2 is likely the main route for the racemization of lysine 1 because the racemization rate of 1 was faster than that of 2, although direct racemization of lysine 1 was possible.
In conclusion, the present methodology enables clean and fast preparation of 2-aminocaprolactam in greater than 70% yield in a single step from readily available lysine. This method is practical because the present high-temperature conditions are recognized as rather convenient for industrial processes. Although the product is racemized during cyclization because of the presence of acidic protons that promote tautomerization to the enol form of compound 2, the optical resolution of racemic 2 has been previously demonstrated.14 We therefore believe that this simple and easy to manipulate reaction will contribute to the preparation of bioactive compounds that contain the caprolactam subunit.
EXPERIMENTAL
Typical procedure for the conversion of 1 to 2-aminocaprolactam 2: L-Lysine (101.5 mg, 0.70 mmol) was dissolved in MeOH-water (4:1 v/v, 2.5 mL) and the solution was placed in a 10 mL reaction vessel (7.35 mm id × 23 cm stainless tube capped on both ends with Swagelok nuts) under an argon atmosphere. After sealing, the reaction vessel was placed in a hot oven (250 °C) for an appropriate reaction time. The reaction vessel was cooled in a dry-ice/MeOH bath, and L-valine was added as an internal standard. The reaction mixture was analyzed and the amounts of each enantiomer of 1 and 2 were quantified via HPLC using Cadenza CD-C18 or SUMICHIRAL OA-5000.
Isolation of 2-aminocaprolactam 2: L-Lysine (101.5 mg, 0.70 mmol) was dissolved in MeOH-water (4:1 v/v, 2.5 mL), and the solution was placed in a 10 mL reaction vessel (7.35 mm id × 23 cm stainless tube capped on both ends with Swagelok nuts) under an argon atmosphere. After sealing, the reaction vessel was placed in a hot oven (250 °C). After 30 min, the reaction vessel was cooled in a dry-ice/MeOH bath, and the reaction mixture was concentrated in vacuo. The residue was subjected to flash chromatography (silica gel/MeOH) to give 2-aminocaprolactam 2 in 76% yield (0.53 mmol). The 1H and 13C NMR spectra of the product were compared with those of a commercially available sample and found to be identical. Pale yellow solid; mp 73-74 °C (lit. 70-73 °C)15; 1H NMR (500 MHz, CDCl3) δ 6.24 (s, 1H), 3.54 (dd, J = 10.9, 2.0 Hz, 1H), 3.22 (ddd, J = 8.8, 6.1, 2.7 Hz, 2H), 2.07 – 1.96 (m, 1H), 1.90 – 1.77 (m, 4H), 1.69 (dtt, J = 13.8, 12.7, 3.5 Hz, 1H), 1.61 – 1.51 (m, 1H), 1.48 – 1.32 (m, 1H); 13C NMR (126 MHz, CDCl3) δ 179.4, 54.0, 42.0, 33.8, 29.1, 28.5; HRMS (ESI-TOF): calcd for C6H12N2NaO 151.0847 [M + Na+], found 151.0842.
Preparation of dimethylaminocaprolactam 3: Triethylamine (2.77 mL, 20 mmol) was added to a suspension of L-2-aminocaprolactam hydrochloride (3.293 g, 20.0 mmol) in MeCN (100 mL) at room temperature. Formalin (37%, 16.23 g, 0.2 mol) and acetic acid (0.11 mL, 2 mmol) were then added to the solution, followed by NaBH(OAc)3 (12.716 g, 60 mmol) portionwise. The resulting reaction mixture was stirred at room temperature for 8 h and concentrated in vacuo. 10% NaOH aq (200 mL) was added to the residue, and the resulting aqueous solution was extracted with CH2Cl2 (4 × 200 mL). The organic phase was combined, and HCl solution of dioxane (4 M, 5.5 mL) was added. Concentration of the solution under reduced pressure afforded a solid product that was recrystallized in EtOAc and isopropanol. The obtained crystals were dissolved in water (50 mL) and basified with 10% NaOH (10 mL), then the solution was extracted with CH2Cl2 (4 × 100 mL). The organic phase was combined, and dried over MgSO4. Filtration and concentration gave compound 3 in 28% (0.8878 g, 5.68 mmol). Colorless oil; [α]D +15.1 (c 0.98, CHCl3); 1H NMR (400 MHz, CDCl3) δ 6.99 (s, 1H), 3.48 (dt, J = 14.6, 6.9 Hz, 1H), 2.98–2.86 (m, 1H), 2.86–2.79 (m, 1H), 2.20 (s, 6H), 1.83 (qd, J = 8.6, 8.1, 4.0 Hz, 1H), 1.71–1.60 (m, 2H), 1.58–1.40 (m, 3H); 13C NMR (101 MHz, CDCl3) δ 178.1, 69.7, 42.5 (2C), 41.3, 29.6, 26.9, 25.5; Anal. Calcd. for C8H16N2O: C, 61.50; H, 10.32; N, 17.93. Found: C, 61.17; H, 10.04; N, 17.91; HRMS (ESI-TOF): calcd for C8H17N2O 157.1341 [M + H+], found 157.1341.
ACKNOWLEDGEMENTS
This work was partially supported by JSPS KAKENHI Grant Number 24241023.
References
1. (a) Z. Thale, F. R. Kinder, K. W. Bair, J. Bontempo, A. M. Czuchta, R. W. Versace, P. E. Phillips, M. L. Sanders, S. Wattanasin, and P. Crews, J. Org. Chem., 2001, 66, 1733; CrossRef (b) A. Groweiss, J. J. Newcomer, B. R. O’Keefe, A. Blackman, and M. R. Boyd, J. Nat. Prod., 1999, 62, 1691; (c) R. Fernandez, M. Dherbomez, Y. Letourneux, M. Nabil, J. F. Verbist, and J. F. Biard, J. Nat. Prod., 1999, 62, 678; CrossRef (d) M. V. D’Auria, C. Giannini, L. Minale, A. Zampella, C. Debitus, and M. Frostin, J. Nat. Prod., 1997, 60, 814; (e) M. Adamczeski, E. Quinoa, and P. Crews, J. Org. Chem., 1990, 55, 240; CrossRef (f) M. Adamczeski, E. Quinoa, and P. Crews, J. Am. Chem. Soc., 1989, 111, 647; CrossRef (g) E. Quinoa, M. Adamczeski, P. Crews, and G. J. Bakus, J. Org. Chem., 1986, 51, 4494. CrossRef
2. B. S. Davidson and R. W. Schumacher, Tetrahedron, 1993, 49, 6569. CrossRef
3. (a) R. K. Boeckman, Jr., T. J. Clark, and B. C. Shook, Org. Lett., 2002, 4, 2109; CrossRef (b) F. R. Kinder, Jr., S. Wattanasin, R. W. Versace, K. W. Bair, J. Bontempo, M. A. Green, Y. J. Lu, H. R. Marepalli, P. E. Phillips, D. Roche, L. D. Tran, R.-M. Wang, L. Waykole, D. D. Xu, and S. Zabludoff, J. Org. Chem., 2001, 66, 2118; CrossRef (c) J. A. Marshall and G. P. Luke, Synlett, 1992, 1007; CrossRef (d) C. A Broka and J. Ehrler, Tetrahedron Lett., 1991, 32, 5907. CrossRef
4. (a) M. L. Neitzel, D. L. Aubele, J. L. Marugg, J. J. Jagodzinski, A. W. Konradi, M. A. Pleiss, B. Szoke, W. Zmolek, E. Goldbach, K. P. Quinn, J.-M. Sauer, E. F. Brigham, W. Wallace, M. P. Bova, S. Hemphill, and G. Basi, Bioorg. Med. Chem. Lett., 2011, 21, 3715; CrossRef (b) M. F. Parker, J. J. Bronson, D. M. Barten, J. A. Corsa, W. Du, K. M. Felsenstein, V. L. Guss, D. Izzarelli, A. Loo, K. E. McElhone, L. R. Marcin, R. Padmanabha, R. Pak, C. T. Polson, J. H. Toyn, S. Varma, J. Wang, V. Wong, M. Zheng, and S. B. Roberts, Bioorg. Med. Chem. Lett., 2007, 17, 5790. CrossRef
5. (a) S. P. O’Connor, K. Atwal, C. Li, E. C.-K. Liu, S. M. Seiler, M. Shi, Y. Shi, P. D. Stein, and Y. Wang, Bioorg. Med. Chem. Lett., 2008, 18, 4696; (b) Y. Shi, C. Li, S. P. O’Connor, J. Zhang, M. Shi, S. N. Bisaha, Y. Wang, D. Sitkoff, A. T. Pudzianowski, C. Huang, H. E. Klei, K. Kish, J. Yanchunas, Jr., E. C.-K. Liu, K. S. Hartl, S. M. Seiler, T. E. Steinbacher, W. A. Schumacher, K. S. Atwal, and P. D. Stein, Bioorg. Med. Chem. Lett., 2009, 19, 6882; (c) Y. Shi, J. Zhang, M. Shi, S. P. O’Connor, S. N. Bisaha, C. Li, D. Sitkoff, A. T. Pudzianowski, S. Chong, H. E. Klei, K. Kish, J. Yanchunas, Jr., E. C.-K. Liu, K. S. Hartl, S. M. Seiler, T. E. Steinbacher, W. A. Schumacher, K. S. Atwal, and P. D. Stein, Bioorg. Med. Chem. Lett., 2009, 19, 4034.
6. Z. Yang, M. Funabashi, K. Nonaka, M. Hosobuchi, T. Shibata, P. Pahari, and S. G. Van Lanen, J. Biol. Chem., 2010, 285, 12899, and references cited therein. CrossRef
7. A. Font, Tetrahedron Lett., 1980, 21, 2443; M. Brenner and H. R. Rickenbacher, Helv. Chim. Acta, 1958, 41, 181.
8. A. Mondrzyk and H. Ritter, Polym. Int., 2015, 64, 661; J. Fischer and H. Ritter, Beilstein J. Org. Chem., 2013, 9, 2803.
9. W. Kessler and M. Brenner, Helv. Chim. Acta, 1969, 52, 901. CrossRef
10. M. Goto, M. Umeda, A. Kodama, T. Hirose, S. Nagaoka, S. Matsuda, S. Masuhara, and J. Hiraki, J. Chem. Eng. Jpn., 2004, 37, 353. CrossRef
11. A. Kamimura, Y. Oishi, K. Kaiso, T. Sugimoto, and K. Kashiwagi, ChemSusChem, 2008, 1, 82. CrossRef
12. (a) A. Kamimura, K. Kaiso, S. Suzuki, Y. Oishi, Y. Ohara, T. Sugimoto, K. Kashiwagi, and M. Yoshimoto, Green Chem., 2011, 13, 2055; CrossRef (b) A. Kamimura, K. Ikeda, S. Suzuki, K. Kato, Y. Akinari, T. Sugimoto, K. Kashiwagi, K. Kaiso, H. Matsumoto, and M. Yoshimoto, ChemSusChem, 2014, 7, 2473; CrossRef (c) H. Matsumoto, Y. Akinari, K. Kaiso, and A. Kamimura, J. Mater. Cycles Waste Manag., 2015, 17, in press: DOI 10.1007/s10163-015-0425-4. CrossRef
13. S. A. Ahmed, N. Esaki, H. Tanaka, and K. Soda, Biochemistry, 1986, 25, 385. CrossRef
14. K. Sakai, R. Sakurai, A. Yazawa, and N. Hirayama, Tetrahedron: Asymmetry, 2003, 14, 3713. CrossRef
15. A. Bladé-Font, Tetrahedron Lett., 1980, 21, 2443. CrossRef